DOI:
10.1039/C9SC03798E
(Edge Article)
Chem. Sci., 2020,
11, 281-289
Physicochemical-property guided design of a highly sensitive probe to image nitrosative stress in the pathology of stroke†
Received
31st July 2019
, Accepted 8th November 2019
First published on 11th November 2019
Abstract
In vivo real-time imaging of nitrosative stress in the pathology of stroke has long been a formidable challenge due to both the presence of the blood–brain barrier (BBB) and the elusive nature of reactive nitrogen species, while this task is also informative to gain a molecular level understanding of neurovascular injury caused by nitrosative stress during the stroke episode. Herein, using a physicochemical property-guided probe design strategy in combination with the reaction-based probe design rationale, we have developed an ultrasensitive probe for imaging nitrosative stress evolved in the pathology of stroke. This probe demonstrates an almost zero background fluorescence signal but a maximum 1000-fold fluorescence enhancement in response to peroxynitrite, the nitrosative stress marker. Due to its good physicochemical properties, the probe readily penetrates the BBB after intravenous administration, and quickly accumulates in mice brain to sense local vascular injuries. After accomplishing its imaging mission, the probe is easily metabolized and therefore won't cause safety concerns. These desirable features make the probe competent for the straightforward visualization of nitrosative stress progression in stroke pathology.
Stroke is an acute episode of focal dysfunction of the brain, spinal cord or retina lasting longer than 24 h.1 It is one of the most dangerous diseases with a high rate of mortality and disability. Despite recent remarkable advances in its treatment and prevention, stroke remains the second single most frequent cause of death for people older than 60 years and the first cause of permanent disability.2 With the aging of the world's population, the global burden of stroke keeps increasing. In consequence, there is a pressing need to continue investigating the mechanism, prevention and treatment of this devastating disease. Several key players contribute to neuronal injury and death following stroke, including oxidative and nitrosative stress, excitotoxicity, and inflammation,3 among which, the damaging role of nitrosative stress is attracting increasing research interest. Nitrosative stress is represented by the overproduction of peroxynitrite (ONOO−) during both cerebral ischemia and subsequent reperfusion.4,5 It facilitates the demise of the penumbra by protein nitration, lipid peroxidation, DNA oxidation, mitochondrial damage, activation or inhibition of various signaling pathways, and blood–brain barrier (BBB) breakdown.6 Due to its ultrahigh toxicity, it is emerging as an attractive molecular target for therapeutic intervention.6
One of the main challenges in the development of ONOO−-targeted stroke treatment is the ideal timing and dosage of medication, as this determines a lot of clinical outcomes.7 A technology capable of spatiotemporally mapping nitrosative stress during stroke onset and progression would improve people's understanding of when and how ONOO− overproduction contributes to stroke pathology, yielding straightforward information for treatment design. However, this has long been a forbidden task due to the extremely short half-life of ONOO− and its non-genetic origin.8,9 Recent advances in reaction-based small-molecule fluorescent probes provide the possibility to track elusive small biomolecules in their local environments.10,11 Actually, for targeting ONOO−, there have been several robust probes reported that were confirmed for their specificity and capability to image nitrosative stress in the context of inflammation,12–16 kidney injury,17 hepatotoxicity,18–21 and heart disease.22,23 However, the context of the neurovascular unit is more formidable due to the presence of the blood–brain barrier (BBB). Actually, due to the rigid planar structure of most fluorophores which is essential for their emissive properties, few probes have been reported to readily penetrate the BBB and realize the non-invasive imaging of stroke events. Herein, we reported our physicochemical-property guided design of an ultrasensitive fluorogenic probe, which demonstrates desirable brain distribution after intravenous injection to fulfill the direct observation of nitrosative stress during stroke onset and progression.
Results and discussion
Physicochemical-property guided probe design
To image local nitrosative stress during stroke onset, it is necessary for the probe to readily penetrate the BBB and accumulate there to a desirable concentration. Similar to the principles in drug development, we assume that the physicochemical properties of a probe should play a key role in determining its in vivo absorption and distribution properties. To rationally design a probe with promising brain absorption and distribution properties, we screened a set of classical fluorescent dyes with sufficient brightness for their partition coefficient between water and oil (log
P), which is the fundamental principle for BBB permeability (Fig. 1a).24 Log
P measurements were conducted with a shake flask n-octanol/water partition experiment. The results showed that among the eight fluorophores commonly used for bio-imaging (Fig. S1†), the benzo-BODIPY scaffold (F4) showed a log
P of 2.60 which falls into the suggested threshold of 2–5 proposed by Hitchcock et al. for possible BBB permeability (Fig. 1a).24 Moreover, F4 also demonstrated a moderate water solubility of 250 μM as determined by the UV-Visible spectrophotometric method (Fig. S2†). Besides, F4 was quite bright with a quantum yield (Φ) of 0.788 in aqueous solution. All these physicochemical and photophysical properties make the F4 fluorophore a desirable starting point for probe development.
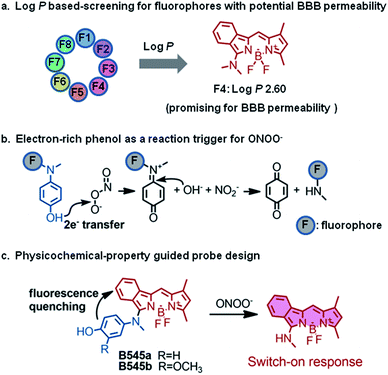 |
| Fig. 1 Physicochemical-property guided fluorescent probe design for non-invasive imaging of ONOO− during stroke in live mice. (a) Partition coefficients (log P) of classical fluorophores were determined and benzo-BODIPY (F4) was predicted to have a potentially good BBB permeability. (b) Electron-rich phenols may be oxidized to quinine by ONOO− and this reaction has been used as a trigger for ONOO− probe development. (c) Probes B545a and B545b were designed based on the F4 fluorophore which showed desirable log P for BBB permeability. B545a and B545b were hypothesized to undergo a biocompatible reaction with ONOO− to be transformed into highly fluorescent products. | |
Given the reactivity of ONOO− to oxidize electron-rich phenols into quinines which has been utilized with a high degree of success for ONOO− probe design (Fig. 1b),25–30 the p-hydroxyl aniline moiety was selected as a reaction trigger for ONOO− sensing, and probes B545a and B545b were designed (Fig. 1c). We envisioned that probes B545a and B545b should have little fluorescence due to the free rotation between the fluorophore and the p-hydroxyl aniline moiety, while the p-hydroxyl aniline moiety should be readily oxidized by ONOO− under biological conditions, yielding a fluorophore structurally similar to F4 with strong fluorescence. And this change in probe fluorescence intensity may be recorded as a signal output for ONOO− sensing.
Probes B545a and B545b were facilely synthesized by the nucleophilic substitution of α-chloro benzo-BODIPY with the corresponding anilines referring to literature procedures.31 Their structures were characterized by both NMR spectroscopy and HRMS analysis.
Validating the reactivity of probes B545a and B545b towards ONOO−
Initially, the performance of the two probes in response to ONOO− was evaluated in PBS buffer (10 mM, pH 7.4). As expected, the probes alone were barely fluorescent (5 μM), presumably due to the energy consumption caused by the free rotation of the phenol ring around the BODIPY ring (Fig. S3a†). However, the addition of ONOO− (10 μM) triggered almost a simultaneous fluorescence intensity increase of the two probes, with probe B545b giving a more dramatic increase (741-fold) than B545a (5-fold) (Fig. S3b†). We presume that the better sensitivity of B545b is due to the additional methoxyl group ortho to the hydroxyl group which is electron-donating and in consequence makes the electron transferring reaction towards ONOO− more efficient. B545b was then selected as a candidate probe for ONOO− detection and its responsive features were studied in detail under biologically relevant conditions (PBS, 10 mM, pH 7.4).
First, changes in the absorptive spectra of B545b in response to various doses of ONOO− were examined. As shown in Fig. 2a, B545b showed its maximum absorption at 508 nm (ε 23
350) attributed to the π → π* absorption of the BODIPY core. However, the intensity of this band decreased gradually with increasing dose of ONOO−. And a slight blue shift of the maximum absorbance wavelength (498 nm) was observed, suggesting that the benzo-BODIPY scaffold remained largely intact under this condition while the newly formed substitution demonstrated a lower auxochromic effect compared to the p-hydroxyl aniline moiety. This observation agrees with the proposed dearylation reaction of B545b induced by ONOO−.
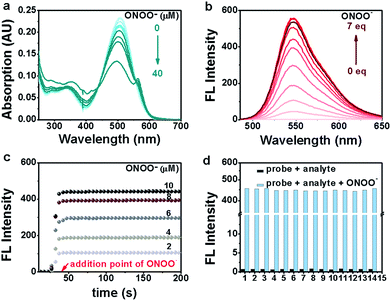 |
| Fig. 2 UV-Vis and fluorescence response of probe B545b to ONOO−. (a) Absorption spectra of B545b (10.0 μM) before and after being treated with various amounts of ONOO−. (b) Fluorescence spectra of probe B545b (5.0 μM) before and after treatment with ONOO− at indicated final concentrations. (c) Time-lapse emission of B545b (5.0 μM) at 545 nm in response to ONOO−. (d) Fluorescence intensity of B545b (5.0 μM) at 545 nm after treatment with various biologically related species. (1) Probe blank, (2) NO, (3) NO2−, (4) NO3− (5) H2O2, (6) THBP, (7) ˙OH, (8) 1O2 (9) O2−, (10) ClO−, (11) GSH, (12) Cys, (13) Zn2+, and (14) Cu2+. All analytes were kept at a final concentration of 100 μM except ONOO− which was kept at 10 μM. All measurements were carried out at ambient temperature with excitation at 475 nm and emission at 545 nm. | |
Then, the fluorescence titration experiment was performed, and an increase of B545b emission intensity was observed after ONOO− treatment. The increase was ONOO− dose-dependent (Fig. 2b). Plotting the B545b emission intensity at 545 nm versus ONOO− concentration gave an exponential dependence (Fig. S4†), suggesting a positive correlation between the ONOO− concentration and B545b fluorescence intensity. Notably, even a trace amount of ONOO− as low as 50 nM could still trigger an 8-fold enhancement of B545b emission intensity (Fig. S5†). An ONOO− concentration as low as 0.5 nM can induce a statistically significant increase of B545b fluorescence (Fig. S6†). All these results suggest the high sensitivity of B545b, supported by both its weak background signal and the high quantum yield of the F4 fluorophore.
Next, the kinetic properties of the detection reaction were studied. For this purpose, B545b fluorescence was recorded in a time-lapsed way before and after ONOO− treatment. An immediate increase of B545b fluorescence was observed as ONOO− was added, and the increase plateaued in 10 seconds (Fig. 2c), suggesting the ultrafast detection kinetics of B545b for ONOO−.
The selectivity of B545b for ONOO− was tested by recording its fluorescence response towards various biologically related species especially reactive oxidative species. It turned out that only ONOO− could switch on the fluorescence of B545b (Fig. 2d). It is noteworthy that B545b could still respond to ONOO− with a similar degree of fluorescence enhancement even in the presence of other biologically related species, suggesting its potential applicability for detecting ONOO− in a complex environment.
As the H+ concentration is modestly increased in ischemic tissues in early stages of ischemia as a result of lactic acidosis which leads to a decrease in pH to 6.4–6.7,32 it is therefore necessary to check the effect of surrounding pH on the detection sensitivity of B545b. As shown in Fig. S7,†B545b in various pH surroundings responded to a set dose of ONOO− with a similar degree of fluorescence increase, suggesting that B545b should be able to image ONOO− with a similar degree of sensitivity in the acidified bio-microenvironments.
Finally, after confirming the sensitive and selective fluorescence switch-on response of B545b to ONOO−, we monitored the detection reaction with a liquid chromatograph equipped with a mass detector (LC-MS) to make further confirmation on the detection mechanism. Treating B545b with ONOO− caused an ONOO−-dose dependent decrease of the B545b signal in the chromatogram while the emergence of a peak whose molecular weight agrees well with the proposed detection product in Fig. 1c (Fig. S8–S10†).
B545b safety and BBB permeability evaluation
Having confirmed the good performance of probe B545b as a fluorogenic probe for sensing ONOO− in aqueous solution, we then tested its cytotoxicity and BBB permeability as a further evaluation of its bio-imaging applicability. With a CCK8 assay, B545b was found to cause a negligible effect on the viability of EA.hy926 cells (Fig. S11†), implying its safety for bio-imaging.
The log
P of B545b was measured to be 2.7 by the shake flask n-octanol/water partition experiment, which, as expected, falls into the scope of 2–5 proposed by Hitchcock et al. for possible BBB permeability.24 To make further confirmation, the BBB permeability of B545b was determined with the classical pharmacokinetic techniques (intravenous administration and tissue sampling).33 Mice were administered with B545b (5 mg kg−1) via intravenous injection, and then sacrificed at different time intervals to measure B545b concentrations in the brain and plasma. As shown in Fig. S12,† due to its desirable physicochemical properties, B545b accumulates easily in the brain tissue, and a brain-to-plasma concentration ratio of 5 was observed in mice 30 min after probe administration. Moreover, B545b is easily metabolized. Its plasma and brain concentration dropped significantly in 2 h after administration. This good brain uptake, but non-retention pharmacokinetic properties, of B545b is especially desirable, as this should decrease its risk on brain function.
B545b-aided observation of ONOO− formation in endothelial cells
To test the sensitivity of B545b to image ONOO− in live cells, we first optimized its working concentration for live cell staining. EA.hy926 cells were first treated with 3-morpholinosydnonimine (SIN-1, 0.06 mM), an ONOO− donor,34 to up-regulate intracellular ONOO− levels. After being stained with B545b of various concentrations for 30 min, cells were observed under a confocal microscope. The results showed that the intracellular B545b fluorescence increased in a probe dose-dependent way, and significant brightness was observed at a concentration of 0.5 μM (Fig. S13†). Furthermore, B545b at 0.5 μM also worked well to image endogenous ONOO− in cells induced by oxygen-glucose deprivation stimulation (OGD, 4 hours).35 This suggests that a B545b concentration of 0.5 μM should be sufficient for imaging experiments (Fig. S14†).
Then, the capability of B545b to image various degrees of nitrosative stress in EA.hy926 cells was studied. Cells were first stimulated with various doses of SIN-1 for 60 min to induce various degrees of nitrosative stress, and then were stained with B545b for 30 min. The intracellular B545b fluorescence was observed to increase in a SIN-1 dose-dependent way (Fig. 3a and b), indicating the positive correlation between B545b fluorescence and the degree of nitrosative stress. It is noteworthy that B545b was able to track the dynamic progression of intracellular nitrosative stress induced by SIN-1 stimulation. As shown in Fig. 3c and 3d, when EA.hy926 cells were first loaded with B545b (30 min incubation) and then stimulated with SIN-1, the intracellular B545b fluorescence gradually intensified as SIN-1 stimulation proceeded. These results taken together suggest the sensitivity of B545b to image intracellular nitrosative stress.
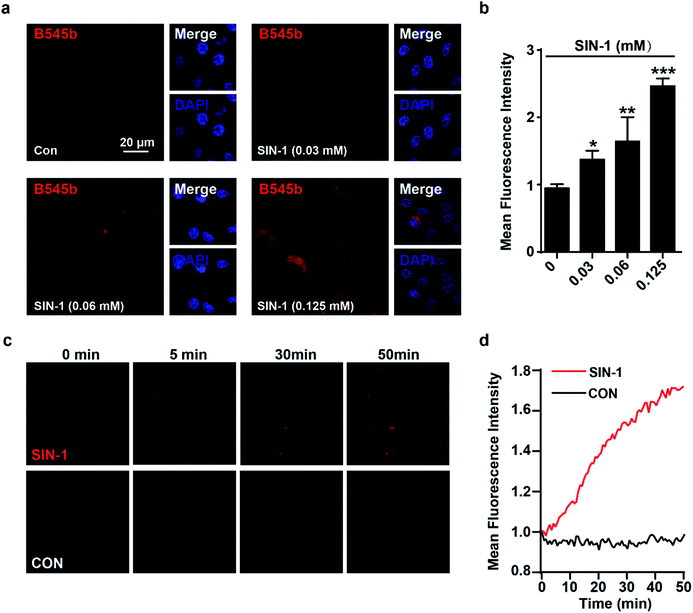 |
| Fig. 3 Imaging SIN-1 induced ONOO− in EA.hy926 endothelial cells. (a) Cells were incubated with various doses of SIN-1 for 1 h, and then stained with B545b (0.5 μM) for 30 min. (b) Quantified B545b fluorescence in (a) (mean ± S.E.M, n = 4). (c) Time-lapse series of EA.hy926 cells first loaded with B545b and then stimulated with SIN-1 (1 mM). (d) Quantified B545b fluorescence in (c). Data are presented as a densitometric ratio change compared with control. DAPI (4′,6-diamidino-2-phenylindole) counterstaining indicates nuclear localization (blue, λem 420–480, λex 405 nm). B545b fluorescence was collected at 560–620 nm with λex 543 nm. ***P < 0.001, **P < 0.01, and *P < 0.1 versus control. | |
Next, the selectivity of B545b for ONOO− in live endothelial cells was studied. For this purpose, cells were first treated with ONOO− scavengers,36–38 and then were stimulated with SIN-1 or OGD. The results showed that neutralizing ONOO− with these scavengers significantly decreased SIN-1 or OGD induced B545b fluorescence (Fig. 4), suggesting the selectivity of B545b for ONOO− in live cells.
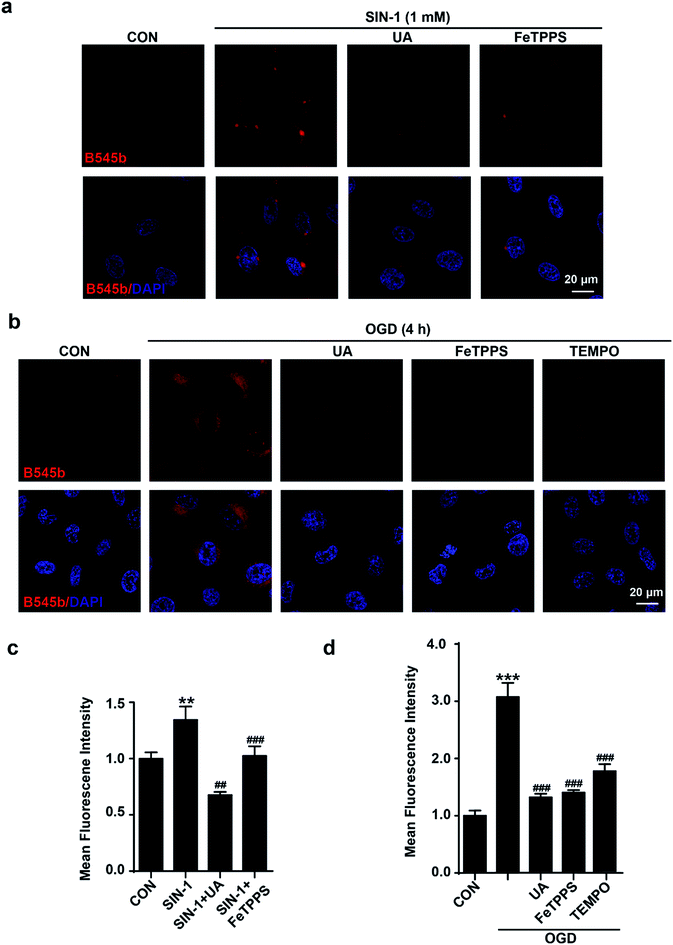 |
| Fig. 4 Characterization of B545b specificity for ONOO− in endothelial cells. (a) Scavenging ONOO− with uric acid (100 μM) or FeTPPS (1 μM) inhibited SIN-1 induced B545b fluorescence. (b) Pretreating cells with uric acid (100 μM), FeTPPS (1 μM) or TEMPO (300 μM) 1 h prior to OGD exposure (4 h) significantly suppressed B545b fluorescence. (c and d) Quantification of B545b mean fluorescence intensity (mean ± S.E.M, n = 4) in (a) and (b). Data are presented as a densitometric ratio change compared with control. DAPI counterstaining indicates nuclear localization (blue, λex 405 nm and λem 420–480 nm). B545b fluorescence was collected at 560–620 nm with λex 543 nm. ***P < 0.001, **P < 0.01 versus control, ###P < 0.001, and ##P < 0.01 versus SIN-1 or OGD. | |
In vivo real-time imaging of the ONOO− flux incurred by brain microvessel occlusion
Having confirmed the sensitivity and selectivity of probe B545b for ONOO− in live cells, and its good BBB permeability, we then tested its ability to image ONOO− induced by brain vascular injury in mice. First, a photothrombosis-induced focal ischemia model was used to evaluate its ability to track the ONOO− flux incurred by brain microvessel occlusion in live mice. For this purpose, B545b was administered to mice through tail injection. One hour later, the mice were subjected to laser irradiation to induce microvessel occlusion and hence ischemic injury.39 Simultaneously B545b fluorescence in the occluded area was recorded using an in vivo two-photon laser scanning microscope (TPLSM) (Fig. 5a). As shown in Fig. 5b, which represents the time series of images from a continuous time-lapse movie (S1), B545b fluorescence was switched on simultaneously as the intravascular clot occurred, suggesting the immediate overproduction of ONOO− in response to clot formation. Interestingly, a trace amount of B545b fluorescence was also observed around the clotted region once the clot was formed but decreased soon as ischemia progressed, leaving the clotted site which is the major site of ONOO− overproduction. This result suggests that B545b can efficiently detect the burst of pathologic ONOO− and can visualize the dynamic changes of ONOO− in a temporally and spatially resolved way. The result also provides the direct proof for the instant overproduction of ONOO− in vascular injury.
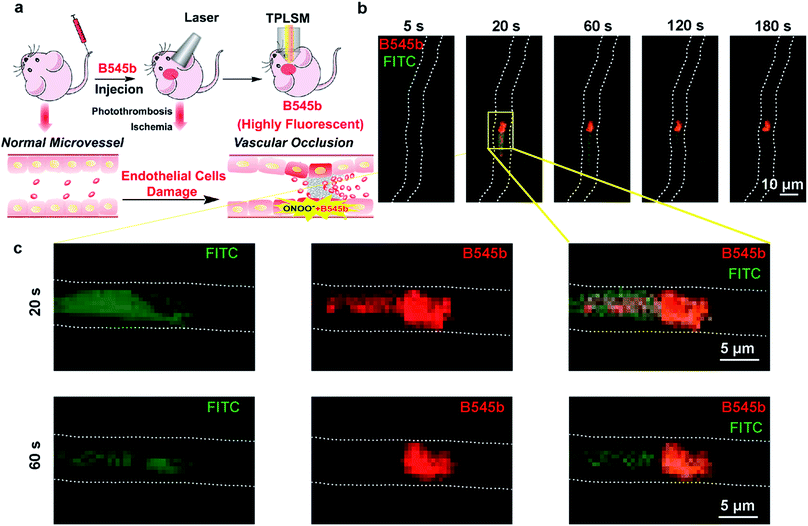 |
| Fig. 5
In vivo real-time imaging of the endogenous ONOO− flux incurred by brain microvessel occlusion. (a) Schematic illustration of experimental methods for microvessel occlusion and imaging. First, probe B545b and FITC–dextran were intravenously injected into mice. Then, microvessel occlusion was induced by photothrombosis, and imaged using a two-photon laser scanning microscope (TPLSM). (b) The time-series images representing individual frames from a continuous time lapse movie which showed the dynamic B545b fluorescence change in response to vascular occlusion in live mice. (c) Individual fluorescence of B545b and FITC in the photothrombosis model. | |
Detecting ONOO− production in the brain parenchyma after vascular injury
Having confirmed the ability of B545b to track the burst of ONOO− in the clotted microvessel region, we then tested whether it could also detect ONOO− production in the brain parenchyma after vascular injury. For this purpose, an intraluminal middle cerebral artery occlusion (MCAO) model was used to induce cerebral infarction.40 Mice were administered with B545b through tail injection. 20 min later, the mice were subjected to transient MCAO of 0.5 h or 1 h. An in vivo imaging system (IVIS) showed the MCAO-related activation of B545b fluorescence in the ipsilateral side of brain 0.5 h after MCAO (Fig. 6a). Ischemic injury evokes a cellular stress response, which involves the expression of FasL. As shown in Fig. 6b, an elevation in FasL expression was observed in MCAO mice, which indicated the damage induced by ischemic injury.41 Observation of the tissues under a confocal microscope (Fig. 6c) revealed that B545b fluorescence increased mainly in the cortex and partly in the striatum of the left brain. There was no significant Nissl staining in the ipsilateral brain at least 0.5 and 1 h after ischemia (Fig. 6d). The control group with B545b administration but no MCAO treatment demonstrated negligible B545b fluorescence while B545b fluorescence was significantly improved in the brain tissues from MCAO-treated mice. This result suggests that a transient ischemia of 30 min, even without reperfusion injury, is sufficient enough to cause nitrosative stress injury to the brain parenchyma. It should be noted that an MCAO of 60 min gave weaker B545b fluorescence than an MCAO of 30 min, which may be due to the quick metabolism of B545b, as shown in Fig S12.†
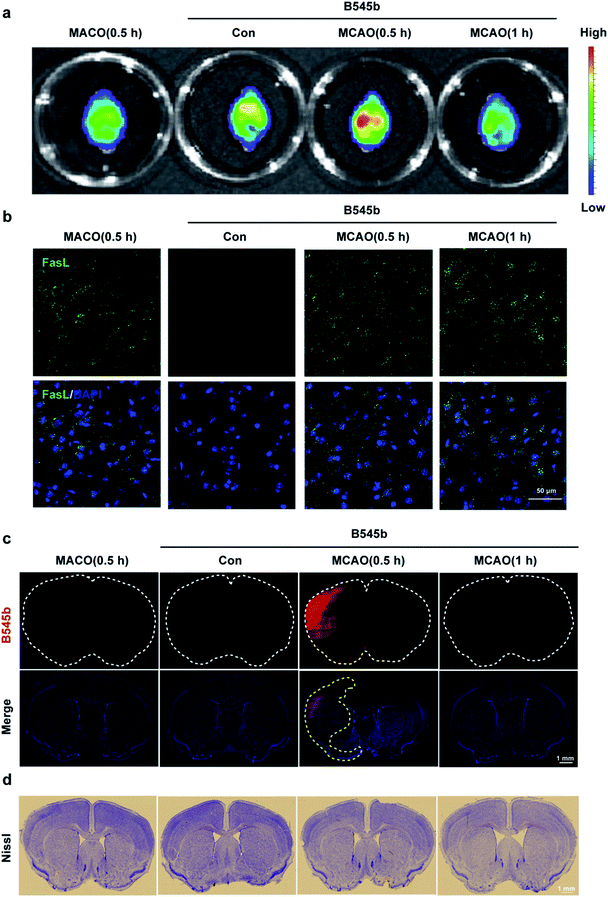 |
| Fig. 6 Imaging of ONOO− accumulation after MCAO in the brain parenchyma. (a) Cerebral imaging and analysis. After B545b administration, the mice were treated with MCAO for 0.5 or 1 h. The whole brains were scanned using a Maestro in vivo imaging system with a 480 nm excitation wavelength and a 560 nm filter. (b) Immunofluorescence staining of FasL (green) and DAPI (blue) in the brain after B545b injection and MCAO treatment. Scale bar = 50 μm. (c) Confocal imaging of brain slices with the B545b signal (red) and DAPI (blue). B545b fluorescence was collected at 500–540 nm with λex 488 nm. B545b fluorescence significantly increased 0.5 h after MCAO in the ischemic brain. The white dotted lines show the brain sections and the yellow dotted lines show the ischemic region of brain. Scale bar = 1 mm. (d) Nissl staining with the whole brain sections. Scale bar = 1 mm. | |
Conclusions
In summary, using a physicochemical-property guided probe design strategy, we have developed a fluorogenic probe B545b for imaging ONOO− during the onset of stroke. The probe demonstrates an extremely weak background signal but significant brightness upon ONOO− stimulation, which makes it sensitive enough to trap endogenously produced pathological ONOO−. Moreover, due to its desirable physicochemical properties including its partition coefficient between water and oil and moderate water solubility, B545b can be administered intravenously to mice and it readily penetrates the brain blood barrier. These advantages make B545b sensitive enough to track the ONOO− flux in clotted microvessels, and to image ischemia-induced brain parenchymal damage. Facilitated by B545b, immediate ONOO− overproduction accompanying thrombus formation was observed. Moreover, ONOO− overproduction in the brain parenchyma was also observed at the early stage of ischemia. This information should be helpful for the design of future anti-oxidant therapy for stroke. These results highlight B545b as a promising tool to probe the molecular role of ONOO− in the progression of neurovascular injury in stroke.
Conflicts of interest
There are no conflicts to declare.
Ethical statement
All animal procedures were performed in accordance with the Guidelines for Care and Use of Laboratory Animals of Zhejiang University and Experiments were approved by the Ethics Committees for Animal Experiments of Zhejiang University.
Acknowledgements
This work was supported in part by the National Natural Science Foundations of China (21778048, 21642007, 81473202, 81573411, and 81225022), the Zhejiang Provincial Natural Science Foundation of China (R18H300001 and Z16H310003), and the National Science & Technology Major Project “Key New Drug Creation and Manufacturing Program” (2018ZX09711002-010-004).
Notes and references
- G. J. Hankey, Lancet, 2017, 389, 641–654 CrossRef.
- A. Chamorro, U. Dirnagl, X. Urra and A. M. Planas, Lancet Neurol., 2016, 15, 869–881 CrossRef CAS.
- E. H. Lo, M. A. Moskowitz and T. P. Jacobs, Stroke, 2005, 36, 189–192 CrossRef PubMed.
- N. Fukuyama, S. Takizawa, H. Ishida, K. Hoshiai, Y. Shinohara and H. Nakazawa, J. Cereb. Blood Flow Metab., 1998, 18, 123–129 CrossRef CAS.
- C. Szabo, H. Ischiropoulos and R. Radi, Nat. Rev. Drug Discovery, 2007, 6, 662–680 CrossRef CAS.
- A. Chamorro, J. Stroke, 2018, 20, 197–207 CrossRef.
- S. Amaro, D. Canovas, M. Castellanos, J. Gallego, J. Marti-Febregas, T. Segura and A. Chamorro, Int. J. Stroke, 2010, 5, 325–328 CrossRef.
- R. Radi, Proc. Natl. Acad. Sci. U. S. A., 2018, 115, 5839–5848 CrossRef CAS.
- R. Radi, J. Biol. Chem., 2013, 288, 26464–26472 CrossRef CAS.
- J. Chan, S. C. Dodani and C. J. Chang, Nat. Chem., 2012, 4, 973–984 CrossRef CAS.
- D. Wu, A. C. Sedgwick, T. Gunnlaugsson, E. U. Akkaya, J. Yoon and T. D. James, Chem. Soc. Rev., 2017, 46, 7105–7123 RSC.
- D. Cheng, Y. Pan, L. Wang, Z. Zeng, L. Yuan, X. Zhang and Y. T. Chang, J. Am. Chem. Soc., 2017, 139, 285–292 CrossRef CAS.
- J. B. Li, L. Chen, Q. Wang, H. W. Liu, X. X. Hu, L. Yuan and X. B. Zhang, Anal. Chem., 2018, 90, 4167–4173 CrossRef CAS PubMed.
- Y. Wu, A. Shi, Y. Li, H. Zeng, X. Chen, J. Wu and X. Fan, Analyst, 2018, 143, 5512–5519 RSC.
- J. Zhang, X. Zhen, P. K. Upputuri, M. Pramanik, P. Chen and K. Pu, Adv. Mater., 2017, 29, 1604764 CrossRef.
- C. Yin, H. Zhu, C. Xie, L. Zhang, P. Chen, Q. Fan, W. Huang and K. Pu, Adv. Funct. Mater., 2017, 27, 1700493 CrossRef.
- J. Huang, J. Li, Y. Lyu, Q. Miao and K. Pu, Nat. Mater., 2019, 18, 1133–1143 CrossRef CAS.
- D. Cheng, J. Peng, Y. Lv, D. Su, D. Liu, M. Chen, L. Yuan and X. Zhang, J. Am. Chem. Soc., 2019, 141, 6352–6361 CrossRef CAS.
- A. J. Shuhendler, K. Pu, L. Cui, J. P. Uetrecht and J. Rao, Nat. Biotechnol., 2014, 32, 373–380 CrossRef CAS.
- T. Peng, X. Chen, L. Gao, T. Zhang, W. Wang, J. Shen and D. Yang, Chem. Sci., 2016, 7, 5407–5413 RSC.
- Y. Li, X. Xie, X. Yang, M. Li, X. Jiao, Y. Sun, X. Wang and B. Tang, Chem. Sci., 2017, 8, 4006–4011 RSC.
- T. Peng, N. K. Wong, X. Chen, Y. K. Chan, Z. Sun, J. J. Hu, J. Shen, H. El-Nezami and D. Yang, J. Am. Chem. Soc., 2014, 136, 11728–11734 CrossRef CAS.
- X. Xie, F. Tang, G. Liu, Y. Li, X. Su, X. Jiao, X. Wang and B. Tang, Anal. Chem., 2018, 90, 11629–11635 CrossRef CAS.
- S. A. Hitchcock and L. D. Pennington, J. Med. Chem., 2006, 49, 7559–7583 CrossRef CAS.
- K. Setsukinai, Y. Urano, K. Kakinuma, H. J. Majima and T. Nagano, J. Biol. Chem., 2003, 278, 3170–3175 CrossRef CAS.
- T. Peng, N. K. Wong, X. Chen, Y. K. Chan, Z. Sun, J. J. Hu, J. Shen, H. El-Nezami and D. Yang, J. Am. Chem. Soc., 2014, 136, 11728–11734 CrossRef CAS.
- X. Li, R. R. Tao, L. J. Hong, J. Cheng, Q. Jiang, Y. M. Lu, M. H. Liao, W. F. Ye, N. N. Lu, F. Han, Y. Z. Hu and Y. H. Hu, J. Am. Chem. Soc., 2015, 137, 12296–12303 CrossRef CAS.
- T. Peng, X. Chen, L. Gao, T. Zhang, W. Wang, J. Shen and D. Yang, Chem. Sci., 2016, 7, 5407–5413 RSC.
- K. E. Knewtson, D. Rane and B. R. Peterson, ACS Chem. Biol., 2018, 13, 2595–2602 CrossRef CAS PubMed.
- D. Li, J. Cheng, C. K. Wang, H. Ying, Y. Hu, F. Han and X. Li, Chem. Commun., 2018, 54, 8170–8173 RSC.
- T. Rohand, M. Baruah, W. Qin, N. Boens and W. Dehaen, Chem. Commun., 2006, 266–268 RSC.
- A. M. Hakim and E. A. Shoubridge, Cerebrovasc. Brain Metab. Rev., 1989, 1, 115–132 CAS.
- U. Bickel, NeuroRx, 2005, 2, 15–26 CrossRef.
- J. H. Jang, O. I. Aruoma, L. S. Jen, H. Y. Chung and Y. J. Surh, Free Radical Biol. Med., 2004, 36, 288–299 CrossRef CAS.
- J. Xu, L. He, S. H. Ahmed, S. W. Chen, M. P. Goldberg, J. S. Beckman and C. Y. Hsu, Stroke, 2000, 31, 1744–1751 CrossRef CAS.
- D. C. Hooper, S. Spitsin, R. B. Kean, J. M. Champion, G. M. Dickson, I. Chaudhry and H. Koprowski, Proc. Natl. Acad. Sci. U. S. A., 1998, 95, 675–680 CrossRef CAS.
- T. P. Misko, M. K. Highkin, A. W. Veenhuizen, P. T. Manning, M. K. Stern, M. G. Currie and D. Salvemini, J. Biol. Chem., 1998, 273, 15646–15653 CrossRef CAS.
- R. T. Carroll, P. Galatsis, S. Borosky, K. K. Kopec, V. Kumar, J. S. Althaus and E. D. Hall, Chem. Res. Toxicol., 2000, 13, 294–300 Search PubMed.
- M. Schroeter, S. Jander and G. Stoll, J. Neurosci. Methods, 2002, 117, 43–49 CrossRef.
- N. Shioda, T. Ishigami, F. Han, S. Moriguchi, M. Shibuya, Y. Iwabuchi and K. Fukunaga, Neuroscience, 2007, 148, 221–229 CrossRef CAS.
- Y. M. Lu, R. R. Tao, J. Y. Huang, L. T. Li, M. H. Liao, X. M. Li, K. Fukunaga, Z. H. Hong and F. Han, J. Neuroinflammation, 2012, 9, 172 CAS.
Footnotes |
† Electronic supplementary information (ESI) available. See DOI: 10.1039/c9sc03798e |
‡ These authors contributed equally to this work. |
|
This journal is © The Royal Society of Chemistry 2020 |