DOI:
10.1039/D0RA07732A
(Paper)
RSC Adv., 2020,
10, 41078-41087
Enhanced stability and nitrogen removal efficiency of Klebsiella sp. entrapped in chitosan beads applied in the domestic sewage system
Received
9th September 2020
, Accepted 2nd November 2020
First published on 11th November 2020
Abstract
Although numerous denitrifying bacteria have been isolated and characterized, their capacity is seriously compromised by traditional inoculant addition and environmental stress in open bioreactors for wastewater treatment. In this study, a biocompatible material, chitosan, was used as a carrier to immobilize a simultaneously heterotrophic nitrifying–aerobic denitrifying bacterium Klebsiella sp., KSND, for continuous nitrogen removal from domestic wastewater in an open purification tank. The results showed that immobilization had no significant effect on cell viability and was beneficial for the reproduction and adhesion of cells. The entrapped KSND exhibited a slightly higher nitrogen removal efficiency of 90.09% than that of free KSND (87.69%). Subsequently, repeated batch cultivation experiments and analysis of the effects of organic contaminants and metal ions were performed using artificial wastewater and domestic wastewater. The findings revealed that the immobilized KSND beads presented desirable biophysical properties with good mechanical stability, cell viability, and enrichment, remarkable stability in organic contaminants and metal ions, and high efficiency nitrogen removal capacity. In conclusion, the developed immobilized denitrifying bacteria system has great potential for continuous wastewater treatment in open bioreactors.
1. Introduction
The nitrogen cycle is a critical biogeochemical cycle in aquatic and terrestrial ecosystems.1 For decades, excessive inorganic nitrogen generated from human activities has been discharged into the aquatic environment, resulting in adverse effects on the environment2 and human health.3–5 Therefore, lowering nitrogen levels in wastewater is important and necessary. Biological nitrogen removal is regarded as the most common, efficient, and cost-effective method for wastewater treatment owing to the critical biochemical process for converting nitrogenous compounds to nitrogen gas.6 Traditional biological treatment is dependent on aerobic nitrification and anaerobic denitrification by complex microbial community; however, it has many disadvantages such as large quantity of sludge, low efficiency, time-consuming process, additional carbon sources requirement, etc. For decades, a large number of microorganisms involved in nitrogen metabolism, such as nitrifying, denitrifying, and ammonia-oxidizing bacteria, have been isolated and identified.6,7 These organisms have been demonstrated to be potentially useful wastewater treatment agents. Recently, a group of bacteria capable of simultaneous nitrification and denitrification have been identified, which exhibited higher growth rates and nitrogen removal efficiency than autotrophs and well-known nitrifying and denitrifying bacteria, and can use organic substrates as sources of carbon and energy to convert ammonium into nitrogenous gas under aerobic conditions.6
To date, numerous bacteria with excellent capability of inorganic nitrogen removal have been reported, and research focused on separation, identification, culture, and application of single functional strain has been conducted.8,9 Many studies have demonstrated that direct inoculation of liquid or solid bacterial seed, combined with additional nutrients, allows the reaction system to immediately attain efficient nitrogen removal capacity in a short period. However, this apparent effect usually lasts only for a short duration, and subsequently disappears.10 This outcome is mainly owing to the continuous water inflow and washout in the sewage treatment system, oligotrophy of the available nutrients, toxicity of pollutants, competition of other microbial species in the open environment,11 etc., making it difficult to maintain long-term colonization and effect with the addition of bacterial cultures. Plastic sponge fillers have been widely used for microbial attachment in biochemical systems to facilitate biofilms growth.12 However, besides the high cost of these materials, their surface hydrophobic property is not favorable for firm adhesion of microbial cells. Therefore, despite the identification of numerous excellent strains with promising potential applications in novel technologies and approaches for wastewater treatment, only a few studies have focused on how to enhance efficient colonization and long-term stabile application of strains in open and unattended biochemical systems.6,13
Cell immobilization is physical fixation of viable cells in a defined region to catalyze a specific reaction with no loss of activity with repeated use,14 and has been widely used in industrial-scale microbial fermentation and biocatalysis. Immobilization allows recurrent use and long-term stability of the cells, facilitating maintenance of high cell density and low loss of activity during the bioprocess,15 and support materials provide protection for the viability and stability of cells against harsh environments (e.g., shear stress, pH, organic contaminants, etc.).16 Recently, hydrophilic synthetic polymers (such as polyacrylate, acrylamide, polyurethane, polyvinyl, and resins) and/or natural polymers (such as alginate, carrageenin, and agarose) have been used for the immobilization of microalgae and activated sludge for application in wastewater treatment,10,17 which exhibit excellent biocompatibility and physical properties. The immobilized hydrocarbon-degrading bacteria and hydrogen-producing bacteria have presented immense potential for crude oil-contaminated seawater treatment and wastewater fermentation.18,19 However, studies on the effective use of immobilized encapsulation systems for nitrifying and denitrifying bacteria, especially evaluation of their performance in domestic sewage treatment in open bioreactor systems, are limited.
In this study, a simple cell immobilization system with chitosan was employed to encapsulate the simultaneously heterotrophic nitrifying–aerobic denitrifying bacterium Klebsiella sp. KSND, which has excellent performance in nitrogen removal from low C/N domestic wastewater. Chitosan was selected as the solid matrix owing to its advantages such as simple immobilization, biocompatibility, high porous structure, hydrophilic characteristic, and good mechanical properties. The feasibility and performance of chitosan-immobilized KSND beads were investigated, including analysis of the effects of encapsulation system on cell growth and nitrogen removal capacity, continuous batch experiments to determine the reusability of the beads, as well as investigation of the influence of environmental factors on KSND cells. Furthermore, the total nitrogen (TN) removal capability of the biological purification tank containing the immobilized cells applied in domestic sewage treatment was evaluated.
2. Materials and methods
2.1 Materials
The simultaneously heterotrophic nitrifying–aerobic denitrifying bacterial strain Klebsiella sp. KSND was used in this study.20 Domestic wastewater from the living quarters of Zhejiang A & F University was employed as the influent raw sewage for all the experiments. The initial concentrations of TN, ammonia nitrogen (NH4+-N), and chemical oxygen demand (COD) in the influent domestic wastewater were approximately 36–48, 28–43, and 103–188 mg L−1 respectively, with undetectable amounts of nitrite and nitrate. The pH was approximately 7.0–7.2 and temperature was 20 ± 1.7 °C.
2.2 Media and growth conditions
The seed medium consisted of the following (per L): 5 g, glucose; 0.5 g, K2HPO4; 0.5 g, KH2PO4; 0.5 g, MgSO4; 2 mL, trace element solution; 1% yeast powder solution; and 1% peptone solution. To obtain seed culture, KSND cells were precultured in seed medium at 30 °C overnight, collected, and washed with sterilized phosphate buffer. Luria-Bertani agarose medium (LB, containing 10 g L−1 peptone, 5 g L−1 yeast, 10 g L−1 sodium chloride, and 2% agar) was used for the activation of KSND cells. Artificial wastewater consisted of the following (per L): 5 g, glucose; 0.38 g, NH4Cl; 0.5 g, K2HPO4; 0.5 g, KH2PO4; 0.5 g, MgSO4; and 2 mL, trace element solution.6 The initial pH of all the media was adjusted to 7.0.
2.3 Preparation of immobilized KSND-SA beads
The chitosan beads were prepared as described previously.21 In brief, the chitosan solution was prepared by mixing 4% chitosan and 1% acetic acid in 60 mL of distilled water. The chitosan solution was stirred uniformly and autoclaved for 30 min at 115 °C and cooled to room temperature before use. A total of 250 mL of KSND seed cultures were centrifuged at 3000 rpm and 4 °C for 5 min, and the collected cells were washed with sterile water and resuspended in 40 mL of PBS buffer. The immobilized KSND-SA beads were obtained by dropping the chitosan–bacteria mixture into 0.25 mol L−1 NaOH solution (pH 12). Subsequently, the beads formed in the alkaline solution were washed 3 times with sterile distilled water until their pH reached 7, and were preserved in physiological saline until further use.
2.4 Cells culture
To evaluate the cell viability and nitrogen removal of chitosan-immobilized KSND cells (KSND-SA), 250 mL flasks containing 100 mL of the artificial wastewater were inoculated with 1 mL fresh seed cultures and equal amount (1 mL seed cultures for encapsulation) of KSND-SA beads, respectively, and incubated at 30 °C and 200 rpm. Samples were periodically collected and analyzed for cell growth and NH4+-N removal efficiency. To investigate the performances and stability of KSND-SA beads in successive batches, 3% (v/v) KSND-SA beads were inoculated into to 250 mL flasks containing 100 mL of artificial wastewater and domestic wastewater, respectively, and incubated at 30 °C and 200 rpm for 60 h. After the first batch experiments, the KSND-SA beads were recycled and washed with sterile water, and then inoculated into 250 mL flasks containing 100 mL of fresh artificial wastewater and domestic wastewater for the second batch experiments, respectively. All the successive batch experiments were conducted under same operation conditions as those of the first batch experiments.
2.5 Effects of metal ions and organic contaminants on the immobilized KSND-SA beads
The effects of metal ions and organic contaminants on the activity of immobilized KSND-SA beads were respectively determined. A total of 3% (v/v) immobilized KSND-SA beads and equal amount of free KSND cells were inoculated into 250 mL flasks containing 100 mL of artificial wastewater supplemented with metal ions (0.05 mM CuCl2, CoCl2, MnSO4, FeCl2, and ZnSO4, respectively) or organic contaminants (5 mg L−1 aniline, carbon tetrachloride, phenol, methanol, and formaldehyde, respectively), and incubated at 30 °C and 200 rpm for 48 h to investigate the cell viability and nitrogen removal captivity, respectively. The concentrations of NH4+-N, TN, nitrite, and nitrate and OD600 values were measured periodically. All the assays were performed in triplicate.
2.6 Application of immobilized KSND-SA beads in open biological purification tank
To further evaluate the application potential of immobilized KSND-SA beads in enlarged, open, and frequently fluid bioreactors, an open purification tank bioreactor (daily treatment capacity of 0.8 t of domestic sewage) with aerobic–anaerobic operating process was employed.6 The immobilized beads (1% (v/v)) were placed into Chamber 1 (aerobic) and Chamber 2 (anaerobic) of the bioreactor. In the control group, 1% (v/v) plastic sponge filler was placed and inoculated with equal amounts of free KSND seed culture. Domestic wastewater was continuously filled into Chamber 1 at a constant flow rate of 0.695 L min−1 and up to 103 L per day of total volume, and automatically overflowed out from Chamber 2 at the same rate. The dissolved oxygen (DO) levels of Chamber 1 and Chamber 2 were maintained at 5.0 ± 0.3 and 0.5 ± 0.2 mg L−1, respectively. The levels of TN, NH4+-N, nitrite, nitrate, and OD600 were measured periodically.
2.7 Analytical methods
The cell growth (OD600) was evaluated using an ultraviolet spectrophotometer. For determination of NH4+-N, TN, nitrate, nitrite, and COD contents,6,7 the collected water sample was centrifuged at 3000 rpm for 3 min and analyzed. 1 mL sodium tartrate (500 g L−1) and 1 mL Nessler's reagent (160 g L−1 sodium hydroxide, 70 g L−1 potassium iodide and 100 g L−1 mercury iodide) were mixed with the samples for 15 minutes incubation, and then measured at 420 nm to calculate the concentration of NH4+-N. For nitrate quantitative analysis, 1 mL of hydrochloric acid solution (1 M)and 0.1 mL sulfuric acid solution (0.8%) were mixed with the sample, the absorbance was measured at 220 nm and calibrated at 275 nm for the calculation of concentration. Nitrite nitrogen was assessed at a wavelength of 540 nm after adding 1 mL of chromogenic reagent (containing 100 mL L−1 phosphoric acid, 40.00 g L−1 sulfanilamide, and 2.00 g L−1 N-(1-naphthyl) ethylenediamine dihydrochloride) to the sample.22 The TN content was determined by alkaline potassium persulfate digestion ultraviolet spectrophotometric method. Samples were incubated at 124 °C for 30 minutes, then 5 mL of alkaline potassium persulfate solution (400 g L−1 potassium persulfate and 150 g L−1 sodium hydroxide) and 1 mL of hydrochloric acid (10%) were added, the absorbance was measured at 220 nm and 275 nm for calculating the TN concentration.23 COD concentration was determined at the wavelength of 600 ± 20 nm by fast digestion spectrophotometric method, the KSND cells were enumerated as described in our previous method.20 In brief, 1 mL of the sample solution was appropriately diluted and coated on LB agar plate, and cultured overnight at 37 °C. The randomly picked 100 clones from the cultured library were identified with specific primer pairs HAR-TF/HAR-TR of KSND hydroxylamine reductase HAR gene. The PCR was performed as follows: 94 °C for 5 min, 30 cycles of 94 °C for 1 min, 53 °C for 1 min, and 72 °C for 1 min, and a final step at 72 °C for 10 min.
2.8 Scanning electron microscopy
Scanning electron microscopy (SEM) analysis was performed as described previously.24 In brief, samples were collected from the bioreactor system and fixed in 2.5% glutaraldehyde at 4 °C for 2 h. Then, the samples were gently rinsed twice with 0.1 M phosphate buffer at pH 7.0 for 15 min. Subsequently, the samples were subjected to ethanol gradient dehydration, with various ethanol concentrations (30%, 50%, 70%, 80%, 90%, and 95% ethanol for 15 min, respectively) for two times. Finally, the samples were freeze-dried, fixed on specimen stubs, and examined under an SEM at 3.0 kV.
3. Results and discussion
3.1 Characteristics of Klebsiella sp.-entrapped chitosan beads
The chitosan beads were almost spherical and uniform in size, average diameter is 3–4 mm. The entrapment of simultaneously nitrifying–denitrifying bacterium (Klebsiella sp. strain KSND)6 into the beads did not result in any significant changes in the shape or size of the beads. The beads remained stable during shaking (200 rpm) and circulation in the aerated water experiments for 15 days. SEM analysis revealed the surface morphologies of the chitosan beads as well as microbial colonization (Fig. 1). The unloaded chitosan beads presented a porous microstructure, with a high degree of interconnectivity (Fig. 1A). In contrast, the freshly prepared immobilized KSND-SA beads exhibited fewer pores and denser surface, and the KSND cells were not observed on the surface of chitosan beads (Fig. 1B), but were encapsulated into the chitosan beads. To investigate the viability of KSND cells during entrapment, KSND-SA beads were cultured in inorganic medium for 24 h. The SEM observation showed that the surface of chitosan beads was covered with microbial cells (Fig. 1C), indicating that chitosan encapsulated and immobilized the cells in the interior with good biological compatibility. When compared with common adhesion carriers or biofilm substrates, higher cell concentrations could be obtained and maintained by immobilization and encapsulation, which can confer higher metabolic activity for the encapsulation systems than attachment systems.25 SEM analysis confirmed that KSND-SA beads were successfully constructed by the combination of chitosan and Klebsiella sp. KSND cells.
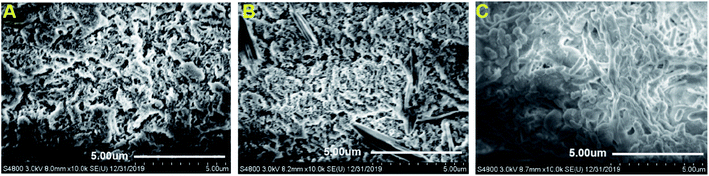 |
| Fig. 1 SEM images of the chitosan-immobilized KSND beads. The surfaces of the unloaded (A) and Klebsiella sp.-entrapped (B) chitosan beads; (C) immobilized Klebsiella sp. in chitosan after 24 h of culture. | |
3.2 Effects of immobilization on the performance of KSND cells
Fig. 2 shows the growth of free and entrapped KSND cells in shake flasks at 30 °C. The same initial counts were maintained for all the inoculants. The growth rate of the immobilized cells was significantly lower than that of the free cells. Free KSND cells showed an exponential growth trend after a short period of adjustment, with the highest OD600 values of 2.41 at 24 h (Fig. 2A). The entrapped KSND cells exhibited a longer adaptation period and slower growth trend from 6 to 48 h, and reached the highest OD600 values of 1.47 at 48 h, which was significantly lower than that of free KSND cells. Previous studies have also reported this trend on immobilized cell cultivation and that the procedures to prepare the entrapped cells are generally more detrimental and often cause cell death, resulting in longer adaptation phase.10,26 The biomass of the entrapped KSND cells in suspension appeared to be lower, which may be owing to two reasons. First, when compared with free cells, embedded KSND cells had less access to nutrients, resulting in growth restriction, and had a longer logarithmic phase owing to slower nutrients consumption,27 which also helped to conserve carbon sources for nitrogen removal. Second, more cells grew on the surfaces of the entrapment material, which was difficult to detect accurately.18 The level of NH4+-N was used to investigate the nitrifying capacity of KSND cells (Fig. 2B). The concentration of NH4+-N decreased from 104 to 12.8 mg L−1 after 24 h in free KSND cells, with a nitrogen removal efficiency and rate of 87.69% and 3.80 mg L−1 h−1, respectively. However, the entrapped KSND-SA cells showed slightly higher nitrification efficiency of 90.09% after 24 h, with a nitrogen removal rate of 3.91 mg L−1 h−1. These results indicated that although the entrapped cells grew more slowly than suspended cells, the immobilized cells were more metabolically active and exhibited higher nitrogen removal efficiency, which is consistent with previous reports.28 Besides the abundant microbial colonization on the surface of the materials, the effects of nutrients adsorption and enrichment by the entrapment materials may also be one of the major mechanisms involved in efficient ammonium removal.29
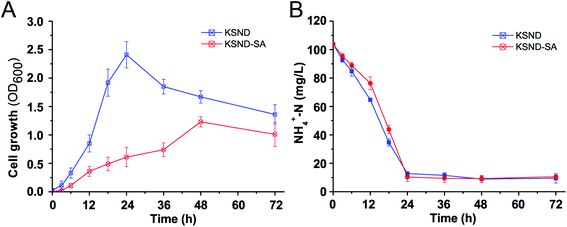 |
| Fig. 2 Evaluation of cell growth (A) and nitrification capacity (B) of immobilized cells. KSND: suspended free cells of Klebsiella sp. KSND strain; KSND-SA: chitosan-immobilized Klebsiella sp. KSND cells. | |
3.3 Stability and bioactivity assessments of immobilized beads in repeated batch experiments
To study the effects of recycling of the immobilized beads on nitrogen removal, artificial wastewater, made up of modified mineral basal medium containing excess amounts of inorganic nitrogen (C/N 5
:
1), and domestic sewage (C/N 4
:
1) were respectively used with immobilized beads in continuous batch experiments. As shown in Fig. 3, during the first five batch experiments, cell growth in artificial wastewater presented an obvious increasing trend, resulting in an increase in cell density (OD600) from 0.24 (first batch) to 0.51 (fourth batch) in mid-log phase (24 h) (Fig. 3A). The maximum growth was reached at 48 h in the fourth batch with an OD600 of 1.43, and the maximum biomass concentration in the first 8 batches reached OD600 of more than 0.8. Importantly, the cell growth characteristics of the beads indicated increased metabolic activity with successive batches, implying that chitosan-immobilized Klebsiella sp. KSND cells had very stable colonization viability, making them suitable for application in open-flow systems. Meanwhile, the nitrogen removal capacity of each batch was evaluated with the initial 100 mg L−1 NH4+-N. As illustrated in Fig. 3B, the nitrogen removal rates for the first batch were only 34%, 63%, and 85% at 24, 48, and 60 h, respectively. However, the nitrogen removal rates from second to fifth batches exhibited a significant improvement at 24 h, reaching 62–78%, and the subsequent batches showed high nitrogen removal efficiency of more than 90%. In the ninth batch, although the cell viability and nitrogen removal efficiency decreased, the immobilized cells exhibited competent nitrogen removal for a month of continuous repeated batch cultivation process.
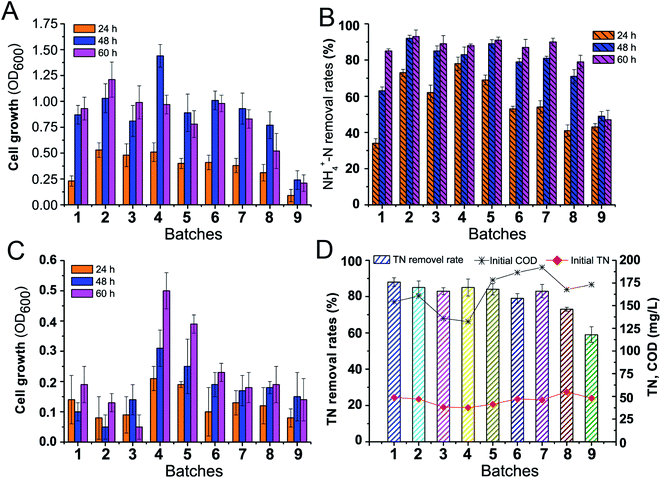 |
| Fig. 3 Performance of immobilized KSND-SA beads in successive repeated batch experiments. Cell growth (A) and NH4+-N removal (B) in artificial wastewater; cell growth (C) and TN removal (D) in domestic wastewater. | |
Domestic sewage presented poor nutritional conditions for evaluation, as shown in Fig. 3C, and the cell growth was markedly inhibited, resulting in lower biomass yields. The maximum OD600 values of the cells during the first three batch experiments were only 0.19, 0.13, and 0.14, respectively, and the highest value (OD600 of 0.49) was noted in the fourth batch, which was distinctly lower than that observed in artificial wastewater. This result was somewhat expected because cell growth was compromised owing to nutrients mass amount and availability in domestic sewage. However, the TN removal efficiencies of the chitosan-immobilized Klebsiella sp. KSND cells in each batch remained stable at 80% (Fig. 4D). Although the nitrogen removal efficiency was negatively affected after eight batches of successive recycling of the immobilized cells, it remained at more than 60%. This nearly stable nitrogen removal performance of the immobilized cells suggested the high operation stability of these cells regardless of the changes in the nutritional conditions.30 Moreover, the high-concentration cells embedded in the beads could effectively prevent the loss and contamination of microorganisms in wastewater,31 especially in the form of seed storage for constant replenishment of the microbial population.
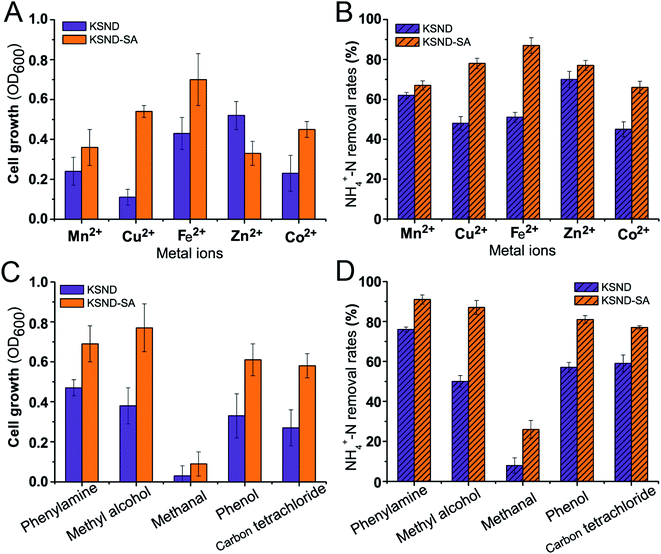 |
| Fig. 4 Effects of metal ions and organic solvents on cell activity. Cell growth (A) and NH4+-N removal (B) were determined following treatments with 5 mM metal ions (Mu2+, Cu2+, Fe2+, Zn2+, and Co2+, respectively). Cell growth (C) and NH4+-N removal (D) were evaluated following treatments with phenylamine, methyl alcohol, methanal, phenol, and carbon tetrachloride, respectively. (The free KSND cells incubated in artificial wastewater only was used as the measure of 100% nitrogen removal efficiency activity.) | |
3.4 Effects of metal ions and organic contaminants on the activity of immobilized cells
The metabolic activity of microorganisms is often disturbed by many factors in sewage, such as pH, heavy metal ions, organic contaminants, etc., which seriously affects the degradation efficiency of pollutants. In the present study, artificial wastewater, made-up of domestic wastewater containing 5 mM of metal ions and organic contaminants, was used to evaluate the cell growth and nitrogen removal of the immobilized cells. As shown in Fig. 4A, the cell growth of free Klebsiella sp. KSND cells was significantly inhibited by 5 mM of Mn2+, Cu2+, Fe2+, Zn2+, and Co2+ ions, resulting in obvious decrease in OD600 values to 0.23, 0.11, 0.43, 0.55, and 0.19, respectively. As expected, the nitrogen removal capacity of free KSND cells was also affected by Mn2+, Cu2+, Fe2+, Zn2+, and Co2+, reducing the nitrogen removal efficiency to 63.1%, 46.8%, 50.7%, 71.3%, and 44.8%, respectively (Fig. 4B). It can be noted from Fig. 4A and B that the immobilized cells presented higher biomass concentration and nitrogen removal efficiency than the free KSND cells following treatments with 5 mM Mn2+, Cu2+, Fe2+, Zn2+, and Co2+ ions, exhibiting OD600 of 0.36, 0.54, 0.71, 0.33, and 0.45, respectively. In particular, approximately 69–89% of the original nitrogen removal efficiencies of the immobilized cells were maintained in the presence of metal ions (Fig. 4B), indicating that chitosan-immobilized Klebsiella sp. KSND cells could be used for efficient nitrogen removal even in the presence of heavy metal ions, which is potentially favorable for the treatments of certain industrial sewage.32
In general, industrial wastewater contains various kinds of organic contaminants, which also cause irreversible damage to microorganisms in water, and even result in the complete loss of microbial degradation efficiency in wastewater treatment. Therefore, in the present study, the effects of different organic contaminants on the cell viability and nitrogen removal efficiency of immobilized KSND-SA beads were further investigated using various low polarity, water-immiscible contaminants (phenylamine and carbon tetrachloride) and high polarity, water-miscible contaminants (methyl alcohol, methanal, and phenol). As shown in Fig. 4C and D, cell viability and nitrogen removal of free KSND cells were significantly inhibited by all of the organic contaminants tested. The cell growth values (OD600) of free KSND cells were only 0.27–0.47 in the presence of carbon tetrachloride, phenol, methyl alcohol, and phenylamine, respectively, and the nitrogen removal efficiency was only approximately 50–76% of the initial value. In contrast, immobilized KSND cells exhibited significantly increased stability in organic contaminants, reaching OD600 of 0.58–0.77 and retaining approximately 77–91% of the initial nitrogen removal efficiency. In the presence of methanal, free KSND cells almost lost their viability and activity, while immobilized cells exhibited obvious biodegradability, maintaining almost 27% of the nitrogen removal activity. Organic contaminants are known to exert adverse effects on the cell metabolic activity, resulting in varying degrees of toxicity.33 In the present study, the protection conferred by embedded material may have retained the activity of the immobilized KSND cells exposed to organic contaminants. Microbial cells are usually sensitive to restrictive environmental conditions and their fluctuations. Immobilization of microorganisms is an efficient and sustainable solution to overcome this problem, and provides the microorganisms with a stable and non-perturbed microenvironment structure, thus protecting them from harsh environmental conditions,34,35 such as organic contaminants, shear forces, pH, metal ions, temperature fluctuations, and toxins. Furthermore, immobilization can facilitate high biomass density and cell-seeded repository. The remarkable tolerance of immobilized KSND cells to metal ions and organic contaminants and excellent nitrogen removal capability makes them an attractive candidate for complex wastewater treatments.
3.5 Effect of immobilized KSND cells on biological purification tank performance
The biological purification tank with plastic sponge packing is widely used in domestic sewage treatment. The establishment of an ecosystem mainly depends on microorganisms adhering to the packing surface and growing into biofilms, which is closely related to the effectiveness of the wastewater treatment. The continuous water impact and dramatic fluctuation of environmental factors often cause serious damage to the microorganisms in the system. In the present study, the application potential of immobilized KSND-SA beads, instead of plastic sponge filler, in integrated biological purification tank (daily treatment capacity, 0.8 t of domestic sewage, modified DO control (Chamber 1 and Chamber 2)20) was further investigated for 30 days. As shown in Fig. 5A, the daily influent concentrations of COD, TN, and NH4+-N were approximately 103.8–187.4, 36.1–47.8, and 28.5–43.2 mg L−1, respectively. In the control group comprising plastic sponge filler inoculated with KSND seed culture (0.5%), the effluent concentrations of TN and NH4+-N slowly dropped to about 10.2 and 7.1 mg L−1 within 4 days, respectively. After 5 days of the experiment, the TN and NH4+-N removal efficiencies were stable at 80–90%, and the corresponding effluent concentrations decreased to 3.8–8.9 and 0.4–5.4 mg L−1, respectively. In the KSND-SA beads group, the effluent concentrations of TN and NH4+-N dropped to about 9.2 and 3.1 mg L−1 within 4 days, respectively (Fig. 5A), and the decline in nitrogen concentration was faster than that noted in the control group. Obviously, the TN and NH4+-N removal efficiencies were stable at 80–94.6%, which were higher than those found in the control group. This higher nitrogen removal efficiency of immobilized KSND cells may be owing to the ability of the immobilized cells to withstand continuous flow of water and external factors.36 Moreover, the beads contain a large number of KSND cells that can serve as a seed storage area37 and continuously provide high vitality seed to maintain the abundance of bacteria in the system.38
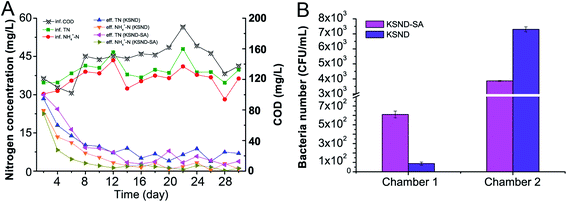 |
| Fig. 5 Application potential of immobilized KSND-SA beads in open biological purification tank. (A) Nitrogen source removal and stability of immobilized beads in domestic sewage treatment. (B) Population of KSND (free) and immobilized KSND-SA beads in aerobic Chamber 1 and anaerobic Chamber 2 of the purification tank. | |
Therefore, the populations of KSND cells in both the systems were investigated. As shown in Fig. 5B, after 30 days, the abundance of free KSND cells inoculated into Chamber 1 remained at a low level of about 0.87 × 102 CFU mL−1 in the later stage of the experiment. However, the population of immobilized KSND cells was higher reaching 6.1 × 102 CFU mL−1 in Chamber 1, which was about 8-fold higher than that noted in the control group. This result suggested that immobilized KSND cells in aerobic stage (Chamber 1) had a stronger NH4+-N removal capacity, which was also reflected in the effluent NH4+-N concentration in both the groups (Fig. 5A). As expected, the abundances of KSND cells in anaerobic stage (Chamber 2) were obviously higher than that in the aerobic stage (Chamber 1), reaching approximately 3.87 × 103 CFU mL−1 in the control group and 7.30 × 103 CFU mL−1 in the immobilized KSND cells group. This finding implied that the immobilized KSND cells group was able to maintain a higher KSND microbial population in the open bioreactor system, which was very favorable for TN removal.39 This increase in the microbial abundance in the immobilized KSND cells group might be owing to the following reasons. First, the hydrophilic surface of the immobilized material provides a firm attachment for microbial colonization, preventing washout of microbial cells.40 Second, the cells embedded in the immobilized beads provide a continuous source of microbial seeds.41 Subsequently, the physical–chemical structural stability of the immobilized cells was examined. The volumes and mechanical properties of the immobilized KSND-SA beads remained nearly the same as those noted at the initial state (Fig. 6A), with no indication of any fatigue and abrasion of the beads within the first 30 days (Fig. 6B). Even after 3 months in open biological purification tank without any supervision, the mechanical properties of the immobilized beads remained intact. These results indicated that chitosan is an excellent immobilization material that can be used as an efficient embedding medium and adhesion substrate for microorganisms, and has potent resistance toward abrasion in air-driven reactors,31 providing a low-cost and efficient alternative for plastic sponge filler in wastewater treatment.
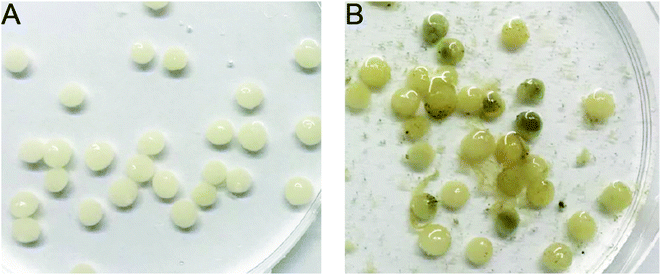 |
| Fig. 6 Immobilized KSND-SA beads. (A) Freshly prepared beads; (B) beads incubated in open purification tank after 30 days. | |
4. Conclusion
In this study, the use of a nontoxic and nonpolluting material, chitosan, for the immobilization of a simultaneously heterotrophic nitrifying–aerobic denitrifying bacterium Klebsiella sp. KSND was investigated. The results showed that the cells were encapsulated and immobilized in the interior of the chitosan beads, and that immobilization had no effect on cell viability and nitrogen removal capacity. Successive repeated batch cultivation of the immobilized cells showed that KSND cells maintained a stable cell density and nitrogen removal efficiency in both artificial and domestic wastewaters. The immobilized system was more efficient in cumulatively removing nitrogen from domestic wastewater containing metal ions or organic contaminants than free KSND cells. When compared with conventional plastic sponge packing system, the colonization, abundance, and nitrogen removal efficiency of the immobilized KSND beads were significantly higher, with no mechanical abrasion during continuous domestic wastewater treatment process for 30 days in open biological purification tank. Taken together, application of bacteria in the form of capsule-based-inoculant could provide a protective niche to the bacteria for high-efficiency wastewater treatment.
Conflicts of interest
The authors declare that they have no known competing financial interests or personal relationships that could have appeared to influence the work reported in this paper.
Acknowledgements
This work was supported by the Key R&D Program Project of Zhejiang Province, China (Grant 2020C02009).
References
- S. K. Padhi, S. Tripathy, R. Sen, A. S. Mahapatra, S. Mohanty and N. K. J. I. B. Maiti, Biodegradation, Characterisation of heterotrophic nitrifying and aerobic denitrifying Klebsiella pneumoniae CF-S9 strain for bioremediation of wastewater, Int. Biodeterior. Biodegrad., 2013, 78, 67–73 CrossRef CAS.
- Y. C. Kim, C. Gao, Y. Zheng, X. He, W. Yang, L. Chen, S. Wan and L. Guo, Arbuscular mycorrhizal fungal community response to warming and nitrogen addition in a semiarid steppe ecosystem, Mycorrhiza, 2015, 25, 267–276 CrossRef CAS.
- B. Suchetana, B. Rajagopalan and J. Silverstein, Investigating regime shifts and the factors controlling Total Inorganic Nitrogen concentrations in treated wastewater using non-homogeneous Hidden Markov and multinomial logistic regression models, Sci. Total Environ., 2019, 646, 625–633 CrossRef CAS.
- Z. Chen, X. Zhou, S. Geng, Y. Miao, Y. Cao, Z. Chen, J. Zhang and S. Han, Interactive effect of nitrogen addition and throughfall reduction decreases soil aggregate stability through reducing biological binding agents, For. Ecol. Manag., 2019, 445, 13–19 CrossRef.
- J. Xia and S. Wan, Independent effects of warming and nitrogen addition on plant phenology in the Inner Mongolian steppe, Ann. Bot., 2013, 111, 1207–1217 CrossRef.
- P. Jin, Y. Chen, Z. Zheng and Q. Du, Evaluation of a novel low-carbon to nitrogen-and temperature-tolerant simultaneously nitrifying–denitrifying bacterium and its use in the treatment of river water, RSC Adv., 2018, 8, 27417–27422 RSC.
- Y. Chen, P. Jin, Z. Cui, T. Xu, R. Zhao and Z. Zheng, Identification and characterization of Janthinobacterium svalbardensis F19, a novel low-C/N-tolerant denitrifying bacterium, Appl. Sci., 2019, 9, 1937 CrossRef CAS.
- L. Yang, Y. Ren, X. Liang, S. Zhao, J. Wang and Z. Xia, Nitrogen removal characteristics of a heterotrophic nitrifier Acinetobacter junii YB and its potential application for the treatment of high-strength nitrogenous wastewater, Bioresour. Technol., 2015, 193, 227–233 CrossRef CAS.
- P. Jin, Y. Chen, R. Yao, Z. Zheng and Q. Du, New insight into the nitrogen metabolism of simultaneous heterotrophic nitrification-aerobic denitrification bacterium in mRNA expression, J. Hazard. Mater., 2019, 371, 295–303 CrossRef CAS.
- X. Zeng, M. K. Danquah, C. Zheng, R. Potumarthi, X. D. Chen and Y. Lu, NaCS–PDMDAAC immobilized autotrophic cultivation of Chlorella sp. for wastewater nitrogen and phosphate removal, Chem. Eng. J., 2012, 187, 185–192 CrossRef CAS.
- T. P. Lam, T. M. Lee, C. Y. Chen and J. S. Chang, Strategies to control biological contaminants during microalgal cultivation in open ponds, Bioresour. Technol., 2017, 180–187 Search PubMed.
- T. Okubo, T. Onodera, S. Uemura, T. Yamaguchi, A. Ohashi and H. Harada, On-site evaluation of the performance of a full-scale down-flow hanging sponge reactor as a post-treatment process of an up-flow anaerobic sludge blanket reactor for treating sewage in India, Bioresour. Technol., 2015, 194, 156–164 CrossRef CAS.
- S. Warneke, L. A. Schipper, M. G. Matiasek, K. M. Scow, S. Cameron, D. A. Bruesewitz and I. R. McDonald, Nitrate removal, communities of denitrifiers and adverse effects in different carbon substrates for use in denitrification beds, Water Res., 2011, 45, 5463–5475 CrossRef CAS.
- K. K. Ephraim and D. M. Kraemer, Eupergit® C, a carrier for immobilization of enzymes of industrial potential, J. Mol. Catal. B: Enzym., 2000, 10, 157–176 CrossRef.
- I. Eş, J. D. G. Vieira and A. C. Amaral, Principles, techniques, and applications of biocatalyst immobilization for industrial application, Appl. Microbiol. Biotechnol., 2015, 99, 2065–2082 CrossRef.
- M. Asgher, M. Shahid, S. Kamal and H. M. N. Iqbal, Recent trends and valorization of immobilization strategies and ligninolytic
enzymes by industrial biotechnology, J. Mol. Catal. B: Enzym., 2014, 101, 56–66 CrossRef CAS.
- I.-S. Chang, C.-I. Kim and B.-U. Nam, The influence of poly-vinyl-alcohol (PVA) characteristics on the physical stability of encapsulated immobilization media for advanced wastewater treatment, Process Biochem., 2005, 40, 3050–3054 CrossRef CAS.
- A. R. Gentili, M. A. Cubitto, M. Ferrero and M. S. Rodriguéz, Bioremediation of crude oil polluted seawater by a hydrocarbon-degrading bacterial strain immobilized on chitin and chitosan flakes, Int. Biodeterior. Biodegrad., 2006, 57, 222–228 CrossRef CAS.
- L. Singh, M. F. Siddiqui, A. Ahmad, M. H. A. Rahim, M. Sakinah and Z. A. Wahid, Application of polyethylene glycol immobilized Clostridium sp. LS2 for continuous hydrogen production from palm oil mill effluent in upflow anaerobic sludge blanket reactor, Biochem. Eng. J., 2013, 70, 158–165 CrossRef CAS.
- P. Jin, Y. Chen, T. Xu, Z. Cui and Z. Zheng, Efficient nitrogen removal by simultaneous heterotrophic nitrifying-aerobic denitrifying bacterium in a purification tank bioreactor amended with two-stage dissolved oxygen control, Bioresour. Technol., 2019, 281, 392–400 CrossRef CAS.
- S. Fierro, M. del Pilar Sánchez-Saavedra and C. Copalcua, Nitrate and phosphate removal by chitosan immobilized Scenedesmus, Bioresour. Technol., 2008, 99, 1274–1279 CrossRef CAS.
- W. Chen, L. Hao, Q. Zhang and S. Dai, Effect of nitrite on growth and microcystins production of Microcystis aeruginosa PCC7806, J. Appl. Phycol., 2011, 23, 665–671 CrossRef CAS.
- Y. Shao, W. Zhang, N. Eisenhauer, T. Liu, Y. Xiong, C. Liang and S. Fu, Nitrogen deposition cancels out exotic earthworm effects on plant-feeding nematode communities, J. Anim. Ecol., 2017, 86, 708–717 CrossRef.
- E. Mirzaei B, A. Ramazani S A, M. Shafiee and M. Danaei, Studies on glutaraldehyde crosslinked chitosan hydrogel properties for drug delivery systems, J. Polym. Mater. Int. J., 2013, 62, 605–611 CrossRef CAS.
- W. M. Rostron, D. C. Stuckey and A. A. Young, Nitrification of high strength ammonia wastewaters: comparative study of immobilisation media, Water Res., 2001, 35, 1169–1178 CrossRef CAS.
- E. J. T. M. Leenen, V. A. P. D. Santos, K. C. F. Grolle, J. Tramper and R. Wijffels, Characteristics of and selection criteria for support materials for cell immobilization in wastewater treatment, Water Res., 1996, 30, 2985–2996 CrossRef CAS.
- S. Yashverry, Photosynthetic activity, and lipid and hydrocarbon production by alginate-immobilized cells of Botryococcus in relation to growth phase, J. Microbiol. Biotechnol., 2003, 13, 687–691 Search PubMed.
- P. S. Lau, N. F. Y. Tam and Y. S. Wong, Wastewater nutrients removal by carrageenan and alginate immobilized Chlorella Vulgaris, Environ. Technol. Lett., 1997, 18, 945–951 CrossRef CAS.
- L. E. De-Bashan and B. Yoav, Immobilized microalgae for removing pollutants: Review of practical aspects, Bioresour. Technol., 2010, 101, 1611–1627 CrossRef CAS.
- J. M. Prandini, M. L. B. Da Silva, M. P. Mezzari, M. Pirolli, W. Michelon and H. M. Soares, Enhancement of nutrient removal from swine wastewater digestate coupled to biogas purification by microalgae Scenedesmus spp, Bioresour. Technol., 2016, 202, 67–75 CrossRef CAS.
- H. He, Y. Chen, X. Li, Y. Cheng, C. Yang and G. Zeng, Influence of salinity on microorganisms in activated sludge processes: a review, Int. Biodeterior. Biodegrad., 2017, 119, 520–527 CrossRef CAS.
- G. Bayramoğlu, S. Bektaş and M. Y. Arıca, Biosorption of heavy metal ions on immobilized white-rot fungus Trametes versicolor, J. Hazard. Mater., 2003, 101, 285–300 CrossRef.
- S. Torres, A. Pandey and G. R. Castro, Organic solvent adaptation of Gram positive bacteria: applications and biotechnological potentials, Biotechnol. Adv., 2011, 29, 442–452 CrossRef CAS.
- X. Yang, G. Tian, N. Jiang and B. Su, Immobilization technology: a sustainable solution for biofuel cell design, Energy Environ. Sci., 2012, 5, 5540–5563 RSC.
- T. Takei, K. Ikeda, H. Ijima and K. Kawakami, Fabrication of poly (vinyl alcohol) hydrogel beads crosslinked using sodium sulfate for microorganism immobilization, Process Biochem., 2011, 46, 566–571 CrossRef CAS.
- S. El Fantroussi and S. N. Agathos, Is bioaugmentation a feasible strategy for pollutant removal and site remediation?, Curr. Opin. Microbiol., 2005, 8, 268–275 CrossRef CAS.
- A. E. S. Vercelheze, B. M. Marim, A. L. Oliveira and S. Mali, Development of biodegradable coatings for maize seeds and their application for Azospirillum brasilense immobilization, Appl. Microbiol. Biotechnol., 2019, 103, 2193–2203 CrossRef CAS.
- R. P. John, R. Tyagi, S. Brar, R. Surampalli and D. Prévost, Bio-encapsulation of microbial cells for targeted agricultural delivery, Crit. Rev. Biotechnol., 2011, 31, 211–226 CrossRef CAS.
- S. He and G. Xue, Algal-based immobilization process to treat the effluent from a secondary wastewater treatment plant (WWTP), J. Hazard. Mater., 2010, 178, 895–899 CrossRef CAS.
- J. H. Park and N. Bolan, Lead immobilization and bioavailability in microbial and root interface, J. Hazard. Mater., 2013, 261, 777–783 CrossRef CAS.
- X. Shi, G. Zhou, S. Liao, S. Shan, G. Wang and Z. Guo, Immobilization of cadmium by immobilized Alishewanella sp. WH16-1 with alginate-lotus seed pods in pot experiments of Cd-contaminated paddy soil, J. Hazard. Mater., 2018, 357, 431–439 CrossRef CAS.
Footnote |
† These authors contributed equally to this work. |
|
This journal is © The Royal Society of Chemistry 2020 |
Click here to see how this site uses Cookies. View our privacy policy here.