DOI:
10.1039/D0RA07533G
(Paper)
RSC Adv., 2020,
10, 38663-38671
Photocatalytic degradation of diphenhydramine in aqueous solution by natural dolomite†
Received
2nd September 2020
, Accepted 8th October 2020
First published on 21st October 2020
Abstract
Natural dolomite, an inexpensive and vastly available natural material, was demonstrated as a potential heterogeneous photocatalyst for the efficient removal of diphenhydramine (DP) from aqueous solution under simulated solar light in this study. About 65% DP removal and 14% mineralization were achieved with dolomite as a catalyst after 75 min irradiation. The electron spin resonance analysis and scavenger experiments verified that 1O2, ˙OH, and O2−˙ produced in the dolomite system were the main reactive species responsible for DP degradation. Furthermore, first-principle calculations combined with deoxygenation experiments were employed to elucidate the photocatalytic mechanism. The results revealed that the dolomite changed from an insulator to a semiconductor after partial substitution of Mg2+ by Fe2+, suggesting that natural dolomite could act as a semiconductor photocatalyst in photoreactions. Under irradiation, photo-excited electrons and holes separate and migrate to the surface of dolomite, and subsequently react to form reactive species resulting in the DP degradation. Product studies demonstrated that the main degradation pathways of DP included hydroxylation of the aromatic ring as well as hydroxyl radical mediated oxidation of the alkylamine side chain. This work indicated that natural dolomite could be applied in water and wastewater treatment as a promising photocatalyst.
1. Introduction
Pharmaceutically active compounds (PhACs) are a class of compounds used to prevent, control, and treat diseases and to promote human and animal health. Due to widespread consumption and incomplete removal in conventional wastewater treatment plants, PhACs are constantly introduced into the environment via discharges of wastewater and direct runoff, resulting in their occurrence in environmental waters.1–3 Prevalence and long-term persistence of trace PhACs in aquatic environments can cause potential risks to aquatic organisms and human health.4–6
Diphenhydramine (DP), an H1-histamine receptor antagonist, is commonly used to treat allergies, hives, itching and insomnia, and also used as an analgesic adjuvant in cancer pain.7 Previous reports showed its presence in wastewater influent and effluent and surface water.8,9 Therefore, to develop effective techniques for the removal of DP from aqueous solution is of great environmental interest.
In recent years, advanced oxidation processes (AOPs), as an environmental friendly technology based on the oxidation of contaminants by strong oxidizing radicals, have received great attention. Among the AOPs, UV photolysis, UV/H2O2 photolysis, Fenton and photo-Fenton oxidation, heterogenous photocatalysis, and sonolysis have been applied for the removal of DP in aqueous solution. About 26% of the initial 5 μM of DP was eliminated under the UV irradiation (1272 mJ cm−2), and adding 0.29 mM H2O2 in the photoreaction greatly improved the DP removal rate.10 Photocatalytic degradation of DP by TiO2 achieved 44.8% of DP removal under black blue lamps and 9.0% of mineralization under simulated solar radiation after 60 min irradiation, and the addition of H2O2 drastically improved the photocatalytic process, obtaining 100% DP degradation and 28.6% total organic carbon (TOC) reduction in UVC system.11 Using iron oxide nanoparticles as catalyst via a photo-Fenton process, 100% of DP elimination and 83% of TOC reduction were obtained.12 Heterogeneous photocatalysis using TiO2 and its modified forms as catalysts also exhibited high efficiency in DP degradation and mineralization.13,14 Ultrasonic irradiation at 640 kHz could effectively degrade DP in aqueous solution, and hydroxyl radical produced during cavitation played a crucial role in DP degradation.15
It appears that heterogeneous photocatalysis is a promising technique in water purification and wastewater treatment, with potential to achieve complete mineralization of organic pollutants into CO2, water, and inorganic ions under solar irradiation. Currently, design and utilization of novel photocatalytic materials have drawn great attention in the remediation of organic micropollutants and clean energy production.16–20 Dolomite is an anhydrous carbonate mineral composed of calcium magnesium carbonate, with a ideal formula of CaMg(CO3)2. Natural dolomite often contains a certain amount of iron due to the partial substitution of Mg2+ by Fe2+ in the lattice.21 As an industrial mineral, dolomite is widely used in food and pharmaceutical industries, production of fertilizers, glass and building materials, iron and steelmaking, and environmental remediation.22 No studies on photocatalytic degradation of organic pollutants by natural dolomite and their modified forms have been reported in a recent literature search by the authors. As such, the main objectives of the present study were (1) to examine the possible catalytic effects of dolomite on the photodegradation of DP in aqueous solution, and evaluate its photocatalytic performance; (2) to identify the byproducts of DP in the photoreaction and speculate the possible photolytic pathways; and (3) to elucidate the intrinsic mechanisms for the photocatalytic activities of natural dolomite.
2. Experimental
2.1 Materials
The dolomite used in this study was supplied by XBKC Co., Ltd (Shiyan, China). The diphenhydramine hydrochloride with a purity > 98%, superoxide dismutase (SOD), and TiO2 were provided by Sinopharm Chemical Reagent Co., Ltd (Shanghai, China). Acetonitrile, acetic acid, triethylamine, 1, 4-diazabicyclo [2.2.2] octane (DABCO), and isopropanol (IPA) were of high-performance liquid chromatography (HPLC) grade, and supplied by J&K Chemical Co., Ltd (Beijing, China). The spin-trapping reagents 5,5-dimethyl-1-pyrroline N-oxide (DMPO) and 2,2,6,6-tetramethylpiperidine (TEMP) were purchased from Sigma-Aldrich (Shanghai, China). All aqueous solutions were prepared with Milli-Q ultrapure water.
2.2 Pre-treatment and characterization of natural dolomite
The dolomite was first sieved to obtain a uniform 40–60 mesh sized particles. Then, the samples were washed with ultrapure water for three times, dried at 60 °C for 8 h, and stored in a desiccator for further use. No chemical treatments were made in order to maintain the natural attributes of dolomite. The morphology of samples were characterized using field emission scanning electron microscope (JEOL, JSM-6700) (Fig. S1†). The X-ray diffraction (XRD) pattern of the washed dolomite was recorded at 5° to 90° (2θ) using Bruker D8 advanced XRD instrument equipped with Cu-Kα radiation, and the collected XRD data were analyzed using MDI Jade 6.5 software. The elemental compositions of the dolomite were determined by a Rigaku-ZSX Primus II X-ray fluorescence (XRF) spectrometer. The results obtained from XRD (Fig. S2†) and XRF (Table 1) analysis indicate that it is an Fe-containing dolomite. The UV-vis diffuse reflectance spectra (DRS) of the dolomite were collected using a UV-vis spectrophotometer (UV2450, Shimadzu).
Table 1 Elemental compositions of natural dolomite determined by X-ray fluorescence spectrometer
Composition (%) |
CO2 |
MgO |
Al2O3 |
SiO2 |
P2O5 |
SO3 |
K2O |
CaO |
Fe2O3 |
55.6 |
23.4 |
0.103 |
0.304 |
0.007 |
0.026 |
0.0171 |
20.5 |
0.0774 |
2.3 Photolysis experiments
Photoreaction was carried out in a PR22-25 photochemical reactor (PerfectLight, China) equipped with a 300 W xenon light source (PLS-SXE300UV) and a water-cooling system DC-0506 (HengPing, Shanghai). The spectral range of the xenon light source is 300–800 nm, and the light power entering the photoreactor was about 120 mW cm−2. During the irradiation, the suspension was stirred continuously with the temperature kept at 4 °C by circulating water to prevent pyrolysis of DP. The reactor was airtight with a quartz glass plate on the open top of the vessel. In each experiment, 1.5 g dolomite sample was mixed with 200 mL DP solution (40 mg L−1) in the 250 mL reactor, and then magnetically stirred for 30 min, then the suspension was irradiated under simulated solar light. At the given time intervals, a 2 mL aliquot of the suspension was collected, filtered through a 0.22 μm membrane, and analyzed by HPLC and LC-MS. Dark control experiments were conducted in the same manner as the regular experiments except that they were not exposed to light. All experiments were performed in duplicates to check the results.
2.4 Analytical determination and photoproduct identification
The concentration of DP in water was determined with a Shimadzu HPLC (LC-20) equipped with a C18 ODS reversed phase column (4.6 mm × 150 mm, 5 μm) and a SPD20 UV detector. The mobile phase consisted of 25 mM acetic acid and acetonitrile (60
:
40, v/v), with pH adjusted to 6.0 ± 0.1 using triethylamine. Isocratic elution was performed at a flow rate 0.8 mL min−1. The injection volume was 20 μL. Oven temperature was kept at 30 °C and the detector wavelength was 220 nm.
Thermo Scientific Q Exactive mass spectrometer paired with the Ultimate 3000 LC system was used for the product studies. The analytical column was Agilent Zorbax XDB-C18 (2.1 mm × 50 mm, 1.8 μm). A mobile phase made of solvent A (0.1% formic acid in acetonitrile) and solvent B (0.1% formic acid in water) was used to elute the column with a flow rate 0.2 mL min−1 and the optimized gradient program 0–8 min, from 5% to 90% A; 8–10 min, 90% A. The column temperature was 30 °C. An injection volume of 5 μL was used for analytical samples. For MS detection, the operating parameters were as follows: spray voltage, 3200 V; capillary temperature, 300 °C; sheath gas pressure, 40 arb; aux gas pressure, 15 arb; S-lens RF, 50 V.
2.5 Determination of reactive species during photocatalytic degradation
In order to determine the reactive species in the photoreactions, electron spin resonance (ESR) signals for singlet oxygen, superoxide anions, and hydroxyl radicals trapped by TEMP and DMPO were obtained on a Bruker model ESP300E electron paramagnetic resonance spectrometer equipped with a Quanta-Ray Nd: YAG laser system as the irradiation light source (λ = 355 nm) at ambient temperature. The settings were center field at 3227.67 G; microwave frequency at 9054.62 MHz; and power at 0.998 mW. To further evaluate the role of these reactive species in the photodegradation of DP, reactive oxygen species (ROS) scavengers including DABCO (1O2 scavenger), IPA (˙OH scavenger) and SOD (O2−˙ scavenger) were used for the inhibition experiments.23–25
2.6 Theoretical calculations
To investigate the photocatalytic mechanism of DP under the catalysis of dolomite, the first-principle calculations were performed employing the VASP code (Vienna Ab initio Simulation Package).26 The generalized gradient approximation (GGA) method with the Perdew–Burke–Ernzerhof (PBE) functional was used for the geometry optimization and electronic band structure calculations.27,28 The kinetic energy cutoff was set as 500 eV, and a 4 × 4 × 1 k-point mesh was applied.29 Optimization was performed with a convergence threshold for the maximum energy change of 1.0 × 10−5 eV per atom and for the maximum force of 0.01 eV Å−1.
The experimental lattice constants of dolomite were a = b = 4.81 Å, c = 16.01 Å, and the space group is R
(NO. 148).30 The unit cell of dolomite in our calculations is composed of 6 C (gray), 18 O (red), 3 Mg (blue), and 3 Ca (green) atoms. And a supercell made of 2 × 2 × 1 was applied (Fig. S3(a)†). In order to simulate the partial substitution of Mg2+ by Fe2+ in natural dolomite, the Mg2+ ions in the unit cell are randomly replaced by Fe2+ ions (pink), with the Fe2+ number changing from 1 to 9. Fig. S3(b)† depicted the substitution of 3 Mg2+ by 3 Fe2+.
3. Results and discussion
3.1 Photodegradation and mineralization of DP in the presence of natural dolomite
Fig. 1 illustrates the photodegradation of DP as a function of irradiation time in the presence and absence of natural dolomite, as well as the corresponding dark controls. For the dark control without dolomite, almost negligible loss of DP within 75 min indicated that the degradation by hydrolysis or thermal degradation could be neglected. While for the dark control with dolomite, approximately 4% of DP loss was detected, which could be attributed to physical adsorption to the surface of dolomite. When exposed to simulated solar light, DP in aqueous solution in the absence of dolomite was gradually decomposed with the irradiation time, and about 12% of DP removal was achieved after 75 min irradiation. A recent study reported DP removal of 32.5, 2.5, and 1.4% after 60 min irradiation under UVC, simulated solar light, and solar irradiation, respectively.11 With the addition of dolomite into the solution, the DP removal increased to 62%, and the rate constant (KDP) estimated from the pseudo-first order kinetic model increased from 0.16 × 10−2 to 1.29 × 10−2 min−1 (Table 2). Obviously, the presence of the natural dolomite greatly promoted the photodegradation of DP, exhibiting remarkable photocatalytic activity. The TOC reduction, an indication of mineralization, follows a similar trend as observed for DP degradation, about 14% of TOC elimination after 75 min irradiation indicated that the dolomite photocatalytic process could effectively lead to the mineralization of DP (Fig. 1).
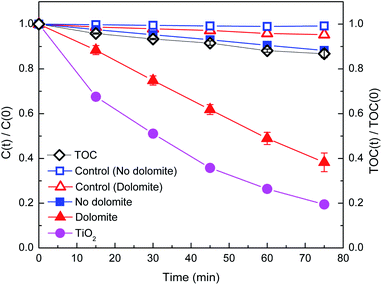 |
| Fig. 1 Photodegradation and mineralization of DP in the presence of natural dolomite under simulated solar light, as well as the corresponding dark control. The added dolomite sample is 1.5 g per 200 mL of DP solution at an initial concentration of 40 mg L−1. | |
Table 2 Fitted parameters for DP photodegradation under different reaction conditions by pseudo-first-order model
Reaction condition |
KDP (min−1) |
R2 |
t1/2 (min) |
No dolomite |
(0.16 ± 0.01) × 10−2 |
0.999 |
433.1 |
Dolomite |
(1.29 ± 0.08) × 10−2 |
0.982 |
53.7 |
TiO2 |
(2.16 ± 0.05) × 10−2 |
0.998 |
32.1 |
Dolomite + IPA |
(0.88 ± 0.05) × 10−2 |
0.99 |
78.7 |
Dolomite + SOD |
(0.82 ± 0.04) × 10−2 |
0.986 |
84.5 |
Dolomite + DABCO |
(1.03 ± 0.04) × 10−2 |
0.992 |
67.2 |
Dolomite + N2 |
(0.93 ± 0.05) × 10−2 |
0.99 |
74.5 |
To further evaluate the photocatalytic performance of dolomite, photocatalytic degradation of DP by dolomite was compared to that by TiO2 under the same conditions. Although the photocatalytic performance of dolomite was 19% lower than that by TiO2, it could still be considered as a promising photocatalyst in the field of water treatment due to its much lower material cost, vast reserves, and environmental-friendly characters. Moreover, the recyclability and stability of the dolomite were evaluated through cycling experiments. As shown in Fig. 2, the degradation rate of DP was about 63% in the first cycle, and significantly decreased to 30% in the second cycle. After 4 cycles, the dolomite almost completely lost its photocatalytic activity. This result suggested that deactivation of the dolomite could occurred after several applications.
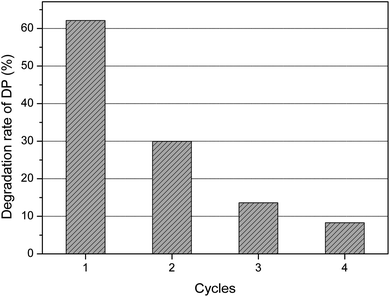 |
| Fig. 2 Photocatalytic efficiency for the natural dolomite in the cycling experiments. The irradiation time is 75 min. | |
3.2 Study of reactive oxygen species
Photo-induced ROS, such as singlet oxygen (1O2), superoxide anion radical (O2−˙), and hydroxyl radical (˙OH) were often considered as the main reactive species in photocatalytic processes. Therefore, it could be speculated that the enhanced DP degradation might also be due to the ROS produced in the dolomite system under irradiation. The EPR spin-trapping technique was deployed to examine the ROS generated in the photocatalytic process. The characteristic peaks of TEMP–1O2, DMPO–O2−˙, and DMPO–˙OH adducts are observed in the dolomite system after irradiation, and the intensity of the peaks increased gradually with the irradiation time, while no signal was detected in the dark (Fig. 3). These evidences verified the generation of 1O2, O2−˙, and ˙OH in the photocatalytic system.
 |
| Fig. 3 ESR spectral changes of the TEMP–1O2 (a), DMPO–˙OH (b), and DMPO–O2−˙ (c) adducts generated in the natural dolomite system under simulated solar light irradiation. | |
In order to quantitatively evaluate the role of ROS in the present system, scavenger experiments were performed. In the presence of SOD (O2−˙ scavenger) and IPA (˙OH scavenger), DP photodegradation was significantly suppressed (Fig. 4), indicating the dominant role of the O2−˙ and ˙OH in the photodegradation of DP. In contrast, the addition of DABCO (1O2 scavenger) showed a slight inhibition, which might be due to weak reactions between the amine drugs (including DP) and 1O2.31 Combining the EPR analyses and the radical scavenger experiments, it could be concluded that the O2−˙, 1O2, and ˙OH were the main reactive species responsible for the enhanced DP degradation in the presence of dolomite, and the O2−˙ and ˙OH played the dominant role.
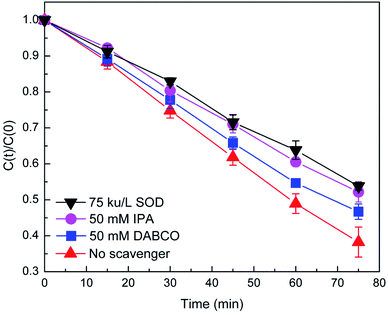 |
| Fig. 4 Suppressed photodegradation of DP in the presence of natural dolomite by the radical scavengers. The initial concentration of IPA, DABCO, and SOD were 50, 50 mM, and 75 kU L−1, respectively. | |
3.3 Mechanisms for photocatalytic activity of dolomite
In order to further elucidate the photocatalytic mechanism, density functional theory (DFT) was employed to calculate the electronic band structure and density of states (DOS) for dolomite. The calculated band gap of the pristine dolomite is 5.02 eV, consistent with a previous report of 5.035 eV (Fig. 5(a)).32 With 3 Fe2+ substitution, two new bands appear in the band structures, resulting in forbidden band gap being divided into three parts (Fig. 5(b)). Among them, the maximum band gap is 2.04 eV. With the increase of Fe2+content, more energy levels appear in the forbidden band gap, and the new bands become wider, leading to reduction of the band gap. After 9 Fe2+ substitution, the maximum band gap decreased to 1.63 eV (Fig. 5(d)), close to the band gap calculated from the UV-vis reflectance spectra (1.33 eV) of dolomite (Fig. S4†). The change in band gap of dolomite from 5.02 to 1.63 eV implies that the dolomite changes from an insulator to a semiconductor with partial substitution of Mg2+ by Fe2+.
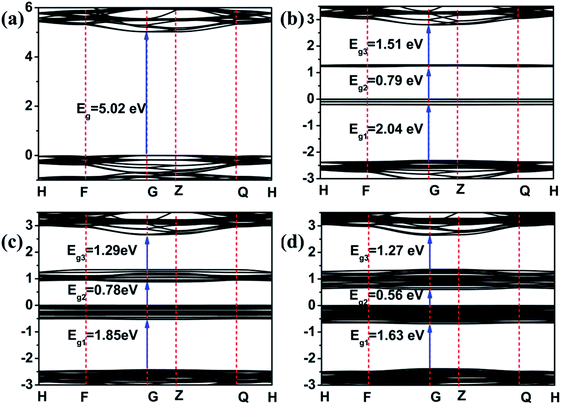 |
| Fig. 5 Band structure for dolomite supercell with 0 (a), 3 (b), 6 (c), and 9 (d) Fe2+-substitution. The Fermi level is set to 0. | |
The DOS calculation indicated that the states near the valence band maximum (VBM) are contributed by the hybrid between O 2p and Mg 3p orbitals, and the states near the conduction band maximum (CBM) mainly originate from C 2p and Ca 3d orbitals for the pristine dolomite (Fig. 6(a)). Two additional doping states appear in the forbidden band gap after 3 Fe2+ substitution, which is attributed to the hybrid between Fe 3d and Ca 3d orbitals (Fig. 6(b)). With the increase of Fe2+ contents, the two doping states become wider, thereby resulting in gradual decrease of the band gap.
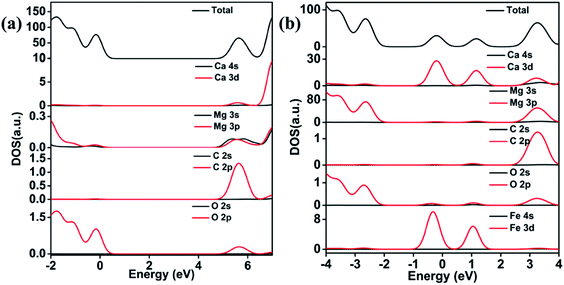 |
| Fig. 6 Density of state for pure (a) and 3 Fe2+-substituted (b) dolomite. The Fermi level is set to 0. | |
Overall, the theoretical calculation results indicate that the dolomite exhibits the characters of semiconductors after the substitution of Mg2+ by Fe2+, suggesting that dolomite could act as a semiconductor photocatalyst under the irradiation of solar light. To verify the role of O2 during the photocatalytic process, deoxygenation experiments were performed and the DP degradation was suppressed by N2 purging (Fig. 7). The generation of ROS in the dolomite system was related to the dissolved oxygen (DO) in solution. N2 purging causes a decrease of DO concentration, thus inhibiting the formation of reactive oxygen species.
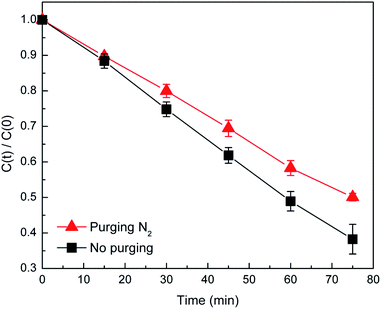 |
| Fig. 7 The photodegradation of DP in the presence of natural dolomite in the deoxygenated solution. | |
According to the theoretical calculations, the results from ESR analysis, and the deoxygenation experiments, we proposed a mechanism for the photocatalysis of dolomite. Under irradiation, photo-excited electrons and holes migrate to the surface of the dolomite (eqn (1)). Subsequently, further reactions according to eqn (2)–(6) yield ˙OH and O2−˙. As for 1O2, its generation might be due to the energy transfer between dolomite and DO.
|
Dolomite + hν → h+ + e−
| (1) |
|
H2O2 + O2−˙ → ˙OH + OH− + O2
| (6) |
3.4 Identification of degradation products and pathways
To obtain a further insight into the photolytic mechanism and the photochemical fate of the DP, the main degradation products of DP in the reaction were identified using HR LC-MS, respectively (Fig. S5†). As shown in Table 3, the photocatalytic process leads to the formation of m/z 183, m/z 272, and m/z 167, which were also detected in the previous studies.15,31,33
Table 3 Retention time, mass spectra, and structure of the identified photoproducts
m/z |
Retention time (min) |
Elemental composition |
Proposed structure |
167 |
7.1 |
C13H11 |
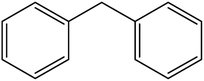 |
183 |
8.0 |
C13H11O2 |
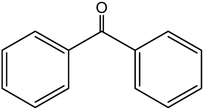 |
256 |
5.8 |
C17H22NO |
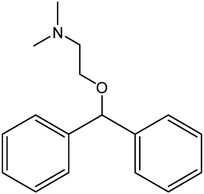 |
272 |
6.0 |
C17H22NO2 |
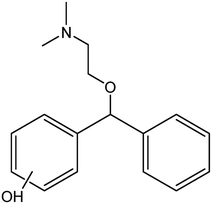 |
Among the observed products, m/z of 272 could be attributed to the hydroxylation of DP (m/z 256) (Fig. 8). Hydroxyl radical is a strong electrophilic species, and readily adds to aromatic rings. Thus, hydroxyl radical addition to the aromatic ring accompanied by dehydrogenation is a common reaction pathway for aromatic compounds.15 Considering the generation of ˙OH in the dolomite system, it is logical to expect the addition of ˙OH to the aromatic ring in DP molecules, leading to the formation of m/z 272. As for the products of m/z 183 and 167, their formation might result from the hydroxyl radical mediated oxidation of the alkylamine side chain (Fig. 8).32,33
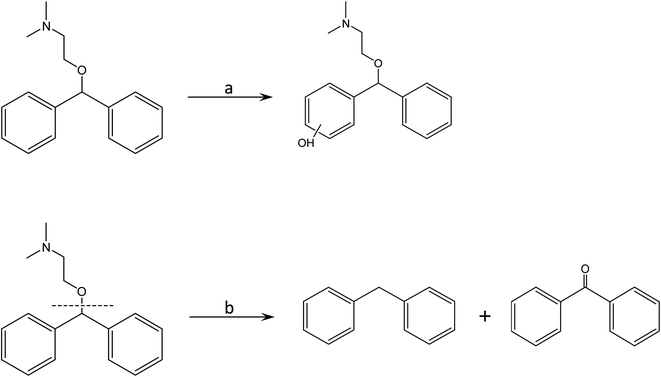 |
| Fig. 8 Proposed degradation pathways of DP in the presence of natural dolomite. | |
In summary, from the intermediates determined it appears that the oxidation process occurs mainly through the hydroxyl radicals. However, the results from the scavenger experiments indicated that the superoxide anions also play an important role. Thus, it seems that the superoxide anions generated were converted to hydroxyl radicals via further reaction, reinforcing the role of hydroxyl radicals.
4. Conclusions
For the first time, dolomite, an inexpensive and easily available mineral, was used for photocatalytic degradation of DP in aqueous solution. The results demonstrated that the presence of dolomite greatly promoted the degradation of DP, exhibiting significant photocatalytic activity. The ESR analysis and scavenger experiments demonstrated that 1O2, O2−˙, and ˙OH were produced in the dolomite system after irradiation, and contributed to the enhanced DP photodegradation. Based on the first-principles calculations, it was proposed that the process of dolomite photocatalysis mainly involved in two steps: first, the dolomite with semiconductor properties produces photo-generated electrons and holes under irradiation, which subsequently react to form reactive species. Second, the reactive species such as hydroxyl radicals caused the oxidation of DP. The product studies indicated, on the one hand, that the hydroxylation of aromatic rings and oxidation of the alkylamine side chain are the main degradation pathways of DP; while, on the other hand, the hydroxyl radicals are produced in the photocatalytic process and play an important role in DP degradation.
Conflicts of interest
There are no conflicts to declare.
Acknowledgements
This work was supported by the National Natural Science Foundation of China (Grant 41403083), the Key Project of Science and Technology of Hubei Provincial Department of Education (Grant D20141305) and Natural Science of Foundation of Hubei Province, China (Grant 2019CFB225).
References
- Q. Bu, B. Wang, J. Huang, S. Deng and H. Yu, Pharmaceuticals and personal care products in the aquatic environment in China: a review, J. Hazard. Mater., 2013, 262, 189–211 CrossRef CAS.
- L. Arpin-Pont, M. J. M. Bueno, E. Gomez and H. Fenet, Occurrence of PPCPs in the marine environment: a review, Environ. Sci. Pollut. Res., 2016, 23, 4978–4991 CrossRef.
- Y. Yang, Y. S. Ok, K. H. Kim, E. E. Kwon and Y. F. Tsang, Occurrences and removal of pharmaceuticals and personal care products (PPCPs) in drinking water and water/sewage treatment plants: a review, Sci. Total Environ., 2017, 596–597, 303–320 CrossRef CAS.
- P. K. Jjemba, Excretion and ecotoxicity of pharmaceutical and personal care products in the environment, Ecotoxicol. Environ. Saf., 2006, 63, 113–130 CrossRef CAS.
- S. A. Ortiz de Garciá, G. P. Pinto, P. A. García-Encina and R. Irusta-Mata, Ecotoxicity and environmental risk assessment of pharmaceuticals and personal care products in aquatic environments and wastewater treatment plants, Ecotoxicology, 2014, 23, 1517–1533 CrossRef.
- L. Cizmas, V. K. Sharma, C. M. Gray and T. J. McDonald, Pharmaceuticals and personal care products in waters: occurrence, toxicity, and risk, Environ. Chem. Lett., 2015, 13, 381–394 CrossRef CAS.
- J. P. Berninger, B. Du, K. A. Connors, S. A. Eytcheson, M. A. Kolkmeier, K. N. Prosser, T. W. Valenti Jr, C. K. Chambliss and B. W. Brooks, Effects of the antihistamine diphenhydramine on selected aquatic organisms, Environ. Toxicol. Chem., 2011, 30, 2065–2072 CrossRef CAS.
- B. Du, A. E. Price, W. C. Scott, L. A. Kristofco, A. J. Ranirez, C. K. Chambliss, J. C. Yederman and B. W. Brooks, Comparison of contaminants of emerging concern removal, discharge, and water quality hazards among centralized and on-site wastewater treatment system effluents receiving common wastewater influent, Sci. Total Environ., 2014, 466–467, 976–984 CrossRef CAS.
- P. E. Stackelberg, E. T. Furlong, M. T. Meyer, S. D. Zaugg, A. K. Henderson and D. B. Reissman, Persistence of pharmaceutical compounds and other organic wastewater contaminants in a conventional drinking-water-treatment plant, Sci. Total Environ., 2004, 329, 99–113 CrossRef CAS.
- F. Yuan, C. Hu, X. Hu, J. Qu and M. Yang, Degradation of selected pharmaceuticals in aqueous solution with UV and UV/H2O2, Water Res., 2009, 43, 1766–1774 CrossRef CAS.
- N. López, P. Marco, J. Giménez and S. Esplugas, Photocatalytic diphenhydramine degradation under different radiation sources: kinetic studies and energetic comparison, Appl. Catal., B, 2018, 220, 497–505 CrossRef.
- L. M. Pastrana-Martínez, N. Pereira, R. Lima, J. L. Faria, H. T. Gomes and A. M. T. Silva, Degradation of diphenhydramine by photo-Fenton using magnetically recoverable iron oxide nanoparticles as catalyst, Chem. Eng. J., 2015, 261, 45–52 CrossRef.
- L. M. Pastrana-Martínez, J. L. Faria, J. M. Doña-Rodríguez, C. Fernández-Rodríguez and A. M. T. Silva, Degradation of diphenhydramine pharmaceutical in aqueous solutions by using two highly active TiO2 photocatalysts: operating parameters and photocatalytic mechanism, Appl. Catal., B, 2012, 113–114, 221–227 CrossRef.
- S. Morales-Torres, L. M. Pastrana-Martínez, J. L. Figueiredo, J. L. Faria and A. M. T. Silva, Graphene oxide-P25 photocatalysts for degradation of diphenhydramine pharmaceutical and methyl orange dye, Appl. Surf. Sci., 2013, 275, 361–368 CrossRef CAS.
- D. Cui, A. M. Mebel, L. E. Arroyo-Mora, C. Zhao, A. D. Caprio and K. O′Shea, Fundamental study of the ultrasonic induced degradation of the popular antihistamine, diphenhydramine (DPH), Water Res., 2018, 144, 265–273 CrossRef CAS.
- A. Xu, W. Tu, S. Shen, Z. Lin, N. Gao and W. Zhong, BiVO4@MoS2 core–shell heterojunction with improved photocatalytic activity for discoloration of Rhodamine B, Appl. Surf. Sci., 2020, 528, 146949 CrossRef CAS.
- Z. Wang, B. Xiao, Z. Lin, S. Shen, A. Xu, Z. Du, Y. Chen and W. Zhong, In situ surface decoration of RuO2 nanoparticles by laser ablation for improved oxygen evolution reaction activity in both acid and alkali solutions, J. Energy Chem., 2021, 54, 510–518 CrossRef.
- W. Zhong, S. Shen, M. He, D. Wang, Z. Wang, Z. Lin, W. Tu and J. Yu, The pulsed laser-induced Schottky junction via in situ forming Cd clusters on CdS surfaces toward efficient visible light-driven photocatalytic hydrogen evolution, Appl. Catal., B, 2019, 258, 117967 CrossRef CAS.
- P. Shao, J. Tian, X. Duan, Y. Yang, W. Shi, X. Luo, F. Cui, S. Luo and S. Wang, Cobalt silicate hydroxide nanosheets in hierarchical hollow architecture with maximized cobalt active site for catalytic oxidation, Chem. Eng. J., 2019, 359, 79–87 CrossRef CAS.
- P. Shao, J. Pei, H. Tang, S. Yu, L. Yang, H. Shi, K. Yu, K. Zhang and X. Luo, Defect-rich porous carbon with anti-interference capability for adsorption of bisphenol A via long-range hydrophobic interaction synergized with short-range dispersion force, J. Hazard. Mater., 2021, 403, 123705 CrossRef CAS.
- R. J. Reeder and W. A. Dollase, Structural variation in the dolomite-ankerite solid-solution series: an X-ray, Mössbauer, and TEM study, Am. Mineral., 1989, 74, 1159–1167 CAS.
- O. Sivrikaya, A study on the physicochemical and thermal characterisation of dolomite and limestone samples for use in ironmaking and steelmaking, Ironmaking Steelmaking, 2018, 45, 764–772 CrossRef CAS.
- P. Shao, S. Yu, X. Duan, L. Yang, H. Shi, L. Ding, J. Tian, L. Yang, X. Luo and S. Wang, Potential difference driving electron transfer via defective carbon nanotubes toward selective oxidation of organic micropollutants, Environ. Sci. Technol., 2020, 54, 8464–8472 CrossRef CAS.
- P. Shao, X. Duan, J. Xu, J. Tian, W. Shi, S. Gao, M. Xu, F. Cui and S. Wang, Heterogenous activation of peroxymonosulfate by amorphous boron for degradation of bisphenol S, J. Hazard. Mater., 2017, 322, 532–539 CrossRef CAS.
- P. Shao, J. Tian, F. Yang, X. Duan, S. Gao, W. Shi, X. Luo, F. Cui, S. Luo and S. Wang, Identification and regulation of active sites on nanodiamonds: establishing a highly efficient catalytic system for oxidation of organic contaminants, Adv. Funct. Mater., 2018, 28, 1705295 CrossRef.
- G. Kresse and J. Furthmüller, Efficiency of ab initio total energy calculations for metals and semiconductors using a plane-wave basis set, Comput. Mater. Sci., 1996, 6, 15–50 CrossRef CAS.
- B. Hammer, L. B. Hansen and J. K. Norskov, Improved adsorption energetics within density-functional theory using revised Perdew-Burke-Ernzerhof functionals, Phys. Rev. B: Condens. Matter Mater. Phys., 1999, 59, 7413–7421 CrossRef.
- W. Zhang and Y. Xiao, Mechanism of electrocatalytically active precious Metal (Ni, Pd, Pt, and Ru) complexes in the graphene basal plane for ORR applications in novel fuel cells, Energy Fuels, 2020, 34, 2425–2434 CrossRef CAS.
- Q. Wang, Z. Q. Liu, D. M. Liu, W. Wang, Z. W. Zhao, F. Y. Cui and G. B. Li, Oxygen vacancy-rich ultrathin sulfur-doped bismuth oxybromide nanosheet as a highly efficient visible-light responsive photocatalyst for environmental remediation, Chem. Eng. J., 2019, 360, 838–847 CrossRef CAS.
- J. Warren, Dolomite: occurrence, evolution and economically important associations, Earth-Sci. Rev., 2000, 52, 1–81 CrossRef CAS.
- Y. Chen, C. Hu, X. Hu and J. Qu, Indirect photodegradation of amine drugs in aqueous solution under simulated sunlight, Environ. Sci. Technol., 2009, 43, 2760–2765 CrossRef CAS.
- F. M. Hossain, G. E. Murch, I. V. Belova and B. D. Turner, Electronic, optical and bonding properties of CaCO3 calcite, Solid State Commun., 2009, 149, 1201–1203 CrossRef CAS.
- N. López, S. Plaza, A. Afkhami, P. Marco, J. Giménez and S. Esplugas, Treatment of diphenhydramine with different AOPs including photo-Fenton at circumneutral pH, Chem. Eng. J., 2017, 318, 112–120 CrossRef.
Footnote |
† Electronic supplementary information (ESI) available. See DOI: 10.1039/d0ra07533g |
|
This journal is © The Royal Society of Chemistry 2020 |
Click here to see how this site uses Cookies. View our privacy policy here.