DOI:
10.1039/D0RA05057A
(Paper)
RSC Adv., 2020,
10, 29627-29632
Extreme enhancement of secondary chirality through coordination-driven steric changes of terpyridyl ligand in glutamide-based molecular gels†
Received
8th June 2020
, Accepted 3rd August 2020
First published on 10th August 2020
Abstract
Aggregation-induced chirality is potentially useful in sensor technology applications. Herein we show extreme enhancement of secondary chirality through coordination-driven steric changes of terpyridyl ligand in molecular gels. The secondary chirality reflecting on enhancement of chiral signals (i.e., circular dichroism (CD) and circularly polarised luminescence (CPL)) of the molecular gels formed from glutamide-attached terpyridine (G-tpy) is extremely enhanced by the coordination of its terpyridyl groups to metal ions such as Cu2+, Zn2+ and Ru2+, which is due to dramatic changes in the stacked structure of the chromophore groups through the formation of metal ion complex. Metal-free terpyridine exists in a non-planar geometry, which suppress π–π stacking interactions among aggregates. The planarity of the terpyridyl group is improved through metal-ion complexation, which induces the metal-ion-coordinated terpyridyl groups to stack. The thermal stabilities of the CD signals are strongly affected by the metal-ion species. CPL signal is generated in the molecular gel formed from G-tpy–Zn2+ complex accompanied by chelation-enhanced fluorescence. It is expected that large and sensitive coordination-driven secondary chirality signals (CD and CPL) are useful for sensing guest molecules and the surrounding environment.
Introduction
Organic ligands can be powerful responsive chromophoric tools for metal-ion complexes, as their absorption and emission properties are effectively changed through coordination.1 In sensors, such changes can be used to detect the nature of the surrounding environment, including temperature, pH, and additives present. A self-assembling system can amplify spectroscopic signals due to dramatic changes in its structural ordering.2 We reported molecular gel-forming chiral glutamide derivatives with organic ligands.3 The glutamide unit acts as a molecular tool that chirally orders the chromophoric groups, thereby inducing circularly polarised signals;4 coordination–complexation with metal ions also induces remarkable changes in chiroptical properties.5 The tuneable and sensitive chiroptical properties of these glutamide-attached chromophores (luminophores), such as circular dichroism (CD) and circularly polarised luminescence (CPL), are attractive features. Such chiroptical properties based on self-assembling system are recognized as “supramolecular chirality”.6 Terpyridyl ligands are broadly used in coordination chemistry because metal complexation strongly enhances its emission signals.7 Emission enhancement from the terpyridyl group is based on its rigid geometry, with the emission wavelength strongly affected by factors that include the kind of metal ion and the coordination structure. Therefore, various terpyridine complexes with unique luminescence properties are potentially useful in a number of applications, such as chemo-sensing, photocatalysis,8 detecting biological activity through DNA intercalation9 and covalent binding to biomolecules, with antitumor, radiotherapy, antiprotozoal agents, and protein probes as possible applications.10,11 Herein, we report that the secondary chirality of glutamide-attached terpyridine (G-tpy, Fig. 1a) self-assemblies arises through coordination with metal ions, which also effectively enhances optical activity. The strategy of this work is schematically illustrated in Fig. 1b.
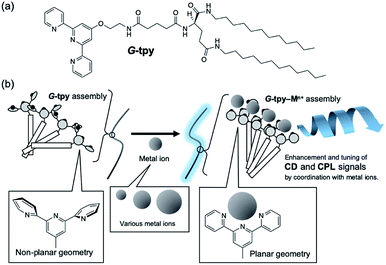 |
| Fig. 1 (a) Chemical structure of the glutamide-attached terpyridine (G-tpy), and (b) schematic illustration of the strategy of this work. | |
Results and discussion
Gelation property of G-tpy
G-tpy was synthesised by the amide coupling reaction of aminopropyl oxy terpyridine11 with double-alkylated glutamide12 using diethyl phosphoryl cyanide as a coupling reagent. The terpyridyl ligand was connected to the self-assembling molecular tool (G) via a flexible ether linker. The solubility of G-tpy was examined in a variety of organic solvents and their mixtures (Table S1†). Since G-tpy formed an organogel in a 9
:
1 mixture of cyclohexane–ethanol at ambient temperature (Fig. S1†), this mixture was chosen as the solvent for investigating G-tpy self-assembly in the absence and presence of metal ions.
Effect of metal ions on the secondary chirality of G-tpy
Absorption spectra of chiral ligand G-tpy and their metal complexes with Cu2+, Zn2+ and Ru3+ in the mixed solvent of cyclohexane and ethanol (9
:
1) were investigated. Two absorption bands were recorded at about 240 and 280 nm from π–π* intraligand charge transfer transition of G-tpy.13 The peaks red shifted to 326 nm, 316 nm and 395 nm by addition of Cu2+, Zn2+ and Ru3+ respectively (Fig. S2†), indicating that the formation of metal ion-coordinated terpyridyl group. This is associated due to the metal to ligand charge transfer transition. Similar spectral changes have been reported for the terpyridine derivatives through the complexation with metal ions.14 The absorption of each system at 320 nm as a function of metal-ion concentration is shown in Fig. S2d,† which reveals that G-tpy self-assembles form 2
:
1 complexes with the metal ions examined in this study. Strong circular dichroism (CD) signals are induced around the absorption band of the terpyridyl moiety by the addition of metal ions at concentration ratios of 2
:
1 and 1
:
1 (Fig. S3†). In this study we spectroscopically and microscopically examined G-tpy solutions with 1.0 molar equivalent of metal ions ([G-tpy]/[metal ion] = 1
:
1). CD enhancement is generally observed in self-assembled G molecules that contain chromophores; this phenomenon is referred to as “secondary chirality” and is based on the supramolecular chirality of the chromophores in the self-assembly. For instance, a G-attached pyrene as the chromophore (G-pyr,3c Fig. S4†) shows a comparably large CD signal around its absorption band; G-pyr: [θ]325 = −4.1 × 105 cm2 dmol−1 L−1, [θ]355 = 10.7 × 105 cm2 dmol−1 L−1. In comparison, almost no CD signals are observed for G-tpy in the absence of metal ions, which is due to the non-planar geometry of the terpyridyl moiety that prevents the spatial chiral arrangement. Interestingly the spectral pattern and the CD intensity of G-tpy are strongly affected by the metal-ion species (Fig. 2a). The addition of 0.5 molar equivalents of Cu2+ induces positive and negative CD signals at around 323 and 276 nm, respectively. A similar CD pattern is observed for G-tpy in the presence of Ru3+, but with extremely large molecular ellipticities. In comparison, the opposite CD pattern is observed for G-tpy complexed with Zn2+, with negative and positive signals at around 291 and 312 nm respectively. The molecular ellipticities of the CD signals obtained from G-tpy in the presence of Zn2+ and Ru3+ are of the order of 105, which is relatively large. Similar changes have been reported for organic–ligand-functionalised G through complex formation with metal ions. For instance, the CD signals of G-attached isoquinoline (G-iq, Fig. S4†) shows induced CD signals at 258 and 290 nm, and these signals change upon the addition of metal ions, such as Cu2+, Zn2+ and Co2+.5a The spectral patterns of G-iq in the presence and absence of a metal ion are completely different and depend on the metal-ion species. For instance, Cu2+ induces a redshift accompanied by a sign-reversed CD signal from the G-iq. The observed intensities of the CD signals without and with Cu2+ are similar. In comparison, Co2+ reduces the intensities of the CD signals of G-iq and the geometry of the isoquinolyl group is almost unchanged after coordination with the metal ion.
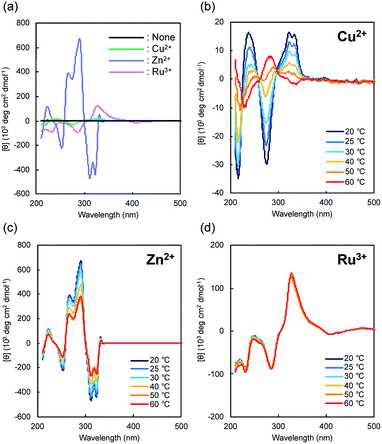 |
| Fig. 2 (a) CD spectra of G-tpy without and with metal ions. (b–d) Temperature dependencies of CD spectra with metal ions. [G-tpy] = 0.5 mM, [metal ion] = 0.5 mM. | |
As discussed above, remarkable CD-signal enhancements from G-tpy are observed after coordination to a metal ion, which are due to dramatic changes in the stacked structure of the chromophore groups through complexation to the metal ion. Metal-free terpyridine exists in a non-planar geometry,15 which suppress π–π stacking interactions among aggregates. The planarity of the terpyridyl group is improved through metal-ion complexation, which induces the metal-ion-coordinated terpyridyl groups to stack. The single-crystal X-ray diffraction analysis has been applied to confirm the steric geometry of pyridine rings in a crystal state of terpyridyl derivatives without and with transition metal ions such as Fe3+,16a Pd2+,16b Co2+,16c and Zn2+.16c In most of the cases, the free terpyridine form a transoid (trans–trans) arrangement,17 whereas the complex become in a square planar geometry after coordination with metal ion. It is important that the planarity of terpyridine–metal ion complex depend on the parameters of metal ions such as size and the coordination number.16 1H NMR spectroscopies of G-tpy in a mixture of cyclohexane-d12 and ethanol-d6 (9
:
1) revealed that the peaks of terpyridyl group were broadened by addition of equivalent of metal ions (Fig. S5†). It was considered that the molecular orientation of G-tpy changed dramatically by coordination with metal ions, however the geometric changes of the pyridyl group could not be confirmed by the 2D NMR. Significant CD-signal enhancement is the result of the spatial chiral arrangement of the planar metal-ion-coordinated terpyridyl group of G-tpy. Since no low energy absorption bands are observed, ligand-to-metal charge transfer appears not to occur.18 Interestingly the thermal responsiveness of the induced CD signals of G-tpy depends on the metal-ion species. Fig. 2 shows the CD spectra of G-tpy–Cu2+ (b), G-tpy–Zn2+ (c), G-tpy–Ru3+ (d) over a wide range of temperatures (20–60 °C). The G-tpy–Cu2+ complex shows CD signals that sign-reverse upon heating, from positive ([θ]323 = 16.4 × 103 deg cm2 dmol−1) to negative ([θ]323 = −0.5 × 103 deg cm2 dmol−1) at 323 nm, and from negative ([θ]276 = −29.8 × 103 deg cm2 dmol−1) to positive ([θ]276 = 7.0 × 103 deg cm2 dmol−1) at 276 nm. Similar CD-inversion trends were reported for complexes of terpyridyl-based ligands connecting alanine moieties, in which chirality could be controlled by the relative proportion of the terpyridyl derivative and its Pt complex.19 The induced CD signals are somewhat more thermally stable in G-tpy through complexation with Zn2+ and Ru3+; in G-tpy–Ru3+ they showed almost no change in the 20–60 °C temperature range.
Differential scanning calorimetry (DSC) was used to observe the gel-to-sol phase transitions of G-tpy in the absence and presence of metal ions. Because of the small enthalpies associated with phase transitions, somewhat higher concentrations (10 mM in a 9
:
1 mixture of cyclohexane–ethanol) of G-tpy were used. As shown in Fig. S6,† a remarkably small endothermic peak (ΔH = 0.5 kJ mol−1) with a maximum at 65 °C was observed for the G-tpy solution devoid of metal ions. In comparison, lower endothermic peaks (38 °C and 51 °C) were observed for G-tpy with 0.5 equivalents of Cu2+ and Zn2+, respectively (G-tpy precipitates from solution upon addition of 0.5 equivalents of Ru3+), and was accompanied by increases in the phase-transition enthalpy (ΔH = 1.08 and 4.07 kJ mol−1, respectively). These results indicate that coordination induces the rearrangement of the molecular orientation of G-tpy accompanied with chiral stacking of terpyridyl groups. The heat sensitivity of the ordering structure of G-tpy is depend on the metal ion species, particularly the G-tpy–Cu2+ complex is sensitive to heat.
Aggregation morphology of G-tpy molecular gel with metal ion
Rearrangement of the molecular orientation through the formation of a metal-ion complex is often accompanied by a morphological transformation. The G-iq formed nano-fibrillar aggregates in 9
:
1 cyclohexane–ethanol solution and transformed into fragmented ribbons and tape-like structures in the presence of Co2+ and Cu2+, respectively.5a G-tpy also formed 7 nm diameter nano-fibrous aggregates, and showed no drastic changes in morphological features upon addition of 1.0 equivalents of Cu2+, Zn2+, or Ru3+ (Fig. 3), irrespective of the remarkable changes observed by CD spectroscopy. It was confirmed that the fibrous aggregates of G-tpy–Zn2+ complex bundled into a larger aggregate. Similar bundled structure is often observed in the self-assemblies, which induce the 3 dimensional structure to form gel. Further investigates are needed to clarify the relevance of aggregation structure and exceptional large CD and fluorescence signals in G-tpy–Zn2+ complex.
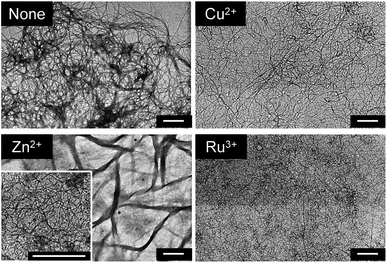 |
| Fig. 3 TEM images of G-tpy without and with metal ions. [G-tpy] = 0.5 mM, [metal ion] = 0.5 mM. The scale bars indicate 500 nm. | |
Coordination-induced circularly polarized luminescence
The blue coloured emission from G-tpy under UV light (365 nm) is affected by the addition of the metal ion; the emission from self-assembled G-tpy is quenched by Cu2+ and Ru3+, but is enhanced by Zn2+ (Fig. 4a). Fluorescence spectra acquired by excitation at 320 nm confirm that the intensity of the fluorescence emitted by G-tpy increased significantly upon addition of Zn2+ (Fig. 4b) and was almost saturated in the presence of 0.5 equivalents of Zn2+, which indicates that fluorescence is also enhanced through the formation of a 1
:
2 complex with Zn2+ (Fig. S7†). Fluorescence signal is remarkably decreased when G-tpy self-assembles with equimolar of Cu2+ or Ru3+ (Fig. 4b). Similar chelation-enhanced fluorescence (CHEF) and chelation-quenched fluorescence (CHQF) have been reported for phenylenevinylene terpyridine chelated with Cd2+ and Zn2+, in which the planarity of the terpyridyl moiety influences emission behavior.20 The complex formed between the terpyridyl group and each metal ion is expected to be complicated due to perturbations from the highly oriented structure of the G moiety. Further investigations are needed in order to clarify the CHEF and CHQF mechanisms involved in G-tpy self-assembly; however, such phenomena are useful for sensor applications despite the absence of mechanistic details. An extremely enhanced CD signal as well as fluorescence emission was observed for the G-tpy–Zn2+ self-assembled structure; therefore G-tpy–Zn2+ was examined by CPL spectroscopy. The spectra in Fig. 5 reveals a CPL signal at around the emission wavelength of G-tpy–Zn2+, in which the CPL and fluorescence intensities are changed simultaneously depending on the excitation wavelength. The CPL dissymmetry factor (|glum|) of G-tpy–Zn2+ (Ex. = 310 nm) was calculated to be 1.7 × 10−3 (at 364.5 nm), which is not surprisingly large and commonly obtained for G-attached chromophores.4 Almost no CPL signal was observed for G-tpy alone, nor its metal ion complexes with Cu2+ or Ru3+ (Fig. S8†).
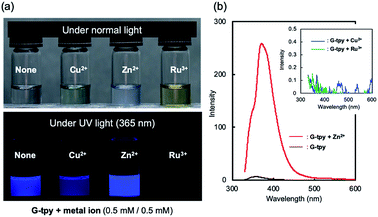 |
| Fig. 4 (a) Photographic images of G-tpy solutions without and with various metal ions under normal and UV light (365 nm). (b) Fluorescence spectra of G-tpy in the presence of Zn2+, Cu2+, and Ru3+. Solvent: cyclohexane–ethanol (9 : 1). [G-tpy] = 0.5 mM, [metal ion] = 0.5 mM. | |
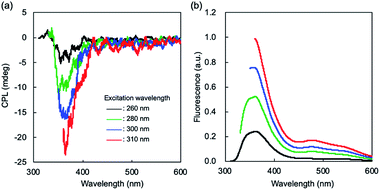 |
| Fig. 5 (a) CPL and (b) fluorescence spectra of G-tpy solutions without and with Zn2+. [G-tpy] = 0.5 mM. [Zn2+] = 0.5 mM. Solvent: cyclohexane–ethanol (9 : 1). | |
Experimental
Synthesis of G-tpy
Materials. All chemicals used for synthesis of G-tpy in this study were of analytical grade and were used as received without any further purification.
Synthetic procedure of G-tpy. The synthetic scheme for G-tpy is depicted in Fig. S9.† N1-4-carboxybutanoyl-N2,N3-didodecyl-L-glutamide (G-COOH) was synthesized according to our previous report,21 and 4′-chloro-2,2′:6′,2′′-terpyridine (tpy-NH2) was synthesized according to the literature.11 The details of synthesis are described in the ESI† and the chemical structures were characterized by elemental analysis (Micro Coder JM10, J-Science Lab Co.,Ltd., Kyoto, Japan), FT-IR (FT/IR4100 spectrophotometer, JASCO Co., Tokyo, Japan) and 1H NMR (JNM-ECZ400R NMR spectrometer, JEOL Ltd., Tokyo, Japan) spectroscopies. G-COOH (0.40 g, 0.67 mmol), tpy-NH2 (0.23 g 0.79 mmol), triethylamine (TEA, 2.53 g 3.48 mmol) and diethyl cyanophosphonate (DEPC, 0.45 mL 2.88 mmol) were dissolved in chloroform (200 mL) and the mixture was stirred for 1 hour in the ice water. Then the reaction mixture was stirred for 1 day in the room temperature. The reaction mixture was washed with 0.3 N HCl (3×), 1 N NaOH (3×) and water (3×). The chloroform solution was dried over Na2SO4 and the solvent was removed. After it was recrystallized by methanol to give a white powder: yield 464 mg (80%).
Identification of G-tpy. Mp 192.0–196.0 °C. IR spectrum (KBr): 3292, 2920, 2850, 1637, 1556, 1204 cm−1. 1H NMR (400 MHz, CDCl3, TMS, 25 °C): δ 0.86–0.90 (t, 6H, –CH3), 1.25–1.30 (m, 36H, –(CH2)9–), 1.45–1.52 (m, 4H, –NHCH2CH2–), 1.90–2.01 (m, 2H, –C*HCH2–) 1.97–2.01 (t, 2H, –C*HNHC(
O)CH2CH2–), 2.30–2.39 (m, 6H, –CH2CH2CH2C(
O)–) 3.15–3.25 (dt, 4H, –CH2–) 3.66–3.86 (m, 2H, –C*H NHC(
O)CH2CH2CH2C(
O)NHCH2–) 4.32–4.50 (m, 3H, –C*HNHC(
O)CH2CH2CH2C(
O)NH CH2CH2–) 6.10–6.15 (t, 1H, –NH–) 6.63–6.72 (t, 1H, –NH–) 6.89–6.97 (d, 2H, –NH–) 7.32–7.35 (t, 2H, H5, 5′′) 7.83–7.88 (t, 2H, H4, 4′′) 8.03–8.05 (s, 2H, H3′, 5′) 8.61–8.63 (d, 2H, H3, 3′′) 8.68–8.70 (d, 2H, H6, 6′′). Anal. calcd for C34H65N3O5: C, 70.4; H, 9.2; N, 11.3. Found: C, 70.0; H, 9.7; N, 11.3.
Methods
Preparation of the G-tpy solution without and with metal ions. G-tpy was dissolved in organic solvent at 70 °C for 5 min, and cooled at ambient temperature. The gelation property of G-tpy was confirmed with various organic solvents listed in Table S1† at the concentration of 5 mM at 10 and 70 °C. The spectroscopic measurements, microscopic observations and thermal analysis of G-tpy aggregates without and with metal ions were carried out in the mixed solvent of cyclohexane and ethanol (9
:
1). The ethanol solution of metal ion (10 μL) was added to the cyclohexane–ethanol solution of G-tpy (1 mL), and the mixture was heated at 70 °C for 5 min, and cooled at 10 °C for 24 h.
Spectroscopic measurements. All spectroscopic measurements were carried out in a quartz-cell using the spectrophotometric grade solvents. UV-vis, fluorescence, CD and CPL spectroscopies of G-tpy solutions without and with metal ions were measured using V-560, FL-6500, J-725 and CPL-300 (JASCO Co., Tokyo, Japan) respectively.
Microscopic observations. The G-tpy solution was dropped on the carbon-deposited poly(vinyl formal)-coated copper grid and dried at 25 °C. Uranyl acetate solution was used for staining G-tpy aggregates, but no staining reagent was use for G-tpy–metal ion complexes. Transmission electron microscopic observations were performed with JEM-1400Plus (JEOL Ltd., Tokyo, Japan).
Thermal analysis. The G-tpy solution was put in 70 μL Ag cell and sealed tightly. Differential scanning calorimetry was performed using DSC thermal analysis system (DSC7000X, Hitachi High-Tech Science Co., Tokyo, Japan) under nitrogen atmosphere.
Conclusions
In summary, the secondary chirality of G-tpy is extremely enhanced by the coordination of its terpyridyl groups to metal ions. The induced CD signals of G-tpy are significantly increased, and the thermal stabilities of the CD signals are strongly affected by the metal-ion species, and CPL signal is generated accompanied by CHEF through the coordination with Zn2+. A large and sensitive CD and CPL signal are useful for sensing guest molecules and the surrounding environment. We reported that G-attached porphyrine (G-por, Fig. S4†) self-assembled with a metal ion can detect pyridine derivatives with various substituents through complexation to the empty site of the metal complex.5d We expect that aggregation-induced chiral signals are potentially applicable to sensor technology because they are responsive to multiple stimuli, such as metal ions and temperature. We are currently systematically studying the effects of various metal ions in order to understand the effects of the steric nature of the terpyridyl structure and the orientation of the G-tpy structure on enhancing and quenching coordination-driven secondary chirality. Further studies are needed to discuss on the remarkable enhancement of chiroptical properties of G-tpy through the coordination with metal ions with nonlinear optical properties such as hyperpolarizability and dipole moment.22
Conflicts of interest
There are no conflicts to declare.
Acknowledgements
This work is supported by Bilateral Joint Research Projects supported by the Japan Society for the Promotion of Science (JSPS), and Fund for the Promotion of Joint International Research by JSPS.
Notes and references
-
(a) D. R. McMillin and J. Moore, Coord. Chem. Rev., 2002, 229, 113 CrossRef CAS;
(b) U. S. Schubert, H. Hofmeier and G. R. Newkome, Modern Terpyridine Chemistry, WILEY-VCH Verlag GmbH& Co. KGaA, 2006 CrossRef.
- I. Eryazici, C. N. Moorefield and G. R. Newkome, Chem. Rev., 2008, 108, 1834 CrossRef CAS PubMed.
-
(a) M. Takafuji, H. Ihara, C. Hirayama, H. Hachisako and K. Yamada, Liq. Cryst., 1995, 18, 97 CrossRef CAS;
(b) H. Ihara, H. Hachisako, C. Hirayama and K. Yamada, J. Chem. Soc., Chem. Commun., 1992, 17, 1244 RSC;
(c) T. Sagawa, S. Fukugawa, T. Yamada and H. Ihara, Langmuir, 2002, 18, 7223 CrossRef CAS;
(d) M. Takafuji, A. Ishiodori, T. Yamada, T. Sakurai and H. Ihara, Chem. Commun., 2004, 1122 RSC.
-
(a) T. Goto, Y. Okazaki, M. Ueki, Y. Kuwahara, M. Takafuji, R. Oda and H. Ihara, Angew. Chem., Int. Ed., 2017, 56, 2989 CrossRef CAS PubMed;
(b) H. Jintoku, M. Kao, A. D. Guerzo, M. Dateki, Y. Yoshigashima, T. Masunaga, M. Takafuji and H. Ihara, J. Mater. Chem. C, 2015, 3, 5970 RSC;
(c) H. Oish, K. Yoshida, Y. Kuwahara, M. Takafuji, R. Oda and H. Ihara, J. Taiwan Inst. Chem. Eng., 2018, 92, 58 CrossRef.
-
(a) H. Ihara, T. Sakurai, T. Yamada, T. Hashimoto, M. Takafuji, T. Sagawa and H. Hachisako, Langmuir, 2002, 18, 7120 CrossRef CAS;
(b) H. Jintoku, T. Sagawa, M. Takafuji and H. Ihara, Chem.–Eur. J., 2011, 17, 11628 CrossRef CAS PubMed;
(c) H. Jintoku, T. Sagawa, T. Sawada, M. Takafuji, H. Hachisako and H. Ihara, Tetrahedron Lett., 2008, 49, 3987 CrossRef CAS;
(d) H. Jintoku, T. Sagawa, M. Takafuji and H. Ihara, Org. Biomol. Chem., 2009, 7, 2430 RSC.
-
(a) P. Duan, H. Cao, L. Zhang and M. Liu, Soft Matter, 2014, 10, 5428 RSC;
(b) T. Shimizu, M. Masuda and H. Minamikawa, Chem. Rev., 2005, 105, 1401 CrossRef CAS PubMed;
(c) M. Liu, L. Zhang and T. Wang, Chem. Rev., 2015, 115, 7304 CrossRef CAS PubMed.
-
(a) V. M. Suresh, A. De and T. K. Maji, Chem. Commun., 2015, 51, 14678 RSC;
(b) G. Barone, A. G. Gennaro, A. M. Giuliania and M. Giustini, RSC Adv., 2016, 6, 4936 RSC;
(c) P. Chen, Q. Li, S. Grindy and N. Holten-Andersen, J. Am. Chem. Soc., 2015, 137, 11590 CrossRef CAS PubMed.
-
(a) T. J. Wadas, Q. M. Wang, Y. J. Kim, C. Flaschenreim, T. N. Blanton and R. Eisenberg, J. Am. Chem. Soc., 2004, 126, 16841 CrossRef CAS PubMed;
(b) C. L. Exstrom, J. R. Sowa, C. A. Daws, D. Janzen, K. R. Mann, G. A. Moore and F. F. Stewart, Chem. Mater., 1995, 7, 15 CrossRef CAS;
(c) C. A. Daws, C. L. Exstrom, J. R. Sowa and K. R. Mann, Chem. Mater., 1997, 9, 363 CrossRef CAS;
(d) W. S. Tang, X. X. Lu, K. M. C. Wong and V. W. W. Yam, J. Mater. Chem., 2005, 15, 2714 RSC;
(e) P. W. Du, J. Schneider, P. Jarosz and R. Eisenberg, J. Am. Chem. Soc., 2006, 128, 7726 CrossRef CAS PubMed;
(f) M. Cortes, J. T. Carney, J. D. Oppenheimer, K. E. Downey and S. D. Cummings, Inorg. Chim. Acta, 2002, 333, 148 CrossRef CAS.
-
(a) K. W. Jennette, S. J. Lippard, G. A. Vassiliades and W. R. Bauer, Proc. Natl. Acad. Sci. U. S. A., 1974, 71, 3839 CrossRef CAS PubMed;
(b) S. J. Lippard, Acc. Chem. Res., 1978, 211 CrossRef CAS;
(c) W. D. McFadyen, L. P. G. Wakelin, I. A. G. Roos and B. L. Hillcoat, Biochem. J., 1987, 242, 177 CrossRef CAS PubMed;
(d) T. Ihara, H. Ohura, C. Shirahama, T. Furuzono, H. Shimada, H. Matsuura and Y. Kitamura, Nat. Commun., 2015, 6, 6640 CrossRef CAS PubMed.
-
(a) D. L. Ma, T. Y. T. Shum, F. Y. Zhang, C. M. Che and M. S. Yang, Chem. Commun., 2005, 46, 75 Search PubMed;
(b) C. Le Sech, K. Takakura, C. Saint-Marc, H. Frohlich, M. Charlier, N. Usami and K. Kobayashi, Radiat. Res., 2000, 153, 454 CrossRef CAS PubMed;
(c) S. Bonse, J. M. Richards, S. A. Ross, G. Lowe and R. L. Krauth-Siegel, J. Med. Chem., 2000, 43, 4812 CrossRef CAS PubMed;
(d) E. M. A. Ratilla and N. M. Kostić, J. Am. Chem. Soc., 1988, 110, 4427 CrossRef CAS.
- G. Maayan, B. Yoo and K. Kirshenbaum, Tetrahedron Lett., 2008, 49, 335 CrossRef CAS PubMed.
-
(a) Y. Kira, Y. Okazaki, T. Sawada, M. Takafuji and H. Ihara, Amino Acids, 2010, 39, 587 CrossRef CAS PubMed;
(b) V. Gopal, J. Xavier, M. Z. Kamal, S. Govindarajan, M. Takafuji, S. Soga, T. Ueno, H. Ihara and N. M. Rao, Bioconjugate Chem., 2011, 22, 2244 CrossRef CAS PubMed.
-
(a) S. Gama, I. Rodrigues, F. Marques, E. Palma, I. Correia, M. F. N. Carvalho, J. C. Pessoa, A. Cruz, S. Mendo, I. C. Santos, F. Mendes, I. Santos and A. Paulo, RSC Adv., 2014, 4, 61363 RSC;
(b) S. Kuyuldar, C. Burda and W. B. Connick, RSC Adv., 2019, 9, 21116 RSC.
- A. K. Pal, B. Larame-Milette and G. S. Hanan, Inorg. Chim. Acta, 2014, 418, 15 CrossRef CAS.
-
(a) S. Y. leung, K. M. Wong and V. W. Yam, Proc. Natl. Acad. Sci. U. S. A., 2016, 113, 2845 CrossRef CAS PubMed;
(b) S. Bhowmik, B. Ghosh and K. Rissanen, Org. Biomol. Chem., 2014, 12, 8836 RSC.
-
(a) E. C. Constable, G. Zhang, C. E. Housecroft and M. Neuburger, Inorg. Chem. Commun., 2010, 13, 878 CrossRef CAS;
(b) F. Darabi, H. Hadadzadeh, J. Simpson and A. Shahpiri, New J. Chem., 2016, 11, 9081 RSC;
(c) B. Whittle, E. L. Horwood, L. H. Rees, S. R. Batten, J. C. Jeffery and M. D. Ward, Polyhedron, 1998, 17, 373 CrossRef CAS.
-
(a) A. Anthonysamy, S. Balasubramanian, V. Shanmugaiah and N. Mathivanan, Dalton Trans., 2008, 2136 RSC;
(b) R. Fallahpour, M. Neubburger and M. Zehnder, New J. Chem., 1999, 23, 53 RSC.
- R. Ziessel, S. Diring and P. Retailleau, Dalton Trans., 2006, 3285 RSC.
- K. Kim, J. Kim, C. Moon, J. Liu, S. Lee, M. Choi, C. Feng and J. Jung, Angew. Chem., Int. Ed., 2019, 58, 11709 CrossRef CAS PubMed.
- Z. Zhang, C. Wang, Z. Zhang, Y. Luo, S. Sun and G. Zhang, Spectrochim. Acta, Part A, 2019, 209, 40 CrossRef CAS PubMed.
-
(a) H. Ihara, M. Takafuji, C. Hirayama and D. F. O'Brien, Langmuir, 1992, 8, 1548 CrossRef CAS;
(b) H. Hachisako, Y. Murata and H. Ihara, J. Chem. Soc., Perkin Trans. 1, 1999, 2, 2569 RSC;
(c) M. Takafuji, H. Ihara, C. Hirayama, H. Hachisako and K. Yamada, Liq. Cryst., 1995, 18, 97 CrossRef CAS.
-
(a) F. Tessore, D. Roberto, R. Ugo and M. Pizzotti, Inorg. Chem., 2005, 44, 8969 Search PubMed;
(b) S. K. Peter, C. Kaulen, A. Hoffman, W. Ogieglo, S. Karthauser, M. Homberger, S. Herrer-Pawlis and U. Simon, J. Phys. Chem. C, 2019, 123, 6537 CrossRef CAS.
Footnote |
† Electronic supplementary information (ESI) available. See DOI: 10.1039/d0ra05057a |
|
This journal is © The Royal Society of Chemistry 2020 |