DOI:
10.1039/C9SC06369B
(Edge Article)
Chem. Sci., 2020,
11, 1672-1676
Direct cyclopropanation of activated N-heteroarenes via site- and stereoselective dearomative reactions†
Received
17th December 2019
, Accepted 30th December 2019
First published on 10th January 2020
Abstract
A divergent cyclopropanation reaction has been accomplished via the dearomative addition of sulfur ylides to activated N-heteroarenes. A series of biologically significant molecular skeletons was obtained by the direct cyclopropanation of quinolinium zwitterions. Furthermore, a straightforward synthetic route to optically enriched cyclopropane-fused heterocycles was developed using sulfur ylides as chiral nucleophiles in the 1,4-dearomative reaction.
Introduction
Cyclopropane is a versatile synthetic intermediate and a common structural motif in natural products and pharmaceuticals.1 In particular, stereoselectively fused cyclopropanes in close vicinity to nitrogen-containing six-membered cyclic compounds are found in many biologically active molecules (Scheme 1A).2 Due to their potential biological activities, the development of efficient methods to access fused six-membered N-heterocyclic frameworks has gained considerable research attention in the fields of medicinal and synthetic chemistry. Although various synthetic approaches have been devised to directly construct a six-membered framework,3 the dearomative functionalization of activated N-heteroarenes provides a simple strategy to access such compounds.4 However, the dearomative reaction is frequently challenging because of the poor site selectivity between the two potentially reactive sites (C2 and C4) being susceptible to nucleophilic attack. Hence, various approaches to improve the site selectivity have been designed (Scheme 1B).5 Although additional strategic approaches are still necessary to effectively control the selectivity-determining variables, the hard or soft nature of the nucleophile has been reported to often affect the ratio of regioisomers.4 In addition, the cascade strategy, known as the Wenkert procedure, is considered to be an outstanding example of dearomative transformation, a key strategy for synthesizing various natural products (Scheme 1C).6 However, to facilitate C4-selective nucleophilic attack, an electron-withdrawing group is required at the C3 position of the activated heteroarene. With our continued efforts in the exploration of cycloaddition reactions,7 a straightforward dearomative transformation of N-aromatic zwitterions via the Wenkert procedure was envisioned (Scheme 1C). In addition, the employment of a nucleophile bearing a neighboring leaving group was proposed, thereby circumventing the stepwise addition of the acid and generating an imine intermediate that could be easily attacked by the tethered nucleophilic moiety. This strategy was further extended for stereoselective reactions. Thus, we herein describe an unprecedented formal cycloaddition of N-aromatic zwitterions by the 1,4-dearomative addition of sulfur ylides. In addition, the asymmetric cyclopropanation of heteroarenes is reported for the first time via the asymmetric 1,4-addition of N-aromatic zwitterions of chiral sulfur ylides.
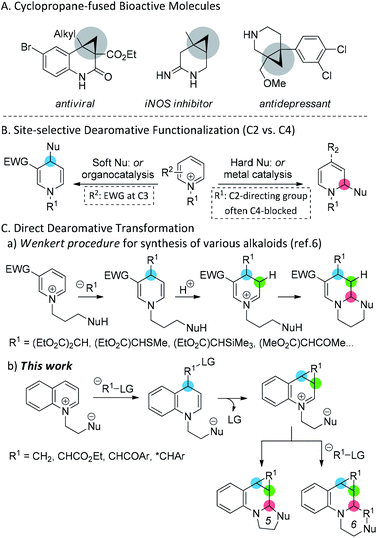 |
| Scheme 1 Dearomative transformations of activated heteroarenes. | |
Results and discussion
To achieve the cycloaddition of activated N-heteroarene via the modified Wenkert procedure, we first considered the use of sulfur ylides as a dearomative reagent. This was based on the fact that (1) sulfur ylides are well known zwitterionic C1 synthons that have been used in a number of transformations;8 in addition, the regioselective cycloaddition of sulfur ylides, known as the Corey–Chaykovsky reaction, is well established9 and (2) the electronic and stereogenic variations of sulfur ylides are known to be simple.10 Prompted by the pioneering reactions of sulfur ylides, we began our investigation by evaluating a number of sulfur ylides for the site-selective dearomative reaction with N-aromatic zwitterions (Table 1).
Table 1 Optimization studies for the divergent cyclopropanationa,b
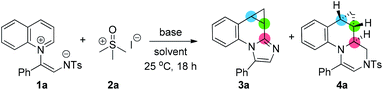
|
Entry |
Base |
Solvent |
3a (%) |
4a (%) |
Conditions: 1a (0.1 mmol), 2a (2 equiv.), base (2 equiv.), solvent (1 mL), 25 °C, 18 h.
NMR yield using CH2Br2 as an internal standard.
2.5 mL of solvent was used.
Isolated yield.
2.5 equiv. of 2a and NaH were added to 2.0 mL of solvent at 40 °C.
|
1 |
NaH |
CH3CN |
13 |
— |
2 |
NaH |
1,2-DCE |
33 |
— |
3 |
NaH |
DMSO |
19 |
34 |
4 |
NaH |
DMF |
— |
82c (81d) |
5 |
NaOMe |
DMF |
51 |
— |
6e |
NaOMe |
DMF |
86 (83d) |
— |
7 |
KOtBu |
DMF |
26 |
29 |
As expected, the reaction of the sulfoxonium ylide, which was generated in situ from quinolinium zwitterion (1a) and trimethylsulfoxonium iodide (2a), afforded low yields of the desired product (3a) in polar solvents (entries 1 and 2). When the reaction was conducted in DMSO, a typical solvent for the Corey–Chaykovsky reaction, an unexpected cycloadduct 4a was synthesized in 34% yield along with the desired product (3a) (entry 3). Surprisingly, a single isomer of 4a was produced diastereoselectively in a high yield when DMF was used as the solvent (entry 4). In addition, sodium methoxide was found to be the optimal base after intensive screening of various bases (entry 5). Interestingly, 4a was not formed under the optimized conditions (entry 6), even though an excess of sulfoxonium ylide was employed.
To decipher the sequence of the cascade reaction, we conducted a control experiment using isolated compound 5 and excess of sulfoxonium ylide (Scheme 2A). However, the expected compound 4a was not observed, and the starting material (5) was fully recovered. It was therefore concluded that the reaction proceeded via a [2 + 1] cycloaddition of zwitterion (1a) and sulfur ylide (A) followed by a formal [5 + 1] cycloaddition of Int II and sulfur ylide A.
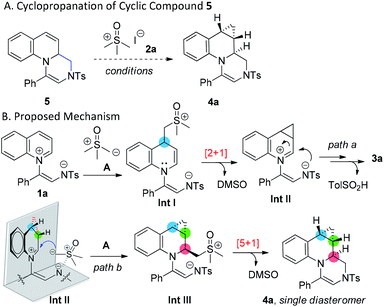 |
| Scheme 2 Proposed mechanism of the cascade cycloaddition. | |
The use of a strong base, NaH, immediately generated free ylide A, which could participate in the intermolecular nucleophilic attack on Int II (path b), along with the evolution of H2 gas. In contrast, the rate of the 2nd intermolecular nucleophilic addition (path b) of a low concentration of ylide A generated from the relatively weak base, NaOMe, may be more sluggish than the intramolecular cyclization route (path a). Since no chiral additives were required to control the diastereoselectivity, we proposed that the second cycloaddition (path b) in the cascade reaction occurred in the direction opposite to the cyclopropane ring of Int II (Scheme 2B).12 Thus, to understand the inherent selective dearomative reaction of sulfur ylides, we examined the generality of the formal cycloaddition of these sulfur ylides with a number of quinolinium zwitterions (Scheme 3). Remarkably, almost all zwitterions (1) selectively underwent 1,4-dearomative transformations to afford the desired cyclopropane-fused cyclic scaffolds (3). In addition, the reaction yields were not significantly influenced by the electronic variation on the quinolinium backbone. However, as expected, steric variation at the reaction site had a drastic effect on the product yield (3c). Furthermore, the reactivity of the aryl substituted sulfonium ylide toward quinolinium zwitterions (1a) was significantly poorer than that of ylide A, resulting in the formation of the desired product (3i) in 41% yield. Moreover, isolable ester- and ketosulfonium ylides (2) exhibited high reactivities with excellent 1,4-dearomative selectivity, resulting in the formation of cyclopropane-fused N-heterocycles 3j–3m, without the detection of any byproducts.
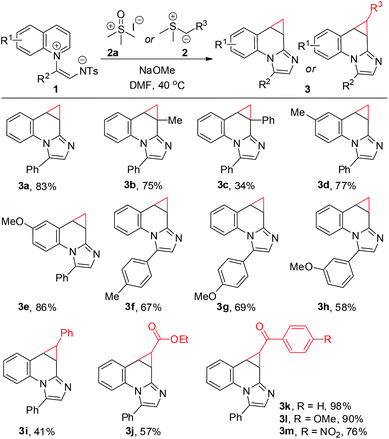 |
| Scheme 3 Formal [2 + 1] cycloaddition of N-aromatic zwitterions via 1,4-dearomative addition of sulfur ylides. | |
As illustrated in Scheme 4, the cascade reaction via dearomative [2 + 1] and [5 + 1] cycloadditions could also be effectively applied to a wide range of quinolinium zwitterions (1) to afford the corresponding cycloadducts 4. It is noteworthy that the 3-substituent on the quinolinium zwitterion, which could hinder both the first and second cycloaddition reactions, could be altered to obtain the desired product 4b in 59% yield. Conducting further experiments using zwitterions (1) bearing other functional groups, we concluded that the substrate scope of the developed method was quite broad. Also, electronic variations in the sulfonyl groups of the zwitterions had little effect on the product yield (4p and 4q).
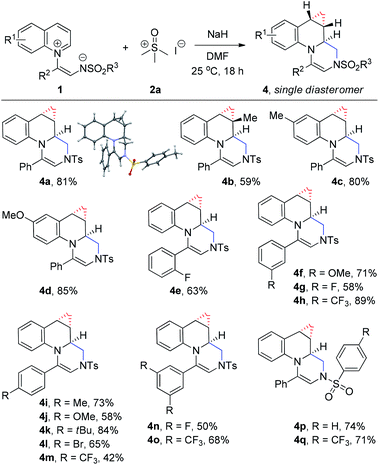 |
| Scheme 4 Substrate scope for the stereoselective synthesis of cyclopropane-fused N-heterocycles. | |
Based on the above selective cyclopropanation reactions, we attempted to develop a straightforward route for generating optically enriched cyclopropane-fused heterocyclic compounds (Scheme 5). We were pleased to find that the first attempt of the cyclopropanation of the quinolinium zwitterion (1a) and a chiral sulfonium salt (6) under the standard conditions using NaOMe as the base and DMF as the solvent gave the desired product (7a) in 30% isolated yield and 93% ee.11 To increase the yield of the desired products, NaH and acetonitrile were selected as the base and solvent, respectively.11 It should be noted that in this case, the double cycloadduct 4 was not obtained, presumably due to the steric bulkiness of the chiral ylide. The observed high enantioselectivity arose from the high facial selectivity of the ylide, which was generated from 6.10a Unlike the enantioselectivity, the diastereomeric ratio of the obtained products was low. However, the two diastereomers were separable and the enantiomeric excesses of both isomers were high. As shown for compounds 7a–7c, the enantioselectivity was not hampered by the presence of substituents on the quinolinium backbone of the zwitterions (1). As expected, variation in the enamide moiety of the zwitterion did not affect the reactivity or selectivity for synthesizing enantioselective cyclopropane-fused products (7d–7f) due to the distance from the reaction site. The developed method was then extended to other sulfonium ylides bearing substituents with different electronic and steric properties. To our delight, good reactivities and high enantioselectivities were observed in all cases regardless of the substituents employed, furnishing the desired products 7g–7i.
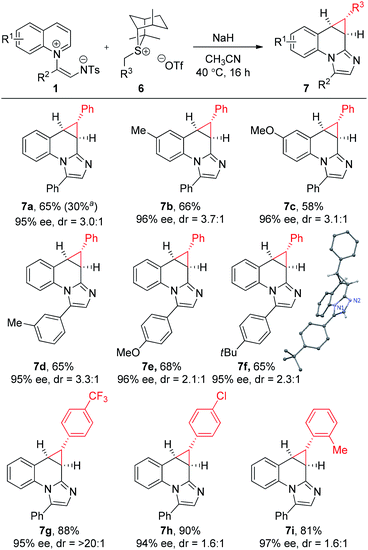 |
| Scheme 5 Enantioselective cyclopropanation of N-aromatic compounds with sulfonium ylides. a The reaction mixture of the quinolinium zwitterion 1 and sulfonium salt 6 was stirred in DMF with NaOMe as the base. | |
To demonstrate the synthetic utility of the obtained products, additional experiments were performed (Scheme 6). A scale-up experiment, carried out using 4 mmol of quinolinium zwitterion 1a, gave the desired product 4a in 79% yield without any loss in efficiency. Moreover, detosylation of the obtained 4h proceeded readily under mild reaction conditions to give 8, which could be efficiently converted into 9via a typical amination reaction.
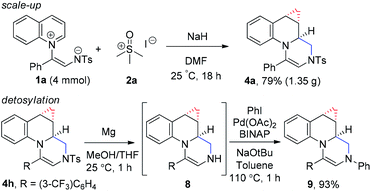 |
| Scheme 6 Applications of cyclopropane-fused N-heterocycles. | |
Conclusions
We revealed the first successful use of sulfur ylides in the dearomative reaction, ultimately enabling a series of formal cycloaddition reactions of N-aromatic zwitterions. Using this approach, biologically relevant heterocyclic compounds with wide structural diversity could be rapidly obtained in a single operation. In addition, the successful development of the asymmetric cyclopropanation of chiral sulfur ylides with quinolinium zwitterions demonstrated the potential applications of N-aromatic zwitterions in organic syntheses.
Conflicts of interest
There are no conflicts to declare.
Acknowledgements
This research was supported by the National Research Foundation of Korea (NRF) Grants (NRF-2019R1A2C1087134, NRF-2019R1A4A2001440) funded by the Korean government, in addition to the GRRC Program of Gyeonggi Province (GRRCKyungHee2017-A01).
Notes and references
- For Selective reviews of cyclopropanes, see:
(a) C. Ebner and E. M. Carreira, Chem. Rev., 2017, 117, 11651 CrossRef CAS PubMed;
(b) D. Y.-K. Chen, R. H. Pouwer and J.-A. Richard, Chem. Soc. Rev., 2012, 41, 4631 RSC;
(c) W. Wu, Z. Lin and H. Jiang, Org. Biomol. Chem., 2018, 16, 7315 RSC;
(d) H. Lebel, J.-F. Marcoux, C. Molinaro and A. B. Charette, Chem. Rev., 2003, 103, 977 CrossRef CAS PubMed.
-
(a) S. Gomi, D. Ikeda, H. Nakamura, H. Naganawa, F. Yamashita, K. Hotta, S. Kondo, Y. Okami, H. Umezawa and Y. Iitaka, J. Antibiot., 1984, 37, 1491 CrossRef CAS PubMed;
(b) I. Takahashi, K.-I. Takahashi, M. Ichimura, M. Morimoto, K. Asano, I. Kawamoto, F. Tomit and H. Nakano, J. Antibiot., 1988, 41, 1915 CrossRef CAS PubMed;
(c) M. Zhang, F. Jovic, T. Vickers, B. Dyck, J. Tamiya, J. Grey, J. A. Tran, B. A. Fleck, R. Pick, A. C. Foster and C. Chen, Bioorg. Med. Chem. Lett., 2008, 18, 3682 CrossRef CAS PubMed;
(d) F. P. Bymaster, K. Golembiowska, M. Kowalska, Y. K. Choi and F. I. Tarazi, Synapse, 2012, 66, 522 CrossRef CAS PubMed;
(e) K. T. Warnock, A. R. S. T. Yang, H. S. Yi, H. L. June Jr, T. Kelly, A. S. Basile, P. Skolnick and H. L. June Sr, Pharmacol., Biochem. Behav., 2012, 103, 111 CrossRef CAS PubMed.
-
(a) M. G. P. Buffat, Tetrahedron, 2004, 60, 1701 CrossRef CAS;
(b) F.-X. Felpin and J. Lebreton, Eur. J. Org. Chem., 2003, 3693 CrossRef CAS;
(c) D. M. Stout and A. I. Meyers, Chem. Rev., 1982, 82, 223 CrossRef CAS.
-
(a) J. A. Bull, J. J. Mousseau, G. Pelletier and A. B. Charette, Chem. Rev., 2012, 112, 2642 CrossRef CAS PubMed;
(b) G. Bertuzzi, L. Bernardi and M. Fochi, Catalyst, 2018, 8, 632 CrossRef;
(c) I. S. Poddubnyi, Chem. Heterocycl. Compd., 1995, 31, 682 CrossRef;
(d) A. Kakehi, Heterocycles, 2012, 85, 1529 CrossRef CAS.
- For recent examples of dearomatization of activated N-heteroarenes, see:
(a) A. Grozavu, H. B. Hepburn, P. J. Smith, H. K. Potukuchi, P. J. Lindsay-Scott and T. J. Donohoe, Nat. Chem., 2019, 11, 242 CrossRef CAS PubMed;
(b) G. Bertuzzi, A. Sinisi, L. Caruana, A. Mazzanti, M. Fochi and L. Bernardi, ACS Catal., 2016, 6, 6473 CrossRef CAS;
(c) M. Nagase, Y. Kuninobu and M. Kanai, J. Am. Chem. Soc., 2016, 138, 6103 CrossRef CAS PubMed;
(d) K. Kubota, Y. Watanabe, K. Hayama and H. Ito, J. Am. Chem. Soc., 2016, 138, 4338 CrossRef CAS PubMed;
(e) O. G. Mancheño, S. Asmus, M. Zurro and T. Fischer, Angew. Chem., Int. Ed., 2015, 54, 8823 CrossRef PubMed;
(f) T. Nishida, H. Ida, Y. Kuninobu and M. Kanai, Nat. Commun., 2014, 5, 3387 CrossRef PubMed;
(g) M. Mohiti, C. Rampalakos, K. Feeney, D. Leonori and V. K. Aggarwal, Chem. Sci., 2014, 5, 602 RSC. For examples of dearomative ring formations of activated N-heteroarenes, see:
(h) S. G. Parameswarappa and F. C. Pigge, Org. Lett., 2010, 12, 3434 CrossRef CAS PubMed;
(i) G. Arnott, H. Brice, J. Clayden and E. Blaney, Org. Lett., 2008, 10, 3089 CrossRef CAS PubMed.
- For selective reviews, see:
(a) J. Bosch and M.-L. Bennasar, Synlett, 1995, 6, 587 CrossRef;
(b) E. Wenkert, Heterocycles, 1984, 21, 325 CrossRef CAS.
-
(a) S.-y. Baek, J. Y. Lee, D. Ko, M.-H. Baik and E. J. Yoo, ACS Catal., 2018, 8, 6353 CrossRef CAS;
(b) N. De, C. E. Song, D. H. Ryu and E. J. Yoo, Chem. Commun., 2018, 54, 6911 RSC;
(c) D. J. Lee, D. Ko and E. J. Yoo, Angew. Chem., Int. Ed., 2015, 54, 13715 CrossRef CAS PubMed;
(d) D. J. Lee, H. S. Han, J. Shin and E. J. Yoo, J. Am. Chem. Soc., 2014, 136, 11606 CrossRef CAS PubMed.
-
(a) V. K. Aggarwal and C. L. Winn, Acc. Chem. Res., 2004, 37, 611 CrossRef CAS PubMed;
(b) L.-Q. Lu, T.-R. Li, Q. Wang and W.-J. Xiao, Chem. Soc. Rev., 2017, 46, 4135 RSC;
(c) J. D. Neuhaus, R. Oost, J. Merad and N. Maulide, Top. Curr. Chem., 2018, 376, 15 CrossRef PubMed.
-
(a) E. J. Corey and M. Chaykovsky, J. Am. Chem. Soc., 1965, 87, 1353 CrossRef CAS;
(b) P. Mosset and R. Grée, Synth. Commun., 1985, 15, 749 CrossRef CAS;
(c) M. G. Edwards, R. J. Paxton, D. S. Pugh, A. C. Whitwood and R. J. K. Taylor, Synthesis, 2008, 20, 3279 Search PubMed;
(d) Y. Xiang, X. Fna, P.-J. Cai and Z.-X. Yu, Eur. J. Org. Chem., 2019, 582 CrossRef CAS.
- For selective examples, see:
(a) O. Illa, M. Namutebi, C. Saha, M. Ostovar, C. C. Chen, M. F. Haddow, S. Nocquet-Thibault, M. Lusi, E. M. McGarrigle and V. K. Aggarwal, J. Am. Chem. Soc., 2013, 135, 11951 CrossRef CAS PubMed;
(b) S. Ye, Z.-Z. Huang, C.-A. Xia, Y. Tang and L.-X. Dai, J. Am. Chem. Soc., 2002, 124, 2432 CrossRef CAS PubMed;
(c) V. K. Aggarwal, E. Alonso, G. Hynd, K. M. Lydon, M. J. Palmer, M. Porcelloni and J. R. Studley, Angew. Chem., Int. Ed., 2001, 40, 1430 CrossRef CAS;
(d) S. Miniére, V. Reboul, P. Metzner, M. Fochi and B. F. Bonini, Tetrahedron: Asymmetry, 2004, 15, 3275 CrossRef.
- See the ESI.†.
-
(a) D. L. Comins, R. R. Goehring, S. P. Joseph and S. O'Connor, J. Org. Chem., 1990, 55, 2574 CrossRef CAS;
(b) D. L. Comins and M. O. Killpack, J. Am. Chem. Soc., 1992, 114, 10972 CrossRef CAS;
(c) J. Streith, A. Boiron, T. Sifferlen, C. Strehler and T. Tschamber, Tetrahedron Lett., 1994, 35, 3927 CrossRef CAS.
Footnotes |
† Electronic supplementary information (ESI) available: Synthetic procedures, full characterisation and spectroscopic data. CCDC 1944296 and 1944297 for 4a and 7f respectively. For ESI and crystallographic data in CIF or other electronic format see DOI: 10.1039/c9sc06369b |
‡ These authors contributed equally. |
|
This journal is © The Royal Society of Chemistry 2020 |