DOI:
10.1039/D0RA07466G
(Paper)
RSC Adv., 2020,
10, 38416-38423
Semiconductor ZnO based photosensitizer core–shell upconversion nanoparticle heterojunction for photodynamic therapy
Received
31st August 2020
, Accepted 12th October 2020
First published on 19th October 2020
Abstract
Photodynamic therapy (PDT) as a noninvasive technique is widely used to treat cancer diseases due to its low side effects. PDT based on upconversion nanoparticles (UCNPs) improved tissue penetration and photo-stability. However, traditional photosensitizers and UCNPs were difficult to incorporate, which limited the circulation of the UCNPs in blood and decreased the PDT effect. Herein, we designed NaErF4@ZnO UCNPs for potential application in thyroid tumor cell PDT. With ZnO coated on NaErF4, the blue (415 nm), green (525 nm/545 nm) and red (661 nm) upconversion luminescence enhanced compared with that of NaErF4 core nanoparticles. Particularly, the generation of UV upconversion emission by NaErF4 sensitized ZnO, which catalyzed H2O and O2 to produce ROS reactive oxygen species (ROS) to induce papillary thyroid carcinoma (PTC) cell lines BHP 5-16. With 1000 μg mL−1 of NaErF4@ZnO UCNPs, the viability of BHP 5-16 cells decreased to about 41% as measured by CCK8 assay with 980 nm NIR irradiation. Moreover, it was confirmed that NaErF4@ZnO UCNPs had low toxicity for BHP 5-16 cells. All these results indicated that NaErF4@ZnO upconversion nanoparticles were an excellent platform for PDT treatment.
Introduction
Photodynamic therapy (PDT) is a noninvasive treatment technique, which utilizes photosensitizers to produce reactive oxygen species (ROS).1–3 ROS include hydroxyl radicals (˙OH), singlet oxygen (1O2) and hydrogen peroxide (H2O2), which can cause oxidative damage to cells.4–6 Compared with normal cells, the ROS levels had an important influence on cancer cells. The PDT technique can treat cancer and other malignant diseases.7–16 However, the further development of PDT was limited to photosensitizers. Most of the photosensitizers were sensitized by ultraviolet (UV) and visible (vis) light, which decreased tissue penetration of the PDT treatment.17–19
Rare earth-doped upconversion nanoparticles (UCNPs) had attracted great interest recently owing to their unique photo-physical capabilities.20–22 Upconversion luminescence (UCL) was anti-Stokes optical process, which can convert near infrared (NIR) light into UV and vis light.23–27 The wavelength of NIR light ranged from 700 nm to 1500 nm, which located in biological spectroscopy window.28–30 Therefore, UCNPs had great potential application in PDT treatment due to their high tissue penetration, low fluorescence background, good photo-stability and high signal-to-noise ratio.31,32 However, the tradition PDT treatment based on UCNPs needed to combine photosensitizers. The photosensitizers loaded on as-synthesized UCNPs, which limited to the circulation of the UCNPs in blood and decreased the PDT effect.33,34 In addition, most of photosensitizers were organic compound and purchases, increasing the PDT treatment costs and toxicity.
Zinc oxide (ZnO) provided a new way to improve this problem owing to its high chemical stability, high thermal stability and low toxicity.35–37 ZnO nanoparticle as a semiconductor had wide band gap about 3.37 eV, which matched with emission peaks range from 330 nm to 400 nm, and UCNPs can radiated this wavelength of UV.38,39 ZnO nanoparticle was a promising photosensitizer, which can be easily embedded into UCNPs.
In this work, we synthesized NaErF4@ZnO UCNPs for PDT application. NaErF4 excited UV upconversion luminescence and sensitized the ZnO to generate the ROS. After ZnO coated on NaErF4, the intensities of upconversion emission enhanced compared with that of NaErF4 nanoparticles. ROS of NaErF4@ZnO UCNPs produced with 980 nm NIR light irradiation, and the NIR irradiation increased the deep of tissue penetration. The evaluation of cell viability based on CCK8 assay method had an outstanding effect on photodynamic therapy for thyroid tumor cells. In addition, the NaErF4@ZnO UCNPs had low toxicity for BHP 5-16 cells. These results indicated that as-prepared NaErF4@ZnO UCNPs were excellent platform for PDT treatment.
Result and discussion
Fig. 1 illustrated 980 nm NIR laser-activated, mitochondria-targeted upconversion NaErF4@ZnO nanoparticles for amplified photodynamic therapy (PDT). We designed ZnO coated NaErF4 upconversion nanoparticles. NaErF4 can be excited the UV and visible upconversion emission via the excitation at 980 nm NIR light. These UV and visible light from NaErF4 can be used by ZnO. The upconverted UV light triggered the photogenerated electrons in the valence band of the ZnO to the conduction band, thus resulting in the formation of photoinduced electron–hole (e−–h+) pair, which catalyzed H2O and O2 produce ROS and induced cancer cell apoptosis. The NIR excitation light (980 nm) had high tissue penetration, which leaded to amplified therapeutic efficacy.
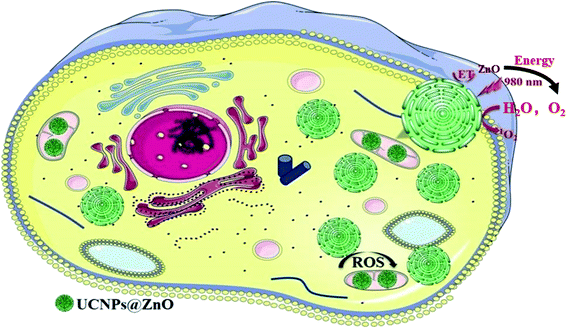 |
| Fig. 1 Schematic illustration of photodynamic therapy mechanism of NaErF4@ZnO upconversion nanoparticles in producing the ROS under the excitation at 980 nm. ET was the energy transfer process. | |
Fig. 2 performed X-ray diffraction patterns (XRD) of the as-synthesized NaErF4 coating ZnO (NaErF4@ZnO) upconversion nanoparticles. The XRD diffraction peaks of NaErF4@ZnO can be assigned to two crystalline phases of NaErF4 and ZnO. The majority of α phase of NaErF4 and hexagonal phased of ZnO were presented. Crystal surface (100), (002), (101), (102), (110) and (103) of samples were mainly attributed to the ZnO. Crystal surface (111), (200), (220), (311), and (400) of samples were corresponded to α-NaErF4. These results indicated that NaErF4 and ZnO upconversion nanoparticles compound was obtained.
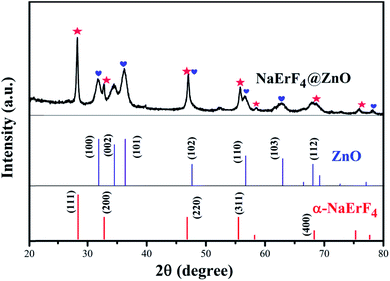 |
| Fig. 2 X-ray diffraction patterns of NaErF4@ZnO upconversion nanoparticles. The diffraction peaks of α-phase NaErF4 and ZnO were marked by red and blue labels, respectively. | |
To further reveal the successful preparation of NaErF4@ZnO upconversion nanoparticles, transmission electron microscopy (TEM) images of NaErF4 and NaErF4@ZnO was showed in Fig. 3a and b. Without coating ZnO, the NaErF4 was quadrilateral-like nanostructures, and the size of samples was about 17.1 nm. As illustrated in Fig. 3b and c, the size of nanoparticles increased to about 42 nm, and the quadrilateral-like nanostructure remained. High resolution TEM (HTEM) image (Fig. 3d) of a single nanoparticle taken from Fig. 3c presented the measured interplanar spacing of 0.28 nm and 0.31 nm, corresponding to the (100) crystal plane to hexagonal phase ZnO and the (111) crystal plane to α phase NaErF4, respectively. Scanning Electron Microscopy (SEM) of NaErF4@ZnO upconversion nanoparticles performed a tetrahedral structure in Fig. 3e. These results suggested that ZnO was coated on the NaErF4 surface, forming NaErF4@ZnO. In addition, energy dispersive X-ray spectrometer (EDS) mapping taken from Fig. 3e was analyzed in Fig. 3f. The EDS mapping showed the presence of Zn, O, Er and F elements, further confirming the preparation of NaErF4@ZnO upconversion nanoparticles. All these observations verified that ZnO was coated successfully on the NaErF4 particles.
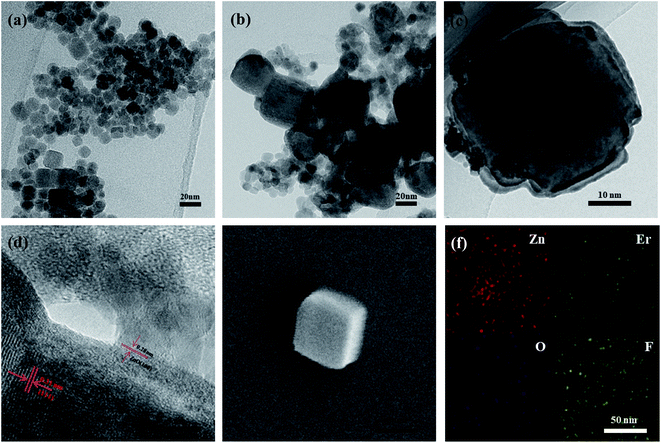 |
| Fig. 3 (a) TEM image of NaErF4 upconversion nanoparticles. (b) TEM image of NaErF4@ZnO upconversion nanoparticles. (c) TEM image of NaErF4@ZnO upconversion single particle. (d) HTEM image taken from single particle in (c) of NaErF4@ZnO. (e) SEM image of NaErF4@ZnO upconversion single particle. (f) EDS mapping of NaErF4@ZnO upconversion nanoparticles. The brightness of the image represented the concentration of the element. | |
In order to investigate the luminescent properties of as-prepared NaErF4@ZnO nanoparticles, the upconversion luminescent spectra of NaErF4 and NaErF4@ZnO was compared in Fig. 4a. It can be seen that the all upconversion emission intensity was increased by coating ZnO particles. While in NaErF4@ZnO system, blue, green and red upconversion luminescence (UCL) of samples can be achieved and UCL intensities were enhanced by 27, 7 and 10 times compared to that of NaErF4 (Fig. 4b), respectively. As demonstrated, upconversion spectrum showed emission bands centered at 525 nm (green UCL), 545 nm (green UCL) and 661 nm (red UCL), which can be assigned to the 2H11/2 → 4I15/2 transition, 4S3/2 → 4I15/2 transition and 4F9/2 → 4I15/2 transition of Er3+ ions (Fig. 4c), respectively. In addition, the UCL emission at 350∼400 nm was observed, which can excited the ZnO particle, sensitized reactive oxygen species generation from oxygen for photodynamic therapy (PDT). The blue UCL at 415 nm originated from Vo2+ → VZn energy level defect transition of ZnO, indicating ZnO was sensitized by UV light. This result confirmed that ZnO was coated on NaErF4 in as-prepared upconversion nanoparticles. Since the production of singlet oxygen was based on 980 nm excitation NIR light, 980 nm NIR illumination improved the tissue penetration and enhanced the PDT efficiency. As performed in Fig. 4d, the ROS generation of NaErF4@ZnO upconversion nanoparticles under 980 nm NIR light was measured via the absorbance of 1,3-diphenyliso-benzofuran (DPBF) at 488 nm. In Fig. 4d, the DPBF absorption intensity of NaErF4@ZnO upconversion nanoparticles with 980 nm irradiation significantly decreased with increase time from 0 min to 30 min, suggesting that a more efficient ROS generation. In constant, the DPBF absorption intensity of only 980 nm irradiation or NaErF4@ZnO nanoparticles had no obvious changing, indicating that only NIR light or materials cannot be produced ROS. This result can further verify that NaErF4@ZnO upconversion nanoparticles can be sensitized to generate ROS under 980 nm excitation, which had great potential in the application of photodynamic therapy.
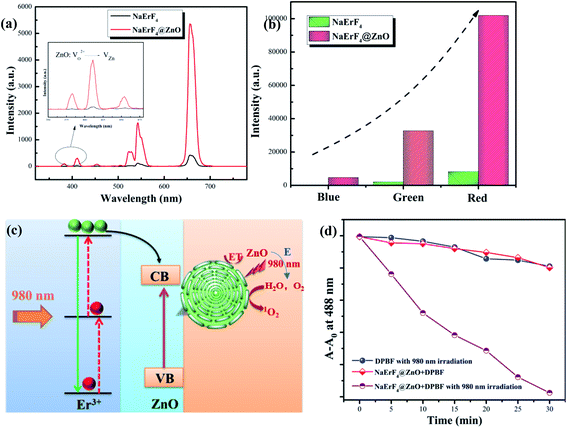 |
| Fig. 4 (a) The upconversion luminescent spectra of NaErF4 and NaErF4@ZnO upconversion nanoparticles, the inset was the upconversion luminescent spectra range from 350 nm to 475 nm bands of samples. (b) The upconversion luminescent intensities of NaErF4 and NaErF4@ZnO nanoparticles. (c) Schematic illustration of upconversion energy transfer mechanism of NaErF4@ZnO. ET was energy transfer and E was energy. (d) DPBF absorbance of NaErF4@ZnO without 980 nm irradiation and 980 nm irradiation without NaErF4@ZnO. DPBF absorbance of NaErF4@ZnO with 980 nm irradiation with increasing of time. A and A0 was DPBF absorption intensity and absorption intensity of control group, respectively. | |
The NaErF4@ZnO upconversion nanoparticles (UCNPs) had potential application in PDT due to their high ROS generation with NIR irradiation. Moreover, bio-toxicity had an important influence on the development of UCNPs for biological applications. In order to investigate the cytotoxicity of NaErF4@ZnO upconversion nanoparticles (UCNPs) in vitro, the toxicity of NaErF4@ZnO UCNPs without 980 nm NIR irradiation to cells was measured BHP 5-16 cells with CCK8 method. A CCK8 assay with BHP 5-16 cells was used to study the cytotoxicity of Yb/Tm/GZO@SiO2 nanoparticles (Fig. 5a). BHP 5-16 cells viability can be followed eqn (1).40
|
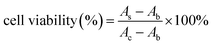 | (1) |
where
As and
Ab were the absorbance of test cells with NaErF
4@ZnO UCNPs and the absorbance of control cells without NaErF
4@ZnO UCNPs, respectively.
Ac represented the absorbance of blank samples containing culture medium without cells and NaErF
4@ZnO UCNPs. As illustrated in
Fig. 5a, the viability of cells was evaluated at three time points (12 h, 24 h and 48 h) after incubating with 400 μg mL
−1, 600 μg mL
−1, 800 μg mL
−1 and 1000 μg mL
−1 NaErF
4@ZnO UCNPs. Although the concentration of NaErF
4@ZnO UCNPs was as high as 1000 μg mL
−1 for 48 h, the cell viabilities were greater than 94.88%, indicating the NaErF
4@ZnO UCNPs had a low toxicity to BHP 5-16 cells. These results demonstrated that NaErF
4@ZnO UCNPs had the low cytotoxicity, allowing for NaErF
4@ZnO UCNPs as PDT application.
In vitro PDT therapeutic effects of NaErF
4@ZnO UCNPs were evaluated using the BHP 5-16 cells upon 980 nm NIR irradiation at different concentrations of UCNPs. BHP 5-16 cells were treated with UCNPs for 12h. BHP 5-16 cells were irradiated at a 980 nm laser with 0.6 W cm
−2 for 30 min (the cell radiated at 2 min intervals), and the cell viability was determined with a CCK8 method. As shown in
Fig. 5b, the cell viabilities of only 980 nm irradiation without NaErF
4@ZnO UCNPs were about 91%, 96%, 89% and 90% at 400 μg mL
−1, 600 μg mL
−1, 800 μg mL
−1 and 1000 μg mL
−1 concentration of NaErF
4@ZnO UCNPs, respectively. It can be seen that 980 nm irradiation without NaErF
4@ZnO UCNPs had no significant impact on cell growth. The cell viabilities of only NaErF
4@ZnO UCNPs without 980 nm irradiation were about 98%, 95%, 92% and 89% at 400 μg mL
−1, 600 μg mL
−1, 800 μg mL
−1 and 1000 μg mL
−1 concentration of NaErF
4@ZnO UCNPs, respectively. This result confirmed the cell viability did not change too much for NaErF
4@ZnO UCNPs, despite the existence of a high concentration of 1000 μg mL
−1. Apparently, the cell viabilities were about 60%, 57%, 44% and 41% at increase concentration of NaErF
4@ZnO UCNPs from 400 μg mL
−1 to 1000 μg mL
−1 with NIR 980 nm laser irradiation. With increasing of concentration of NaErF
4@ZnO UCNPs, BHP 5-16 cells viability gradually decreased under 980 nm excitation. It was worth noting that only NaErF
4@ZnO UCNPs with 980 nm irradiation simultaneous existed, the cell survival rate can be significantly decreased, which further verified that NaErF
4@ZnO UCNPs can generated ROS with 980 nm laser irradiation. This indicated that NaErF
4@ZnO UCNPs had a good PDT effect on BHP 5-16 cells.
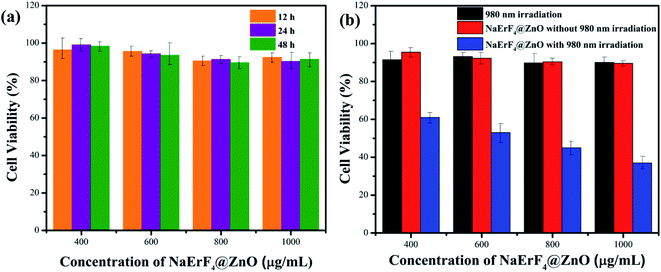 |
| Fig. 5 (a) CCK8 assay of the BHP-5-16 cells viability after incubating with different changed NaErF4@ZnO UCNPs concentrations ranging from 400 to 1000 μg mL−1 for 12 h, 24 h and 48 h. (each group n = 4) (b) CCK8 assay of NaErF4@ZnO UCNPs with different concentrations with and without 980 nm NIR irradiation (0.6 W cm−2, each group n = 4). | |
To further explore the PDT effect of NaErF4@ZnO UCNPs in BHP 5-16 cells in vitro, the production of ROS was measured though DCFH-DA probe. If there was ROS, it would show obvious fluorescence green images by DCFH-DA probe. Fig. 6a performed that control group had no markedly green light image, indicating without ROS expression. As shown in Fig. 6b and c, the images had no green fluorescence and cell morphology was intact structure at the 980 nm irradiation without NaErF4@ZnO UCNPs group and NaErF4@ZnO UCNPs without 980 nm irradiation at concentration of 800 μg mL−1. These results indicated that NaErF4@ZnO UCNPs and 980 nm irradiation had no damage the cell and had no produce ROS. When concentration of NaErF4@ZnO was 400 μg mL−1 with 980 nm irradiation, the image presented green light expression and BHP 5-16 cells had some damage (Fig. 6d). This suggested that ROS produced at 400 μg mL−1 of NaErF4@ZnO with 980 nm irradiation. The brightness of green light enhanced and most of cells had died with increase NaErF4@ZnO concentration from 600 μg mL−1 to 800 μg mL−1 with 980 nm irradiation (Fig. 6e and f), which consistent with ROS level of NaErF4@ZnO UCNPs increasing with rising of concentrations. It was further indicated that NaErF4@ZnO UCNPs had an excellent application in photodynamic therapy.
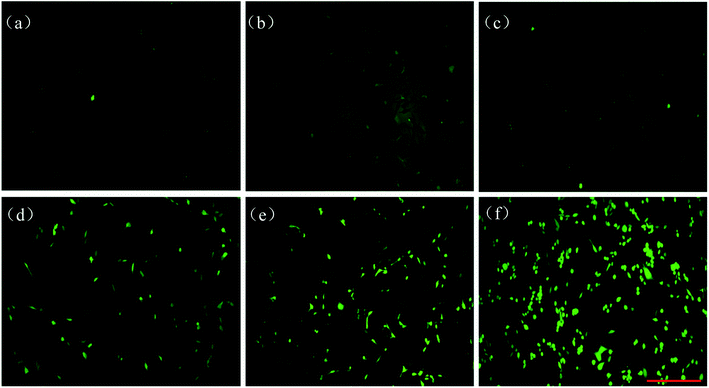 |
| Fig. 6 The production of ROS measured by DCFH-DA probe. (Image scale in a–f: 400 μm) (a) BHP 5-16 cells image of control group, (b) the 980 nm irradiation without NaErF4@ZnO UCNPs group, (c) NaErF4@ZnO UCNPs without 980 nm irradiation group at concentration of 800 μg mL−1, (d) NaErF4@ZnO UCNPs with 980 nm irradiation group at concentration of 400 μg mL−1, (e) NaErF4@ZnO UCNPs with 980 nm irradiation group at concentration of 600 μg mL−1 and (f) NaErF4@ZnO UCNPs with 980 nm irradiation group at concentration of 800 μg mL−1. | |
Conclusion
In summary, a novel NaErF4@ZnO UCNPs was synthesized. The UCL emission of NaErF4 was used to sensitize ZnO. The ZnO as a photosensitizer can produce the ROS. The unique combination of NaErF4 and ZnO UCNPs can be used for PDT of thyroid cancer cell. ROS generation of NaErF4@ZnO upconversion nanoparticles with 980 nm NIR light was measured via the absorbance of DPBF, suggesting sensitization of ROS production with NaErF4@ZnO. Meanwhile, the toxicity of BHP 5-16 cells was evaluated by CCK8 assay. The viability of BHP 5-16 cells has no damage at 1000 μg mL−1 of NaErF4@ZnO for 48 h, indicating this nanoparticle had low toxic for BHP 5-16 cells. The results of CCK8 assay performed that 60%∼41% cell viability can be obtained for NaErF4@ZnO UCNPs at the concentration from 400 μg mL−1 to 1000 μg mL−1 with 30 min 980 nm NIR irradiation. The image based on dihydroethidium staining presented the highest green light with 800 μg mL−1 of NaErF4@ZnO compared with that of 400 μg mL−1 and 600 μg mL−1, which consistent with the dosage concentrations required for PDT of papillary thyroid cancer.
Experiment
Synthesis of NaErF4 core upconversion nanoparticles
The NaOH of 0.6 g was dissolved in 5 mL deionized water, and added oleic acid (10 mL) with ultrasound for 20 min. Er(NO3)3·5H2O (1 mmol), Yb(NO3)3·5H2O (20 mol%) and NaF (8 mmol) were dissolved while stirring for 2 h at 25 °C. After this procedure, the resulting solution was transferred to the reaction kettle (30 mL) and reacted at 140 °C for 12 h. The obtained NaErF4 sample was cooled down to room temperature, and cleaned by centrifugation with deionized water and ethanol. The core upconversion nanoparticles were achieved.
Synthesis of NaErF4@ZnO upconversion nanoparticles
As-synthesized NaErF4 nanoparticles was dispersed in 30 mL ethanol and 20 mL deionized water with ultrasonic for 30 min of water and solution and ultrasonic wave for 30 min. CTAB (0.3 mmol) was added into this suspension with ultrasonic for 10 min. And then ascorbic acid (0.2 mmol), hexamethylenetetramine (1 mmol) and Zn(NO3)2·6H2O (1 mmol) were added into the previous mixture with magnetic stirring for 1 h in room temperature, and heated up to 85 °C for 10 h. The samples were cleaned by centrifugation with deionized water and ethanol, and dried in an oven for 24 h. The obtained samples were annealed for 2 h at 350 °C in the muffle furnace. The NaErF4@ZnO core/shell upconversion nanoparticles (UCNPs) was obtained.
Characterization of the NaErF4@ZnO (UCNPs)
TEM images was obtained with JEM-2100. Elemental analysis was achieved with an energy-dispersive X-ray (EDX) by TEM. The X-ray diffraction (XRD) of the NaErF4 and NaErF4@ZnO was collected by a diffractometer with Cu Kα that worked at 40.0 kV and 30.0 mA. The upconversion fluorescence spectrometer was measured with 980 nm laser excitation.
Cell culture
The BHP 5-16 cells were cultured RPMI 1640 (Hyclone, China) supplemented with 10% fetal bovine serum (Gibico, USA) and 1% penicillin–streptomycin (Solarbio, China). Cells were maintained in a humidified incubator (Thermo Forma, USA) containing 5% CO2 at 37 °C, and all the experiments were performed in a clean atmosphere.
In vitro cytotoxicity evaluation
A Cell Counting Kit-8 (CCK-8) (Beyotime Institute of Biotechnology) was used for the evaluation of cell cytotoxicity. A total of 5000 cells were seeded into 96 well plates, which were cultured in AIM medium and treated with different concentrations of NaErF4@ZnO UCNPs (400 μg mL−1, 600 μg mL−1, 800 μg mL−1, 1000 μg mL−1) in a humidified atmosphere at 37 °C with 5% CO2 for 12 h, 24 h and 48 h, respectively. CCK-8 (10 μL) was added into each well and incubated for 1.5 h at 37 °C. The absorbance was measured using the iMark Microplate Reader at a wavelength of 450 nm (BIO-RAD Instruments, USA).
In vitro ROS generation assay
The Reactive Oxygen Species Assay Kit (Beyotime Institute of Biotechnology) was used for the detection of intracellular ROS. BHP 5-16 (2 × 107) cells were seeded into 24 well plates, which were cultured in 1640 medium and treated with different concentrations of NaErF4@ZnO UCNPs (400 μg mL−1, 600 μg mL−1, 800 μg mL−1). The medium was discarded and added fresh 1640 medium after 12 h, then the samples were irradiated at a 980 nm laser with 0.6 W cm−2 for 30 min, 500 μL DCFH-DA reagent was added into each well and incubated for 20 min at 37 °C. The fluorescence images were achieved with a fluorescence microscope at a wavelength of 488 nm.
Conflicts of interest
There are no conflicts to declare.
Acknowledgements
This work was financially supported by the Science & Technology Development Fund of Tianjin Education Commission for Higher Education (No. 2018KJ069) and Startup Funding of Scientific Research, Tianjin Medical University Metabolic Diseases Hospital and Tianjin Institute of Endocrinology (No. 2017DX07).
References
- C. A. Robertson, D. H. Evans and H. Abrahamse, Photodynamic therapy (PDT): a short review on cellular mechanisms and cancer research applications for PDT, J. Photochem. Photobiol., B, 2009, 96, 1–8 CrossRef CAS.
- J. Jin, Y. Zhu, Z. Zhang and W. Zhang, Enhancing the efficacy of photodynamic therapy (PDT) through a porphyrin/POSS alternating copolymer, Angew. Chem., 2018, 57, 16354–16358 CrossRef CAS.
- K. Wang, Z. Zhang, L. Lin, J. Chen, K. Hao, H. Tian and X. Chen, Covalent organic nanosheets integrated heterojunction with two strategies to overcome hypoxic-tumor photodynamic therapy, Chem. Mater., 2019, 31, 3313–3323 CrossRef CAS.
- P. Ray, B. W. Huang and Y. Tsuji, Reactive oxygen species (ROS) homeostasis and redox regulation in cellular signaling, Cell. Signalling, 2012, 24, 981–990 CrossRef CAS.
- R. D. Guzy, M. M. Mack and P. T. Schumacker, Mitochondrial complex III is required for hypoxia-induced ROS production and gene transcription in yeast, Antioxid. Redox Signaling, 2007, 9, 1317–1328 CrossRef CAS.
- K. K. Griendling and G. FitzGerald, Oxidative stress and cardiovascular injury part I: basic mechanisms and in vivo monitoring of ROS, Circulation, 2003, 108, 1912–1916 CrossRef.
- J. C. Kennedy, R. H. Pottier and D. C. Pross, Photodynamic therapy with endogenous protoporphyrin IX: basic principles and present clinical experience, J. Photochem. Photobiol., B, 1990, 6, 143–148 CrossRef CAS.
- R. Bonnett, Photosensitizers of the porphyrin and phthalocyanine series for photodynamic therapy, Cheminform, 1995, 26, 19–33 Search PubMed.
- D. Kessel, Y. Luo, Y. Deng and C. K. Chang, The role of subcellular localization in initiation of apoptosis by photodynamic therapy, Photochem. Photobiol., 2010, 65, 422–426 CrossRef.
- D. Bechet, P. Couleaud, F. Céline, M. Viriot, F. Guillemin and M. Barberi, Nanoparticles as vehicles for delivery of photodynamic therapy agents, Trends Biotechnol., 2008, 26, 612–621 CrossRef CAS.
- S. M. Halpern, A. V. Anstey, R. S. Dawe, B. L. Diffey, P. M. Farr, J. Ferguson, J. L. Hawk, S. Ibbotson, J. M. McGregor, G. M. Murphy, S. E. Thomas and L. E. Rhodes, Guidelines for topical photodynamic therapy: report of a workshop of the British photodermatology group, Br. J. Dermatol., 2015, 142, 22–31 CrossRef.
- A. P. Castano, P. Mroz and M. R. Hamblin, Photodynamic therapy and anti-tumour immunity, Nat. Rev. Cancer, 2006, 6, 535–545 CrossRef CAS.
- C. Chen, X. Ni, S. Jia, Y. Liang, X. Wu, D. Kong and D. Ding, Massively evoking immunogenic cell death by focused mitochondrial oxidative stress using an AIE luminogen with a twisted molecular structure, Adv. Mater., 2019, 31, 1904914 CrossRef CAS.
- X. Ni, X. Zhang, X. Duan, H. Zheng, X. Xue and D. Ding, Near-Infrared afterglow luminescent aggregation-induced emission dots with ultrahigh tumor-to-liver signal ratio for promoted image-guided cancer surgery, Nano Lett., 2019, 19, 318–330 CrossRef CAS.
- C. Chen, H. Ou, R. Liu and D. Ding, Regulating the photophysical property of organic/polymer optical agents for promoted cancer phototheranostics, Adv. Mater., 2020, 32, 1806331 CrossRef CAS.
- C. Chen, X. Ni, H.-W. Tian, Q. Liu, D.-S. Guo and D. Ding, Calixarene-based supramolecular AIE dots with highly inhibited nonradiative decay and intersystem crossing for ultrasensitive fluorescence image-guided cancer surgery, Angew. Chem., Int. Ed., 2020, 59, 10008 CrossRef CAS.
- H. Fang, S. Xu and B. Liu, Photosensitizers with aggregation-induced emission: materials and biomedical applications, Adv. Mater., 2018, 30, e1801350 CrossRef.
- R. R. Allison, G. H. Downie, R. Cuenca, X. Hu, J. Carter and C. Sibata, Photosensitizers in clinical PDT, Photodiagn. Photodyn. Ther., 2004, 1, 27–42 CrossRef CAS.
- J. Berlanda, T. Kiesslich, V. Engelhardt, B. Krammer and K. Plaetzer, Comparative in vitro study on the characteristics of different photosensitizers employed in PDT, J. Photochem. Photobiol., B, 2010, 99, 173–180 CrossRef.
- G. Chen, H. Qiu, P. N. Prasad and X. Chen, Upconversion nanoparticles: design, nanochemistry, and applications in theranostics, Chem. Rev., 2014, 114, 5161–5214 CrossRef CAS.
- C. Wang, H. Tao, L. Cheng and Z. Liu, Near-infrared light induced in vivo photodynamic therapy of cancer based on upconversion nanoparticles, Biomaterials, 2011, 32, 6145–6154 CrossRef CAS.
- F. Wang, J. Wang and X. Liu, Direct evidence of a surface quenching effect on size-dependent luminescence of upconversion nanoparticles, Angew. Chem., 2010, 122, 7618–7622 CrossRef.
- L. Xiong, Z. Chen, Q. Tian, T. Cao, C. Xu and F. Li, High contrast upconversion luminescence targeted imaging in vivo using peptide-labeled nanophosphors, Anal. Chem., 2009, 81, 8687–8694 CrossRef CAS.
- J. Silver, M. I. Martinez-Rubio, T. G. Ireland, R. George and R. Withnall, Effect of particle morphology and crystallite size on the upconversion luminescence properties of erbium and ytterbium co-doped yttrium oxide phosphors, Cheminform, 2001, 32, 948–953 Search PubMed.
- F. Zhang, Y. Wan, T. Yu, F. Zhang, Y. Shi, S. Xie, Y. Li, L. Xu, B. Tu and D. Zhao, Uniform nanostructured arrays of sodium rare-earth fluorides for highly efficient multicolor upconversion luminescence, Angew. Chem., 2007, 119, 8122–8125 CrossRef.
- H. Song, B. Sun, T. Wang, S. Lu, L. Yang, B. Chen, X. Wang and X. Kong, Three-photon upconversion luminescence phenomenon for the green levels in Er3+/Yb3+ codoped cubic nanocrystalline yttria, Solid State Commun., 2004, 132, 409–413 CrossRef CAS.
- J. Wang, R. Li, Z. Zhang, W. Sun, R. Xu, Y. Xie, Z. Xing and X. Zhang, Efficient photocatalytic degradation of organic dyes over titanium dioxide coating upconversion luminescence agent under visible and sunlight irradiation, Appl. Catal., A, 2008, 334, 227–233 CrossRef CAS.
- I. P. Yong, H. N. Sang and J. H. Kim, Comparative Study of Upconverting Nanoparticles with Various Crystal Structures, Core/Shell Structures, and Surface Characteristics, J. Phys. Chem. C, 2013, 117, 2239–2244 CrossRef.
- Z. Yi, X. Li, Z. Xue, X. Liang, W. Lu, H. Peng, H. Liu, S. Zeng and J. Hao, Tumor detection: Remarkable NIR enhancement of multifunctional nanoprobes for in vivo trimodal bioimaging and upconversion optical/T2-weighted MRI-guided small tumor diagnosis, Adv. Funct. Mater., 2015, 25, 7102 CrossRef CAS.
- Z. Xue, Z. Yi, X. Li, Y. Li, M. Jiang, H. Liu and S. Zeng, Upconversion optical/magnetic resonance imaging-guided small tumor detection and in vivo tri-modal bioimaging based on high-performance luminescent nanorods, Biomaterials, 2017, 115, 90–103 CrossRef CAS.
- D. Song, S. Chi, X. Li, C. Wang, Z. Li and Z. Liu, Upconversion system with quantum dots as sensitizer: Improved photoluminescence and PDT efficiency, ACS Appl. Mater. Interfaces, 2019, 11, 41100–41108 CrossRef CAS.
- C. Wang, L. Cheng and Z. Liu, Upconversion nanoparticles for photodynamic therapy and other cancer therapeutics, Theranostics, 2013, 3, 317–330 CrossRef.
- Q. Q. Dou, A. Rengaramchandran, S. T. Selvan, R. Paulmurugan and Y. Zhang, Core–shell upconversion nanoparticle-semiconductor heterostructures for photodynamic therapy, Sci. Rep., 2015, 5, 8252 CrossRef CAS.
- H. J. Wang, R. Shrestha and Y. Zhang, Encapsulation of photosensitizers and upconversion nanocrystals in lipid micelles for photodynamic therapy, Part. Part. Syst. Charact., 2013, 31, 228–235 CrossRef.
- D. Bechet, P. Couleaud, F. Céline, M. Viriot and F. Guillemin, Nanoparticles as vehicles for delivery of photodynamic therapy agents, Trends Biotechnol., 2008, 26, 612–621 CrossRef CAS.
- S. P. Singh, Multifunctional magnetic quantum dots for cancer theranostics, J. Biomed. Nanotechnol., 2011, 7, 95–97 CrossRef CAS.
- W. Chen, Nanoparticle self-lighting photodynamic therapy for cancer treatment, J. Biomed. Nanotechnol., 2008, 4, 369–376 CrossRef CAS.
- X. Wang, X. Kong, Y. Yu, Y. Sun and H. Zhang, Effect of annealing on upconversion luminescence of ZnO:Er3+ nanocrystals and high thermal sensitivity, J. Phys. Chem. C, 2007, 111, 15119–15124 CrossRef CAS.
- Y. Sun, Y. Chen, L. Tian, Y. Yu, X. Kong, Q. Zeng, Y. Zhang and H. Zhang, Morphology-dependent upconversion luminescence of ZnO:Er3+ nanocrystals, J. Lumin., 2008, 128, 15–21 CrossRef CAS.
- Y. Li, R. Wang, Y. Xu, W. Zheng and Y. M. Li, Influence of silica surface coating on operated photodynamic therapy property of Yb3+–Tm3+: Ga(III)-doped ZnO upconversion nanoparticles, Inorg. Chem., 2018, 57, 8012–8018 CrossRef CAS.
|
This journal is © The Royal Society of Chemistry 2020 |
Click here to see how this site uses Cookies. View our privacy policy here.