DOI:
10.1039/D0RA06697D
(Paper)
RSC Adv., 2020,
10, 40948-40959
A study on the selective catalytic reduction of NOx by ammonia on sulphated iron-based catalysts
Received
3rd August 2020
, Accepted 8th October 2020
First published on 10th November 2020
Abstract
A series of sulphated iron-based catalysts was prepared via an impregnation method by changing the loading content of Fe3+ and SO42− on ZrO2, and their performance in the selective catalytic reduction (SCR) of NOx by ammonia was investigated. The NOx conversion exhibited large differences among the sulphated iron-based catalysts. To explore the synergistic mechanism of iron and sulphates, XRD, BET, H2-TPR, XPS, TPD and in situ DRIFTS were used to characterize the catalysts, and it was found that among all the catalysts, the NOx conversion by Fe2SZr was greater than 90% at 350–450 °C. The results indicated that the interaction between Fe3+ and SO42− can have an effect on the redox ability, acid sites, and adsorption of NOx and NH3. With an increase in the content of Fe3+, the redox activity of the catalyst and the adsorption of ammonia improved at medium and low temperatures. However, at higher temperatures, an increase in Fe3+ led to a decrease in the conversion of NOx due to the enhanced oxidation of NH3. At medium and low temperatures, an increase in the content of SO42− decreased the concentration of Fe3+ on the surface of the catalyst and inhibited the adsorption of NOx and NH3. The addition of SO42− reduced the redox activity of the catalyst and inhibited the oxidation reaction of NH3, which follows the Eley–Rideal mechanism at high temperatures, further enhancing the SCR activity of the FexSyZr catalyst.
1. Introduction
The temperature of the waste gas of a bituminous coal-fired power plant is generally 300–450 °C.1,2 In particular, the temperature after burning lignite reaches up to 420 °C. Also, under conditions with a high sulphur content, the commercially available catalysts (vanadium-based catalysts) show low denitrification activity and poor N2 selectivity, which make them easily poisoned and secondary pollution being released into the environment.3–6 Therefore, it is urgent to develop environment-friendly catalysts with sulphur resistance, high activity, and high N2 selectivity at high temperature.7–9
Iron-based catalysts exhibit a good NOx conversion rate and N2 selectivity, and thus, have been favored by many researchers.10–17 However, the selective catalytic reduction (SCR) activity of α-Fe2O3 catalysts at temperatures above 300 °C significantly decreases, and thus they cannot be applied in the denitrification of waste gas after burning coal. In recent years, some researchers attempted to improve the performance of catalysts by sulphating them. Gu et al.18 carried out the sulphation of CeO2 via a gas phase method, achieving a reaction activity of up to 99% in the temperature range of 300–500 °C. Ma et al.19 prepared Fe2(SO4)3/TiO2 via impregnation, exhibiting a conversion rate of 97% and N2O selectivity lower than 5% at 350–450 °C. Therefore, the sulphation of catalysts is a very effective way to improve their reactivity and N2 selectivity at high temperature. According the literature,20–25 the high catalytic efficiency and good sulfur resistance of catalysts upon acidification treatment can be mainly ascribed to the following reasons: (1) the reaction with the active material improves the transformation between the active material ion, increasing the reaction rate. (2) The number of Brönsted acid sites on the surface of the catalyst increases, increasing the strength of the acid sites, which is the main reason for the increase in catalytic activity. (3) Reaction with other substances on the catalyst to form sulfates, which enclose the active substances and reduce the sulphation of the active substances or act as active substances. Importantly, the NOx conversion efficiency and N2 selectivity of the sulphated catalysts improved.
To date, the synergistic mechanism between the active components and sulphates has not been reported. Therefore, we used Fe as an active component, which was sulphated and loaded onto a ZrO2 carrier, to prepare a catalyst with a high NOx conversion rate and high N2 selectivity at high temperature. In this study, we loaded Fe3+ and SO42− in different amounts on the ZrO2 carrier via the impregnation method. The impact of Fe3+ and SO42− loading on the performance of the NH3-SCR catalyst was investigated using different characterization methods, and the synergistic mechanism of Fe3+ and SO42− in the SCR reaction was discussed.
2. Experimental
2.1 Catalyst preparation
All catalysts were prepared via the incipient wetness method. ZrO2 power was weighed and dissolved in deionized water. Subsequently, Fe(NO3)3·9H2O and (NH4)2SO4 were added to the solution dropwise, and then heated to 70 °C and stirred to form a paste. The resulting mixture was dried at 120 °C overnight and calcined in air at 500 °C for 4 h. Finally, a series of iron-based catalysts was obtained, denoted as FexSyZr (y = 5, x = 0, 2, 3.5, 7 is denoted as FexSZr and x = 3.5, y = 0, 5, 10, 15 is denoted as FeSyZr; where x represents the weight percentage of Fe3+ and y represents the weight percentage of SO42−).
2.2 Catalyst characterization
The gas concentration was detected using a Gasmet Dx-4000 FT-IR gas analyser, which can monitor NH3, NO2, NO, N2O and water vapour. The reactant gas was 500 ppm NH3, 500 ppm NO, 3% O2 and N2 balanced.
X-ray diffraction (XRD) measurements were performed on a D/MAX-RB system with Cu Ka radiation. The diffraction curves were measured in the 2θ range 10° to 90° with a step size of 0.018 at a rate of 1 s per step.
BET measurements were performed using a Quantachrome Autosorb AS-1 System using N2 adsorption at 77 K.
X-ray photoelectron spectroscopy (XPS) was performed using an ESCALab220i-XL electron spectrometer with 300 W Mg Ka radiation under 3 × 10−9 mbar. The binding energies were calibrated using adventitious carbon with C 1s at 284.8 eV.
Temperature-programmed reduction (TPR) curves were measured using a Micromeritics ChemiSorb 2720. Firstly, the samples were preheated to 300 °C and maintained at a constant temperature for 1 h under N2 purging and then cooled to 30 °C. The catalysts were reduced in a flow of N2-based gas containing 10% H2/Ar (50 cm3 min−1), and the temperature was programmed from 30 °C to 1000 °C at a heating rate of 10 °C min−1.
Temperature programmed desorption (NH3-TPD) experiments were conducted in an N2-based mixture gas containing 500 ppm NH3 (300 mL min−1). The sample was preheated at 500 °C constantly for 1 h, then cooled to 30 °C, and NH3 was introduced continuously for 1 h. Thirdly, the catalyst was blown with N2 until to no NH3 was detected. Finally, the catalyst was heated from 30 °C to 500 °C at a heating rate of 10 °C min−1.
In situ DRIFTS spectra were characterized using a Nicolet NEXUS 870 FT-IR spectrometer. The sample was pre-treated at 300 °C for 1 h under a flow of N2 (100 cm3 min−1).
3. Results and discussion
3.1 The effects on SCR activity with different loadings of Fe3+ and SO42−
Fig. 1(a) shows the SCR activities of the SZr, Fe2SZr, Fe3.5SZr and Fe7SZr catalysts. According to this figure, the NOx conversions of S5Zr catalyst were very low in the temperature range of 150–500 °C and it reached the highest of 12% at 500 °C. Upon the addition of 2% Fe3+, the NOx conversions improved significantly, climbing rapidly from 10% at 250 °C to 90% at 350 °C, and reached the highest of 98% at 400 °C. Subsequently, with a further increase in the temperature to 500 °C, the NOx conversion decreased to 57%. With a continuous increase in the loading of Fe3+, the NOx conversions were slightly higher than that of the Fe2SZr catalyst in the temperature range of 250–350 °C, but they dropped significantly in the temperature range of 400–500 °C, which was negative at 500 °C.
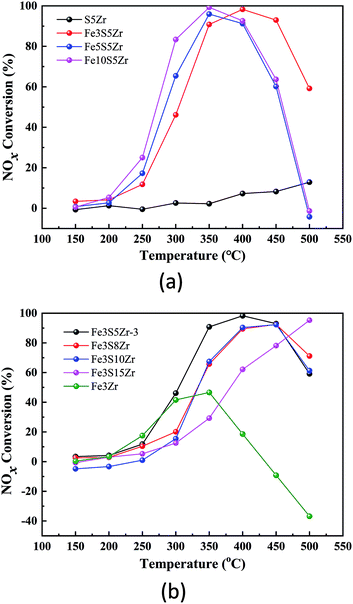 |
| Fig. 1 (a) NOx conversion of catalysts containing 5% SO42− and x% Fe3+ (x = 0, 2, 3.5, 7). (b) NOx conversion of catalysts containing 3.5% Fe3+ and y% SO42− (y = 0, 5, 10, 15). | |
Fig. 1(b) shows the NOx conversions by the FeZr, FeS5Zr, FeS10Zr and FeS15Zr catalysts in the temperature range of 150–500 °C. According to this figure, the NOx conversions of the FeZr, FeS5Zr and FeS10Zr catalysts increased initially and then declined in the temperature range of 150–500 °C. The NOx conversions of the FeZr catalyst were very close to that of the FeS5Zr catalyst in the temperature range of 150–300 °C, which reached the peak of 46% at 350 °C, and then dropped sharply to a negative value from 400 °C to 500 °C. Upon the loading of SO42−, the NOx conversions improved in the high temperature range. When 10% SO42− was added to the catalyst, the NOx conversions were reduced in the temperature range of 250–450 °C compared with that of the FeS5Zr catalyst. With the further addition of SO42− to 15%, the NOx conversion exhibited an increasing trend from 150 °C to 500 °C, which was much lower than that of the FeS5Zr catalyst in the range of 150–450 °C but it reached close to 100% at 500 °C.
3.2 Impact of Fe3+ and SO42− loading on ammonia oxidation
Fig. 2(a) shows the curve of ammonia oxidation using a series of acidified catalysts in the temperature range of 150–500 °C. Accordingly, the SZr catalyst without Fe3+ loading started to oxidize NH3 beginning at 400 °C and reached an NH3 oxidation rate of 40% at 500 °C. However, it was reported that the self-oxidation reaction of NH3 occurs when the temperature is higher than 400 °C, and thus, we speculated that a self-oxidation reaction mainly occurred on the SZr catalyst. With an increase in the loading amount of Fe3+, the conversion of NH3 oxidation shifted to a lower temperature, that is, the greater the loading amount of Fe3+, the more the starting temperature of NH3 oxidation was shifted to a lower temperature and the higher the corresponding oxidation rate of NH3 in the temperature range of 300–450 °C. However, the NH3 oxidation rates of the Fe3.5SZr and Fe7SZr catalysts were close, indicating that although the higher the loading amount of Fe3+, the higher the NH3 oxidation rate, the NH3 oxidation rate approached a constant at a certain temperature with a continuous increase in Fe3+. In general, an increase in the amount of Fe3+ will promote the oxidation of NH3 at high temperature. Combining the activities of the catalysts with varying amounts of Fe3+, the NH3 self-oxidation reaction mainly occurred on the Fe2SZr, Fe3.5SZr and Fe7SZr catalysts at 450–500 °C, which affected the efficiency of the catalytic reduction of the nitrogen oxides. Fig. 2(b) shows the changes in the curves of the NH3 oxidation rate with the catalysts prepared by changing the content of sulphates. Accordingly, for the FeZr catalyst in the absence of sulphates, the oxidation reaction of NH3 started from 250 °C, and the NH3 oxidation rate reached 80% at 350 °C, while it reached 100% at 400 °C. Thus, based on the curve of the NH3 oxidation rate for the FeZr catalyst, it can be concluded that in the temperature range of 350–500 °C, the NH3 oxidation reaction mainly occurred on the FeZr catalyst, thus affecting the SCR reaction. With an increase in the sulphate content, the most efficient ammonia oxidation shifted to a higher temperature, that is, the addition of sulphates inhibited the oxidation reaction of NH3 on the catalyst.
 |
| Fig. 2 (a) NH3 oxidation efficiency of catalysts containing 5% SO42− and x% Fe3+ (x = 0, 2, 3.5, 7). (b) The NH3 oxidation efficiency of catalysts containing 3.5% Fe3+ and y% SO42− (y = 0, 5, 10, 15). | |
3.3 H2-temperature programmed reduction (TPR) analysis
The redox ability of catalysts is of great importance for the SCR reaction, which can be well characterized by H2-temperature programmed reduction (TPR). Actually, the lower the temperature, the better the redox ability of the catalyst at low or medium temperatures.26 The amount of hydrogen consumption is also an important indicator of the catalytic activity. The greater the hydrogen consumption, the stronger the redox capacity of the catalyst.26–28 As shown in Fig. 3(a), there is only one H2 reduction peak for the SZr catalyst at 583 °C, which is mainly attributed to the reduction peak of the sulphate species. According to the H2-TPR curves of the series of Fe2SZr catalysts, the reduction temperatures of the catalysts after the addition of Fe3+ were lower than that of SZr. The Fe3.5SZr and Fe2SZr catalysts each showed only one redox peak, and these peaks were close to each other at 471 °C and 443 °C. These two reduction peaks are considered to be the completely overlapping reduction peaks of the iron oxide and the sulphates. The H2-TPR redox curve of the Fe7SZr catalyst has two reduction peaks at 425 °C and 473 °C. The former reduction peak can be attributed to the reduction peak of the iron oxide, while the peak at 473 °C is considered to be the overlapping reduction peaks of the iron oxide and the sulphates. As shown in Fig. 3(b), the H2-TPR curve of the FeZr catalyst mainly has three reduction peaks at 330 °C and 410 °C, which can be attributed to the reduction peaks of Fe2O3–Fe3O4–Fe.19 After the addition of SO42−, the reduction temperatures of the catalysts are higher than that of FeZr. The FeS10Zr catalyst has only one reduction peak at 519 °C, which is close to the reduction peak of the SZr catalyst, and it is also considered to be the overlapping reduction peaks of the iron oxide and sulphates. The H2-TPR redox curve of the FeS15Zr catalyst has two reduction peaks at 528 °C and 552 °C. The former reduction peak can be attributed to the reduction peak of the iron oxide, while that at 552 °C is considered to be the overlapping reduction peaks of iron oxide and sulphates. According to Fig. 3, it can be concluded that the increase in the Fe3+ content in the catalyst caused a shift in the starting reduction temperature of the catalyst to a lower temperature, indicating that an increase in the content of Fe3+ increased the redox activity of the catalyst, and the addition of SO42− caused the starting reduction temperature of the catalyst to shift to a higher temperature, indicating that the addition of SO42− significantly reduced the redox activity of the catalyst.
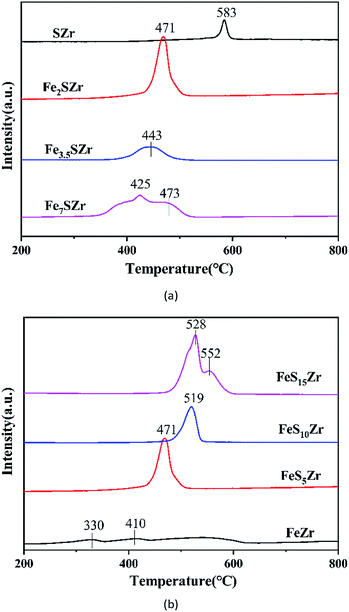 |
| Fig. 3 (a) H2-TPR of catalysts containing 5% SO42− and x% Fe3+ (x = 0, 2, 3.5, 7). (b) H2-TPR of catalysts containing 3.5% Fe3+ and y% SO42− (y = 0, 5, 10, 15). | |
3.4 XPS
Table 1 shows the atomic concentrations of S, O, Fe and Zr on the surfaces of the different catalysts. Accordingly, when the content of Fe3+ was constant, with an increase in SO42−, the concentration of Fe atoms on the surface showed a decreasing trend. This is mainly due to the formation of Fe2(SO4)3. When the content of SO42− was constant, with an increase in Fe2O3, the concentration of S atoms on the surface of the catalyst increased initially, and then decreased. Similarly, we concluded that more Fe2(SO4)3 was produced so that the Fe and S atoms were enriched on the surface. However, when the content of Fe3+ increased to a certain level, the SO42− on the surface was covered by Fe3+, and thus, the concentration of S atoms on the surface of the catalyst showed the tendency to increase initially, and then decrease with an increase in Fe3+.
Table 1 The surface element content in the different catalysts (%)
|
FeZr |
FeS5Zr |
FeS10Zr |
FeS15Zr |
SZr |
Fe3.5SZr |
Fe7SZr |
S 2p |
0.000 |
0.077 |
0.076 |
0.091 |
0.062 |
0.080 |
0.066 |
Zr 3d |
0.217 |
0.161 |
0.184 |
0.164 |
0.212 |
0.132 |
0.131 |
O 1s |
0.698 |
0.719 |
0.722 |
0.723 |
0.726 |
0.695 |
0.690 |
Fe 2p |
0.074 |
0.045 |
0.018 |
0.021 |
0.000 |
0.093 |
0.113 |
The oxidation states of Fe and S on the different catalysts were characterized using XPS, and the results are shown in Fig. 4. The Fe 2p and S bands in Fig. 4 were deconvoluted by searching for the optimal combination with correlation coefficients (R2) above 0.99 (Origin Pro 8.0). From the curves of Fe 2p, as shown in Fig. 4(a and b), the bonding energies of Fe 2p3/2 (710–711.5 eV) and Fe 2p1/2 (724.3–725.5 eV) corresponded well to the references,29 and the bonding energies of 718.1–719.2 eV corresponded well to the fingerprint peak of Fe3+. Specially, when the content of SO42− was 5%, with an increase in Fe3+, the peak with a binding energy at 718.1–719.2 eV became strong, and when the Fe3+ content was 3.5%, with an increase in SO42−, the peak at 718.1–719.2 eV became weak.
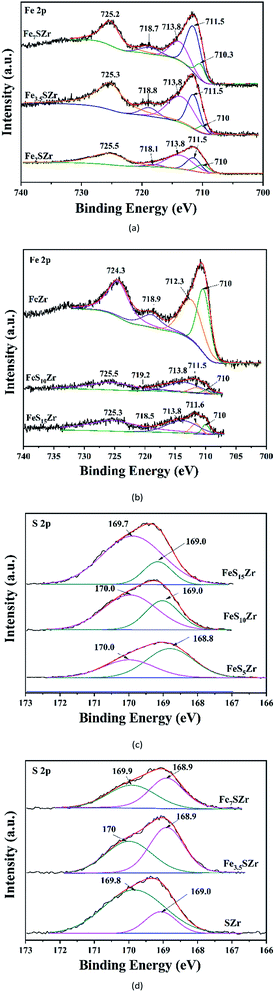 |
| Fig. 4 XPS spectra of different catalysts: (a) XPS-Fe 2p spectra of catalysts containing 5% SO42− and x% Fe3+ (x = 0, 2, 3.5, 7). (b) XPS-Fe 2p spectra of catalysts containing 3.5% Fe3+ and y% SO42− (y = 0, 5, 10, 15). (c) XPS-S 2p spectra of catalysts containing 5% SO42− and x% Fe3+ (x = 0, 2, 3.5, 7). (d) XPS-S 2p spectra of catalysts containing 3.5% Fe3+ and y% SO42− (y = 0, 5, 10, 15). | |
The S 2p XPS spectra for the different catalysts are shown in Fig. 4(c and d). The S 2p XPS spectra of the sulfated catalysts exhibited a main peak at 168.8–170 eV, as shown in Fig. 4. This value is consistent with S6+ such as sulfur in SO42−,19 indicating that S is in the S6+ oxidation state on the catalyst.
3.5 NH3-temperature programmed desorption (TPD) analysis
The acidity of catalysts plays a very important role in the SCR reaction, and Fe3+ and sulphates can provide acidic sites, which can improve the acidity of the catalyst. Therefore, the characteristics of NH3-TPD were analysed for a series of acidified catalysts, as shown in Fig. 5. Fig. 5(a) shows the NH3-TPD curves for the catalysts with different Fe3+ loadings. The SZr catalyst has two desorption peaks at 410 °C and 120 °C. The desorption amount of ammonia at high temperature was much larger than that at low temperature. It has been reported that the desorption at low temperature is mainly physical adsorption or weak chemical adsorption, while the desorption at high temperature is mainly the strong chemical adsorption of NH3.30–32 On the Fe2SZr catalyst, there was a wide NH3 desorption peak in the range of 80 °C to 500 °C. With the addition of Fe3+, the desorption capacity of Fe3.5SZr at low temperature (90–180 °C) was slightly higher than the desorption at high temperature (>180 °C). Compared to SZr, the desorption peak of the Fe7SZr catalyst at high temperature (240 °C) shifted to a lower temperature at nearly 170 °C. Thus, it can be concluded that the addition of Fe2O3 reduced the ability of the catalyst to adsorb ammonia at high temperatures and improved the ability of the catalyst to adsorb ammonia at moderate and low temperatures. Fig. 5(b) shows the NH3-TPD curves for the catalysts with different amounts of sulphates. As shown, only a small amount of physically adsorbed ammonia was released on the FeZr catalyst. The addition of 5% sulphate greatly increased the adsorption amount of NH3. With an increase in the amount of sulphate, the desorption peak of ammonia obviously shifted towards a higher temperature, and the FeS15Zr catalyst showed a desorption peak at 410 °C, which is consistent with the desorption peak of the SZr catalyst, but the desorption amount of ammonia was much less than that of the SZr catalyst. Thus, the addition of SO42− increased the adsorption of ammonia on the catalyst at high temperatures and reduced the amount of ammonia adsorbed by the catalyst at moderate or low temperatures.
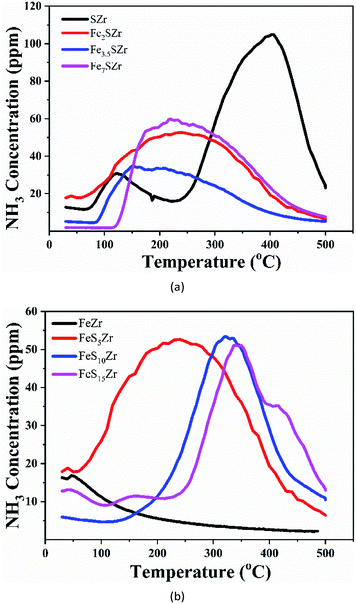 |
| Fig. 5 (a) NH3-TPD of catalysts containing 5% SO42− and x% Fe3+ (x = 0, 2, 3.5, 7). (b) NH3-TPD of catalysts containing 3.5% Fe3+ and y% SO42− (y = 0, 5, 10, 15). | |
3.6 NO + O2-TPD
Fig. 6 shows the NOx-TPD curve for a series of acidified catalysts. As shown in Fig. 6(a), the SZr catalyst mainly has a nitrogen oxide desorption peak at 120 °C with a low desorption amount. After the addition of 2% Fe3+, nitrogen oxides were desorbed from 100 °C to 500 °C, and the amount of nitrogen oxides desorbed was greatly enhanced. When the addition of Fe3+ reached 3.5%, the desorption peak shifted to a higher temperature by approximately 40 °C, but the desorption amount was significantly reduced. When the addition of Fe3+ reached 7%, two desorption peaks at 90 °C and 230 °C existed, and the desorption amount was greater than that of the Fe2SZr catalyst. As shown in Fig. 6(b), the FeZr catalyst has a nitrogen oxide desorption peak at 240 °C with the highest amount of desorption. The addition of 5% SO42− to the catalyst significantly decreased the desorption of nitrogen oxides, indicating that SO42− had an inhibitory effect on the adsorption of nitrogen oxides. The further addition of SO42− to the FeS10Zr catalyst significantly increased the adsorption of nitrogen oxides at low temperature, but decreased the adsorption of nitrogen oxides at high temperature. When the addition of SO42− reached 15%, the adsorption of nitrogen oxides was basically completely suppressed. Because NO can be easily oxidized into NO2 in the presence of O2, and NO together with NO2 are both acidic gases, the addition of Fe3+ to the catalyst enhanced its adsorption capacity of nitrogen oxides, while the addition of SO42− to the catalyst will inhibited the adsorption capacity of nitrogen oxides. However, due to the synergistic effect between Fe3+ and SO42−, the desorption amount of nitrogen oxides of the Fe3.5SZr catalyst was much less than that of the Fe2SZr catalyst.
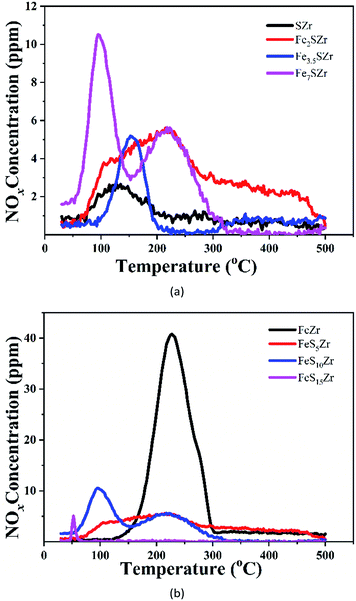 |
| Fig. 6 (a) NO + O2-TPD of catalysts containing 5% SO42− and x% Fe3+ (x = 0, 2, 3.5, 7). (b) The NO + O2-TPD of catalysts containing 3.5% Fe3+ and y% SO42− (y = 0, 5, 10, 15). | |
3.7 In situ diffuse reflectance infrared Fourier transform spectroscopy (DRIFTS)
3.7.1 NH3 species adsorbed on the different catalysts. Fig. 7(a) shows the infrared spectra of a series of catalysts containing 5% SO42− and different amounts of Fe3+ with the saturated adsorption of NH3 at 30 °C and after purging with N2. According to the different reports in the literature and the comparative analysis,26 the region of 3400–2800 cm−1 should be responsible for the NH stretching vibration, which can be considered as the coordination adsorption formed between NH3 and the oxygen atoms of the metal oxide on the catalyst surface by hydrogen bonding. The absorption at 1600 cm−1 is the antisymmetric stretching vibration absorption peak of the NH3 species adsorbed at the Lewis acid sites, while the peaks at 1230, 1210 and 1200 cm−1 are attributed to the symmetric stretching vibration absorption peaks of the NH3 species adsorbed at the Lewis acid sites. The peaks at 1440, 1470, 1650, and 1700 cm−1 are attributed to the anti-symmetric and symmetric stretching vibration absorption peaks of the NH4+ species adsorbed at the Brönsted acid sites.33 It is worth noting that the absorption peak at 1800 cm−1 is not attributed to the absorption of NH3 or NH4+ with an increase in the amount of Fe3+. The increase in the amount of Fe3+ led to the anti-symmetric stretching vibration absorption peak of the NH3 species adsorbed at the Lewis acid sites. Simultaneously, we found that with a further increase in the amount of Fe3+, the amount of NH4+ species adsorbed at the Brönsted acid sites first increased and then decreased. As shown in Fig. 7(b), the increase in the amount of SO42− significantly increased the anti-symmetric and symmetric stretching vibration absorption peaks of the NH4+ species adsorbed at the Brönsted acid sites, but a further increase in the amount of SO42− did not continue to increase the NH4+ species adsorbed at the Brönsted acid sites, which decreased instead, and this may be due to the synergistic effect of iron and SO42−.
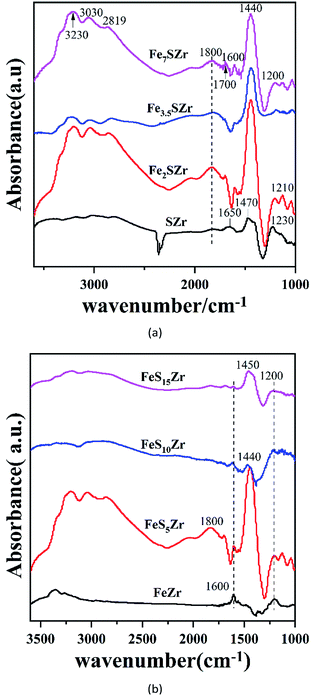 |
| Fig. 7 Infrared spectra of a series of catalysts with the saturated adsorption of NH3 at 30 °C and after purging with N2: (a) catalysts containing 5% SO42− and x% Fe3+ (x = 0, 2, 3.5, 7) and (b) catalysts containing 3.5% Fe3+ and y% SO42− (y = 0, 5, 10, 15). | |
3.7.2 The NOx species adsorbed on the surfaces of the different catalysts. Fig. 8(a) shows the infrared spectra of a series of catalysts containing 5% SO42− and different amounts of Fe3+ with the saturated adsorption of NO + O2 at 30 °C and after purging with N2. At 30 °C, the nitrogen oxide vibration absorption peaks appeared at 1630, 1560, 1440, 1280, 1260 and 1240 cm−1. According to the literature,34–37 the vibration absorption peaks at 1630 and 1560 cm−1 are attributed to the vibration absorption of the bridged and bidentate nitrate species adsorbed on the catalyst, respectively, and the vibration absorption peaks at 1440, 1280, 1260 and 1240 cm−1 are attributed to the linear nitrate species adsorbed on the catalyst. With the addition of Fe3+, the vibration absorption peak of the linear nitrate species at 1440 cm−1 increased, and the anti-symmetric and symmetric vibration absorption peaks of nitrogen oxides at 1230 cm−1 disappeared, showing that the linear nitrate species adsorbed at 1260 cm−1. Combined with the NO-TPD results, with the addition of Fe3+, the catalyst could readily adsorb the unstable nitrate species (e.g., linear nitrates), and the desorption temperatures of all the adsorbed nitrates shifted towards a lower temperature. Fig. 8(b) shows the infrared spectra of a series of catalysts containing 3.5% Fe3+ and different amounts of SO42− with the saturated adsorption of NO + O2 at 30 °C and after purging with N2. With the addition of SO42− to the catalyst, the NO2 species (1620 cm−1) and the linear nitrate species (1280 cm−1) on the catalyst surface were reduced, and the vibration absorption peak of the nitrate species showed a red-shift (from 1520 cm−1 to 1580 cm−1), with a stable nitrate species appearing at 1440 cm−1. The addition of SO42− inhibited the adsorption of nitrogen oxides on the catalyst, which is consistent with the NOx-TPD results.
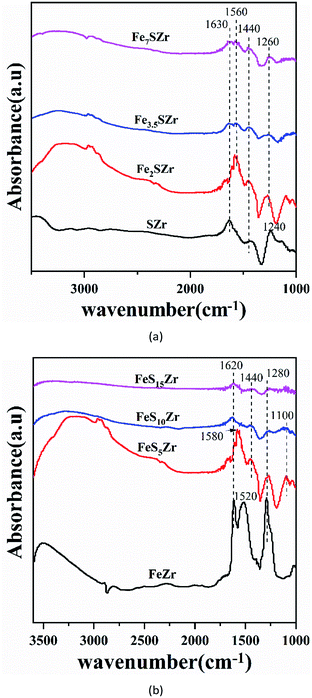 |
| Fig. 8 Infrared spectra of a series of catalysts with the saturated adsorption of NO + O2 at 30 °C and after purging with N2: (a) shows catalysts containing 5% SO42− and x% Fe3+ (x = 0, 2, 3.5, 7) and (b) catalysts containing 3.5% Fe3+ and y% SO42− (y = 0, 5, 10, 15). | |
3.7.3 Transient DRIFTS for the Fe7S5Zr and Fe2S15Zr catalysts. To better understand the SCR reaction on the sulphated catalysts at high temperature and comparatively analyse the effects of additional Fe3+ and SO42− on the mechanism of the NH3-SCR catalytic reaction, we investigated the transient DRIFTS for the Fe7SZr and FeS15Zr catalysts.
3.7.3.1 Transient DRIFTS of the surface of the Fe7SZr catalyst at 300 °C. Fig. 9(a) shows the profile of the time-dependent changes of the adsorbed species on the surface of the Fe7SZr catalyst at 300 °C after introducing NH3 with the saturated adsorption of NO + O2. Accordingly, after the adsorption of NO + O2 on the catalyst surface was saturated, the adsorption peaks appeared at 1620, 1370 and 1140 cm−1. The surface of the catalyst was mainly covered by NO2 species (1620 cm−1) and nitrate species (1370 and 1140 cm−1).38 After NH3 was introduced for 1 min, the adsorbed nitrogen oxide species began to decrease. After 3 min, the absorption peaks of the adsorbed nitrogen oxide species disappeared, and the adsorption peak attributed to the NH4+ species at 1430 cm−1 appeared, together with the absorption peaks for NH3 (1280 and 1600 cm−1) and NH4+ (1410 and 1690 cm−1) species. After 10 min, the absorption peak of NH4+ on the surface of the catalyst gradually increased. These results indicate that the nitrogen oxide species adsorbed on the Fe7SZr catalyst surface can react rapidly with NH3 in the gas phase.
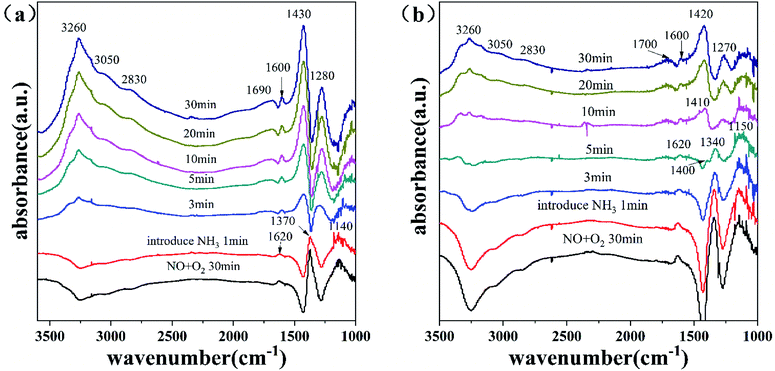 |
| Fig. 9 Profile of the time-dependent changes of the adsorbed species on the surface of different catalysts at 300 °C after introducing NH3 with the saturated adsorption of NO + O2 on the (a) Fe7SZr catalyst and (b) FeS15Zr catalyst. | |
Fig. 10(a) shows the profile of the time-dependent changes of the adsorbed species on the surface of the Fe7SZr catalyst at 300 °C after introducing NO + O2 with the saturated adsorption of NH3. When the NH3 adsorption was saturated, the vibration absorption peaks of NH3 (1280 cm−1) and NH4+ (1680 and 1430 cm−1) on the surface of the catalyst appeared. When NO + O2 was introduced onto the catalyst surface, the absorption peak of the ammonia species on the surface of the catalyst did not change significantly. After 5 min, the intensities of all the absorption peaks of the adsorbed NH3 species began to weaken, and even after 30 min, the adsorbed NH3 species had not reacted completely, and the absorption peak of nitrogen oxide did not appear. This demonstrated that the introduction of NO + O2 caused a weak reaction with various types of adsorbed NH3 species on the surface of the catalyst. Thus, combined with Fig. 10(a) and 9(a), at 300 °C the nitrogen oxides adsorbed on the surface of the Fe7SZr catalyst were the main components that reacted very rapidly with NH3 in the gaseous state, and the various NH3 species adsorbed on the surface could also slowly participate in the reaction.
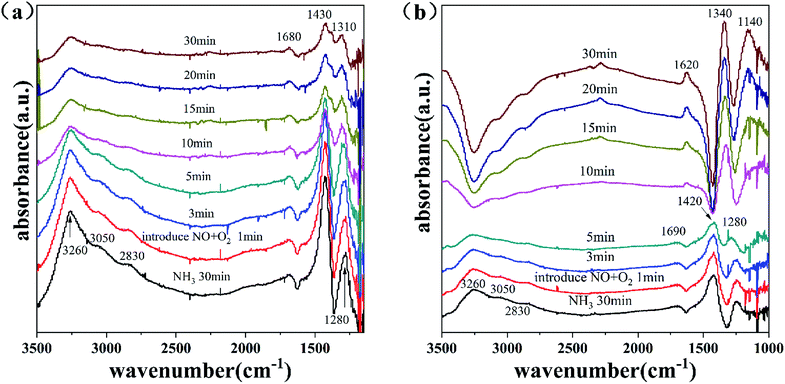 |
| Fig. 10 Profile of the time-dependent changes of the adsorbed species on the surface of different catalysts at 300 °C after introducing NO + O2 with the saturated adsorption of NH3 on the (a) Fe7SZr catalyst and (b) FeS15Zr catalyst. | |
3.7.3.2 Transient DRIFTS of the surface of the FeS15Zr catalyst at 300 °C. Fig. 9(b) shows the profile of the time-dependent changes of the adsorbed species on the surface of the FeS15Zr catalyst at 300 °C after introducing NH3 with the saturated adsorption of NO + O2. Accordingly, after the adsorption of NO + O2 on the catalyst surface was saturated, the adsorption peaks appeared at 1620, 1340 and 1150 cm−1. The surface of the catalyst was mainly covered by NO2 species (1620 cm−1) and nitrate species (1340 and 1150 cm−1).39 After NH3 was introduced, the adsorbed nitrogen oxides species were gradually weakened in 5 min. At the beginning of the 5th minute, the adsorption peak attributed to the NH4+ species appeared at 1400 cm−1. At the 10th minute, the absorption peaks of the nitrogen oxide species basically disappeared, and the absorption peaks of NH3 (1270 and 1600 cm−1) and NH4+ (1410 and 1700 cm−1) species appeared. After 10 min, the catalyst surface was covered by various ammonia species, and they gradually increased. These results indicate that the nitrogen oxide species adsorbed on the surface of the FeS15Zr catalyst can react with NH3 in the gas phase.Fig. 10(b) shows the profile of the time-dependent changes of the adsorbed species on the surface of the FeS15Zr catalyst at 300 °C after introducing NO + O2 with the saturated adsorption of NH3. After NO + O2 was introduced on the catalyst surface for 5 min, all the adsorbed NH3 species disappeared. Starting from the 10th minute, the surface was gradually occupied by nitrate. This indicates that NO + O2 can react with the various NH3 species adsorbed on the surface. Thus, combined with Fig. 10(b) and 9(b), at 300 °C the NH3 species adsorbed on the surface of the FeS15Zr catalyst can rapidly react with NOx in the gas phase, while the nitrogen oxide species adsorbed on the surface can also rapidly react with NH3 in the gas phase.
Combined with the results of the DRIFTs study of the transient reactions of the Fe7SZr and FeS15Zr catalysts at 300 °C, it can be concluded that there are two NH3-SCR reaction paths on the surface of the sulphated iron-based catalyst at 300 °C, namely a Langmuir–Hinshelwood15 reaction mechanism and an Eley–Rideal27,33 reaction mechanism, that is, NH3 adsorption on the catalyst surface. NO is first oxidized into NO2 to be adsorbed on the surface of the catalyst to produce nitrates or nitrite species, and the NH3 species adsorbed on the surface can react with both gaseous NOx and the adsorbed NOx species to produce N2 and H2O. The nitrogen oxide species adsorbed on the surface of the Fe7SZr catalyst can react very rapidly with the gaseous NH3, while various NH3 species adsorbed on the surface can also slowly participate in the reaction, indicating that when the amount of Fe2O3 in the catalyst increases, the SCR reaction occurring on the catalyst obviously follows the Langmuir–Hinshelwood reaction path, with a small portion of the reaction following the Eley–Rideal reaction mechanism simultaneously. The NH3 species adsorbed on the surface of the FeS15Zr catalyst at 300 °C can rapidly react with the NOx in the gas phase, and the nitrogen oxides species adsorbed on the surface can also rapidly react with the NH3 in the gas phase. This indicates that when the amount of SO42− in the catalyst increases, the SCR reaction on the surface of the catalyst obviously follows the Eley–Rideal reaction mechanism, mainly because the increase in the amount of SO42− significantly enhances the ammonia adsorption capacity of the catalyst at a high temperature, while reducing the adsorption of nitrogen oxides, which is conducive to enhancing the NH3 adsorption by the catalyst, thereby promoting the SCR reaction at high temperature. This is consistent with the results of the activity test for the catalyst with varying amounts of SO42−.
4. The synergistic mechanism of Fe3+ and sulphates for NH3-SCR
The roles of Fe3+ and sulphates were very different at low temperature and high temperature. When the SO42− content is constant, the addition of Fe3+ to the catalyst will enhance its adsorption capacity of nitrogen oxides and NH3 species at moderate and low temperatures. According to the references,27,33,40,41 the mechanism of the SCR generally follows the Langmuir–Hinshelwood reaction path at moderate and low temperatures, and thus the Fe3+ obviously adsorbed NH3 and oxidized it to –NH2, which is the important intermediate species for the SCR reaction42–46 and can react with the NO oxidation species (NO2− and NO3−) to form the intermediate species, and then decompose to N2 and H2O. Thus, the addition of Fe3+ improved the redox ability, which affected the activity for the SCR at moderate and low temperatures. However, at high temperature, an increase in the amount of Fe3+ will promote the oxidation of NH3 because it can increase the redox activity of the catalyst, which results in a decrease in the efficiency of the catalytic reduction of nitrogen oxides. When the content of Fe3+ is constant, with an increase in the content of SO42−, the concentration of Fe3+ on the surface showed a decreasing trend because of the formation of Fe2(SO4)3, which leads to the complete inhibition of the adsorption of NOx and NH3 to reduce the conversion of NOx in NH3-SCR at moderate and low temperature. At high temperature, the redox activity of the catalyst is reduced with the addition of SO42−, which restrains the oxidation reaction of NH3 on the catalyst. Meanwhile, the significant increase in NH4+ species adsorbed at the Brönsted acid sites improves the activity for the SCR, which follows the Eley–Rideal reaction path. Overall, the effect on the SCR for Fe3+ and SO42− was restrained by each of them, and thus only the appropriate contents of Fe3+ and SO42− could result in high activity in the temperature range of 300–450 °C. Similar to oxide catalysts such as Mo–Fe,47 W–Fe,48 W–Ce,49 and Mo–Fe50 oxides, the sulphated iron-based catalysts possess acid-redox dinuclear sites, where Fe3+ is the redox site and SO42− is the acid site. The interfaces of the acid-redox sites are key to the SCR reaction activities. Thus, designing catalysts to optimize the interaction between the acid-redox sites is an effective measure to control NO emissions.
5. Conclusions
Different sulphated iron-based catalysts with various loadings of Fe3+ and SO42− on ZrO2 were prepared via the incipient wetness method, and investigated for the selective catalytic reduction (SCR) of NOx by ammonia. Compared to all the catalysts, the Fe2SZr catalyst could achieve above 90% NOx removal efficiency at 350–450 °C, and the main reactions on its surface were the NH3-SCR reaction and the oxidation of NH3. Meanwhile, the interaction between Fe3+ and SO42− was found to affect the redox ability, acid sites, adsorption of NOx and NH3, and play a role in the SCR activity. Specifically, increasing the content of Fe3+ improved the redox activity of the catalyst and enhanced the adsorption of ammonia at medium and low temperatures. In addition, increasing the amount of Fe3+ promoted the oxidation of NH3 at high temperatures, which led to a reduction in the efficiency of NOx conversion. With an increase in SO42−, the concentration of Fe3+ on the surface of the catalyst showed a decreasing trend because of the formation of Fe2(SO4)3, which inhibited the adsorption of NOx and NH3 and reduced the NOx conversion of the NH3-SCR at medium and low temperatures. At high temperatures, the addition of SO42− reduced the redox activity of the catalyst and inhibited the oxidation reaction of NH3. Meanwhile, the significant increase in NH4+ species adsorbed on the Brönsted acid sites improved the SCR activity because the main reaction pathway over the FexSyZr catalyst follows the Eley–Rideal mechanism at high temperatures.
Conflicts of interest
There are no conflicts to declare.
Acknowledgements
This work was financially supported by the National High-Tech Research and Science and Technology Plan Program of Hebei Province (Grant No. 206Z3702G) and Development (Air Pollution Control Technology Research) Program of China (Grant No. 2016YFC0205302 and No. 2016YFC0205300) and National Natural Science Fund of China (Grant No. 21507100).
References
- Z. Fu, M. Guo, C. Liu, N. Ji, C. Song and Q. Liu, Design and Synthesis Functional Selective Catalytic Reduction Catalyst for NOx Removal, Procedia Eng., 2015, 121, 952–956 CrossRef CAS.
- M. Jabłońska and R. Palkovits, Copper based catalysts for the selective ammonia oxidation into nitrogen and water vapour—Recent trends and open challenges, Appl. Catal., B, 2016, 181, 332–351 CrossRef.
- G. Busca, L. Lietti, G. Ramis and F. Berti, Chemical and mechanistic aspects of the selective catalytic reduction of NOx by ammonia over oxide catalysts: a review, Appl. Catal., B, 1998, 18, 1–36 CrossRef CAS.
- A. Boubnov, H. W. P. Carvalho, D. E. Doronkin, T. Guenter, E. Gallo, A. J. Atkins, C. R. Jacob and J. D. Grunwaldt, Selective Catalytic Reduction of NO Over Fe-ZSM-5: Mechanistic Insights by Operando HERFD-XANES and Valence-to-Core X-ray Emission Spectroscopy, J. Am. Chem. Soc., 2014, 136, 13006–13015 CrossRef CAS.
- D. Jo, G. T. Park, T. Ryu and S. B. Hong, Economical synthesis of high-silica LTA zeolites: a step forward in developing a new commercial NH3-SCR catalyst, Appl. Catal., B, 2019, 243, 212–219 CrossRef CAS.
- J. Mu, X. Li, W. Sun, S. Fan, X. Wang, L. Wang, M. Qin, G. Gan, Z. Yin and D. Zhang, Inductive Effect Boosting Catalytic Performance of Advanced Fe1–xVxOδ Catalysts in Low-Temperature NH3 Selective Catalytic Reduction: Insight into the Structure, Interaction, and Mechanisms, ACS Catal., 2018, 8, 6760–6774 CrossRef CAS.
- P. Fabrizioli, T. Bürgi and A. Baiker, Environmental Catalysis on Iron Oxide–Silica Aerogels: Selective Oxidation of NH3 and Reduction of NO by NH3, J. Catal., 2002, 206, 143–154 CrossRef CAS.
- S. Ding, F. Liu, X. Shi and H. Hong, Promotional effect of Nb additive on the activity and hydrothermal stability for the selective catalytic reduction of NOx with NH3 over CeZrOx catalyst, Appl. Catal., B, 2016, 180, 766–774 CrossRef CAS.
- C. Sun, H. Liu, W. Chen, D. Chen, S. Yu, A. Liu, L. Dong and S. Feng, Insights into the Sm/Zr co-doping effects on N2 selectivity and SO2 resistance of a MnOx-TiO2 catalyst for the NH3-SCR reaction, Chem. Eng. J., 2018, 347, 27–40 CrossRef CAS.
- S. Yang, F. Qi, S. Xiong, D. Hao and J. Li, MnOx supported on Fe-Ti spinel: a novel Mn based low temperature SCR catalyst with a high N2 selectivity, Appl. Catal., B, 2016, 181, 570–580 CrossRef CAS.
- R. Zhang, Y. Li and T. Zhen, Ammonia selective catalytic reduction of NO over Fe/Cu-SSZ-13, RSC Adv., 2014, 4, 52130–52139 RSC.
- F. Cao, S. Su, J. Xiang, P. Wang, S. Hu, L. Sun and A. Zhang, The activity and mechanism study of Fe-Mn-Ce/γ-Al_2O_3 catalyst for low temperature selective catalytic reduction of NO with NH_3, Fuel, 2015, 139, 232–239 CrossRef CAS.
- B. Shen, T. Liu, N. Zhao and X. Yang, Iron-doped Mn-Ce/TiO2 catalyst for low temperature selective catalytic reduction of NO with NH3, J. Environ. Sci., 2010, 22, 1447–1454 CrossRef CAS.
- W. Zhao, C. Li, P. Lu, Q. Wen, Y. Zhao, X. Zhang, C. Fan and S. Tao, Iron, lanthanum and manganese oxides loaded on γ-Al2O3 for selective catalytic reduction of NO with NH3 at low temperature, Environ. Technol., 2013, 34, 81–90 CrossRef CAS.
- G. Zhou, B. Zhong, W. Wang, X. Guan, B. Huang, D. Ye and H. Wu, In situ DRIFTS study of NO reduction by NH3 over Fe–Ce–Mn/ZSM-5 catalysts, Catal. Today, 2011, 175, 157–163 CrossRef CAS.
- Y. Xia, W. Zhan, Y. Guo, Y. Guo and G. Lu, Fe-Beta zeolite for selective catalytic reduction of NOx with NH3: influence of Fe content, Chin. J. Catal., 2016, 37, 2069–2078 CrossRef CAS.
- Z. Ma, H. Yang, L. Qian, J. Zheng and X. Zhang, Catalytic reduction of NO by NH3 over Fe–Cu–OX/CNTs-TiO2 composites at low temperature, Appl. Catal., A, 2012, 427–428, 43–48 CrossRef CAS.
- T. Gu, L. Yue, X. Weng, H. Wang and Z. Wu, The enhanced performance of ceria with surface sulfation for selective catalytic reduction of NO by NH3, Catal. Commun., 2010, 12, 310–313 CrossRef CAS.
- M. Lei, J. Li, K. Rui and L. Fu, Catalytic Performance, Characterization, and Mechanism Study of Fe2(SO4)3/TiO2 Catalyst for Selective Catalytic Reduction of NOx by Ammonia, J. Phys. Chem. C, 2011, 115, 7603–7612 CrossRef.
- H. Zhang, Y. Zou and Y. Peng, Influence of sulfation on CeO2-ZrO2 catalysts for NO reduction with NH3, Chin. J. Catal., 2017, 38, 160–167 CrossRef CAS.
- Q. Lu, W. Yun, D. Pang, O. Feng and C. Zhang, SO42–Mn-Co-Ce supported on TiO2/SiO2 with high sulfur durability for low-temperature SCR of NO with NH3, Catal. Commun., 2016, 78, 22–25 CrossRef.
- Q. Zhang, J. Zhang, Z. Song, N. Ping and L. Xin, A novel and environmentally friendly SO42–/CeO2 catalyst for the selective catalytic reduction of NO with NH3, J. Ind. Eng. Chem., 2016, 34, 165–171 CrossRef CAS.
- Y. Yu, J. Chen, J. Wang and Y. Chen, Performances of CuSO4/TiO2 catalysts in selective catalytic reduction of NOx by NH3, Chin. J. Catal., 2016, 37, 281–287 CrossRef CAS.
- T. Xu, X. Wu, X. Liu, L. Cao, Q. Lin and D. Weng, Effect of barium sulfate modification on the SO2 tolerance of V2O5/TiO2 catalyst for NH3-SCR reaction, J. Environ. Sci., 2017, 57, 110–117 CrossRef.
- X. Du, X. Wang, Y. Chen, X. Gao and L. Zhang, Supported metal sulfates on Ce–TiOx as catalysts for NH3–SCR of NO: high resistances to SO2 and potassium, J. Ind. Eng. Chem., 2016, 36, 271–278 CrossRef CAS.
- L. Jian, Z. Zhen, J. Wang, C. Xu, A. Duan, G. Jiang and Q. Yang, The highly active catalysts of nanometric CeO2-supported cobalt oxides for soot combustion, Appl. Catal., B, 2008, 84, 185–195 CrossRef.
- N. Apostolescu, B. Geiger, K. Hizbullah, M. T. Jan, S. Kureti, D. Reichert, F. Schott and W. Weisweiler, Selective catalytic reduction of nitrogen oxides by ammonia on iron oxide catalysts, Appl. Catal., B, 2006, 62, 104–114 CrossRef CAS.
- X. Wang, S. Wu, W. Zou, S. Yu and L. Dong, Fe-Mn/Al2O3 catalysts for low temperature selective catalytic reduction of NO with NH3, Chin. J. Catal., 2016, 37, 1314–1323 CrossRef CAS.
- S. Yang, C. Liu, H. Chang, L. Ma, Z. Qu, N. Yan, C. Wang and J. Li, Improvement of the Activity of γ-Fe2O3 for the Selective Catalytic Reduction of NO with NH3 at High Temperatures: NO Reduction versus NH3 Oxidization, Ind. Eng. Chem. Res., 2013, 52, 5601–5610 CrossRef CAS.
- R. Q. Long and R. T. Yang, Selective Catalytic Oxidation of Ammonia to Nitrogen over Fe2O3–TiO2 Prepared with a Sol–Gel Method, J. Catal., 2002, 207, 158–165 CrossRef CAS.
- L. S. Cheng, R. T. Yang and C. Ning, Iron Oxide and Chromia Supported on Titania-Pillared Clay for Selective Catalytic Reduction of Nitric Oxide with Ammonia, J. Catal., 1996, 164, 70–81 CrossRef CAS.
- R. Q. Long and R. T. Yang, The promoting role of rare earth oxides on Fe-exchanged TiO 2-pillared clay for selective catalytic reduction of nitric oxide by ammonia, Appl. Catal., B, 2000, 27, 87–95 CrossRef CAS.
- G. Ramis, L. Yi, G. Busca, M. Turco, E. Kotur and R. J. Willey, Adsorption, Activation, and Oxidation of Ammonia over SCR Catalysts, J. Catal., 1995, 157, 523–535 CrossRef CAS.
- A. Bourane, O. Dulaurent, S. Salasc, C. Sarda, C. Bouly and D. Bianchi, Heats of Adsorption of Linear NO Species on a Pt/Al2O3 Catalyst Using in Situ Infrared Spectroscopy under Adsorption Equilibrium, J. Catal., 2001, 204, 77–88 CrossRef CAS.
- A. Kotsifa, D. I. Kondarides and X. E. Verykios, Comparative study of the chemisorptive and catalytic properties of supported Pt catalysts related to the selective catalytic reduction of NO by propylene, Appl. Catal., B, 2007, 72, 136–148 CrossRef CAS.
- W. Schieer, H. Vinek and A. Jentys, Surface species during catalytic reduction of NO by propene studied by in situ IR-spectroscopy over PT supported on mesoporous Al2O3 with MCM-41 type structure, Appl. Catal., B, 2001, 33, 263–274 CrossRef.
- M. A. Larrubia, G. Ramis and G. Busca, An FT-IR study of the adsorption and oxidation of N-containing compounds over Fe 2O3-TiO2 SCR catalysts, Appl. Catal., B, 2001, 30, 101–110 CrossRef CAS.
- L. J. France, Q. Yang, W. Li, Z. Chen, J. Guang, D. Guo, L. Wang and X. Li, Ceria modified FeMnOx—Enhanced performance and sulphur resistance for low-temperature SCR of NOx, Appl. Catal., B, 2017, 206, 203–215 CrossRef CAS.
- T. Venkov, K. Hadjiivanov and D. Klissurski, IR spectroscopy study of NO adsorption and NO + O2 co-adsorption on Al2O3, Phys. Chem. Chem. Phys., 2002, 4, 2443–2448 RSC.
- J. Liu, X. Li, R. Li, Q. Zhao, J. Ke, H. Xiao, L. Wang, S. Liu, M. Tadé and S. Wang, Facile synthesis of tube-shaped Mn-Ni-Ti solid solution and preferable Langmuir-Hinshelwood mechanism for selective catalytic reduction of NO by NH3, Appl. Catal., A, 2018, 549, 289–301 CrossRef CAS.
- J. Liu, G.-q. Li, Y.-f. Zhang, X.-q. Liu, Y. Wang and Y. Li, Novel Ce-W-Sb mixed oxide catalyst for selective catalytic reduction of NOx with NH3, Appl. Surf. Sci., 2017, 401, 7–16 CrossRef CAS.
- J. Fan, P. Ning, Z. Song, X. Liu, L. Wang, J. Wang, H. Wang, K. Long and Q. Zhang, Mechanistic aspects of NH3-SCR reaction over CeO2/TiO2-ZrO2-SO42– catalyst: in situ DRIFTS investigation, Chem. Eng. J., 2018, 334, 855–863 CrossRef CAS.
- J. Zhang, X. Li, P. Chen and B. Zhu, Research Status and Prospect on Vanadium-Based Catalysts for NH(3)-SCR Denitration, Materials, 2018, 11, 1632 CrossRef.
- J. Liu, R.-t. Guo, M.-y. Li, P. Sun, S.-m. Liu, W.-g. Pan, S.-w. Liu and X. Sun, Enhancement of the SO2 resistance of Mn/TiO2 SCR catalyst by Eu modification: a mechanism study, Fuel, 2018, 223, 385–393 CrossRef CAS.
- J.-W. Shi, Y. Wang, R. Duan, C. Gao, B. Wang, C. He and C. Niu, The synergistic effects between Ce and Cu in CuyCe1–yW5Ox catalysts for enhanced NH3-SCR of NOx and SO2 tolerance, Catal. Sci. Technol., 2019, 9, 718–730 RSC.
- H. Wang, P. Ning, Y. Zhang, Y. Ma, J. Wang, L. Wang and Q. Zhang, Highly efficient WO3-FeOx catalysts synthesized using a novel solvent-free method for NH3-SCR, J. Hazard. Mater., 2020, 388, 121812 CrossRef CAS.
- Y. Xin, N. Zhang, Q. Li, Z. Zhang, X. Cao, L. Zheng, Y. Zeng and J. A. Anderson, Active Site Identification and Modification of Electronic States by Atomic-Scale Doping To Enhance Oxide Catalyst Innovation, ACS Catal., 2018, 8, 1399–1404 CrossRef CAS.
- Z. Liu, H. Su, B. Chen, J. Li and S. I. Woo, Activity enhancement of WO3 modified Fe2O3 catalyst for the selective catalytic reduction of NOx by NH3, Chem. Eng. J., 2016, 299, 255–262 CrossRef CAS.
- J. Chen, Y. Chen, M. Zhou, Z. Huang, J. Gao, Z. Ma, J. Chen and X. Tang, Enhanced Performance of Ceria-Based NOx Reduction Catalysts by Optimal Support Effect, Environ. Sci. Technol., 2017, 51, 473–478 CrossRef CAS.
- W. Qu, X. Liu, J. Chen, Y. Dong, X. Tang and Y. Chen, Single-atom catalysts reveal the dinuclear characteristic of active sites in NO selective reduction with NH3, Nat. Commun., 2020, 11, 1532 CrossRef CAS.
|
This journal is © The Royal Society of Chemistry 2020 |