DOI:
10.1039/D0RA04802J
(Paper)
RSC Adv., 2020,
10, 32723-32729
Experimental investigation on the evolution of structure and mechanical properties of basalt induced by microwave irradiation
Received
31st May 2020
, Accepted 22nd August 2020
First published on 3rd September 2020
Abstract
Demand is growing for explosive-free and high efficiency rock breakage systems for mining, petroleum and civil engineering applications. Microwave irradiation is becoming a promising technique to deal with rock breakage due to its high efficiency, controllability and environmental friendliness. The cylindrical basalt samples with diameter of 50 mm and height of 100 mm and semi-disc specimens with diameter of 50 mm and thickness of 25 mm were irradiated using microwave apparatus (2.45 GHz, 2 kW). The mechanical properties of microwaved basalt have been tested and the micro fractures were quantitatively analyzed. The structural evolution and mechanical properties of basalt between 100 °C and 400 °C are assessed through the morphology, mineral characteristics and mechanical performance. It is found that the main damage modes of microwaved basalt are intergranular and transgranular fractures. Intergranular fractures generated rapidly at 100 °C, while transgranular fractures generated above 200 °C. Statistically, the length density of fractures grows fastest at 100 °C, while the width of fractures grows fastest at 200 °C. The intergranular and transgranular fractures develop rapidly and intersect each other over 400 °C, which results in rock failure. The length density of the fractures is the main factor inducing the decrease of compressive strength and fracture toughness of basalt which decrease fastest at 100 °C. The elastic modulus decreases fastest at 200 °C, which is closely related to the width of fractures. The Poisson's ratio of basalt is significantly improved by microwaves, and is not only affected by fractures size, but also closely relates to fracture type and distribution.
1. Introduction
Rock breakage has become a significant consideration in underground excavations such as mining, petroleum and mineral exploration, civil engineering and space development. However, the current rock breaking technique has difficulties in optimizing the drilling efficiency and cost.1–4 In the past few decades, microwave irradiation has been a promising technique to deal with rock breakage for the advantages of its high efficiency, controllability and environmental friendliness.5,6 Theoretical and experimental investigations have indicated that the mechanical properties of rock decay significantly after microwave irradiation, and the uniaxial compressive strength, and point load strength of rock decrease rapidly with the increase of irradiation power.7–12 Furthermore, hard rocks such as basalt could be rapidly heated up enough to be broken by low irradiation power,13,14 while high-power but short-term and pulse irradiation could also cause rapid deterioration of the mechanical properties of hard rock.15–19
For a given rock type, it is essentially cracked by thermal stress, due to the difference in absorbing and converting capacities of minerals induces a non-uniform heating of rock exposed to microwave irradiation.20–25 Generally, higher irradiation power and longer irradiation time make the greater porosity and fractures density of the microwaved rock.26,27 Besides, numerical simulation of internal stress distribution shows that the maximum stress is usually not inside the minerals with the best microwave absorption, but at the interface between the high thermal expansion minerals and others.28,29 In addition, according to the simulation results, internal thermal stress of the microwaved rock is also affected by the mineral crystals, more uniform and smaller crystal grains cause lower peak stress.30,31 The current work of microwave irradiation on basalt is mostly focused on the influence of the power of microwave equipment. But the differences of sample size, shape and microwave equipment will dramatically affect the irradiation results even under same power. In fact, rock damage by microwave irradiation is mainly controlled by temperature. But to the best of our knowledge, it is still unclear how the microwave radiation temperature affects the structure and mechanical properties of the rock.
The main purpose of this study is to qualitatively investigate the relationship between structural evolution and mechanical properties of basalt with surface temperature based on the investigation of the changes of fracture morphology, mineral characteristics and mechanical performance by microwave. These observations provide guidance for determining the most energy efficient irradiation temperature parameter to promote the application of microwave in rock excavation engineering and mineral processing engineering.
2. Experiment procedure
2.1 Experimental material
The basalt is collected from the Mentougou Mine to the west of Beijing, China. The mineral compositions test of XRD characterizes that the basalt is mainly composed of feldspar and enstatite (Table 1).
Table 1 Compositions of basalt and material properties of minerals
Mineral |
Feldspar |
Enstatite |
Percentage |
69.0% |
31.0% |
Relative dielectric permittivity |
4.14 − j0.012 |
8.33 − j0.664 |
Coefficient of thermal expansion (1/K) |
3.6 × 10−6 |
1.2 × 10−5 |
According to the results of Electron Probe Micro Analyzer (Table 2), enstatite is with wrinkled surface and irregular shape, while feldspar is with smooth surface and scattered strip-shape (Fig. 1).
Table 2 Results of EPMA analysis of mineral compositions
Mineral |
Content (wt%) |
Na2O |
FeO |
MgO |
SiO2 |
Al2O3 |
CaO |
Feldspar |
4.01 |
0.70 |
0.02 |
53.46 |
28.21 |
11.67 |
Enstatite |
0.07 |
13.23 |
17.09 |
51.56 |
2.71 |
0.92 |
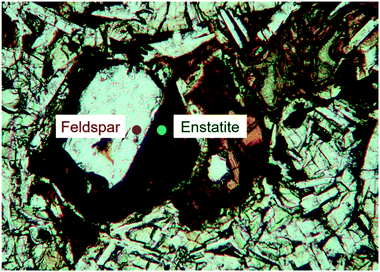 |
| Fig. 1 EPMA morphology of basalt. | |
Three kinds of samples are processed for morphology and mechanical property tests. Disc rock samples with diameter of 10 mm and height of 5 mm were adopted for the micromorphology observation. Cylindrical samples with diameter of 50 mm and height of 100 mm are used for uniaxial compression experiments, and semi-disc specimens with thickness of 25 mm are processed for the fracture toughness test (Fig. 2).
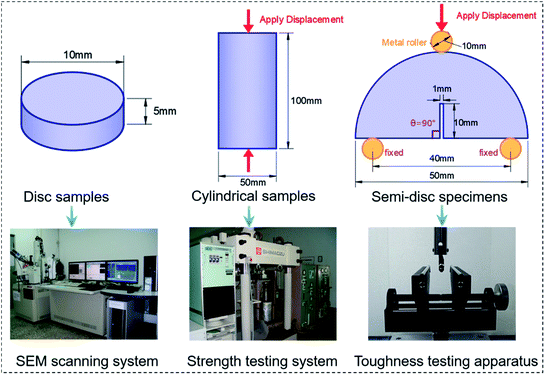 |
| Fig. 2 Preparation of experimental specimens. | |
2.2 Experiment procedure
Basalt samples were heated by an automatic temperature-controlled microwave apparatus Bgrimm CY-MU1400C-S (2.45 GHz, 2 kW) consists of a control system, temperature sensors, magnetron, waveguide and metal gauze (Fig. 3). The microwave cavity is with length of 50 cm, height of 50 cm and width of 30 cm. Microwave heating atmosphere is ordinary air. There is an infrared temperature measuring system in the equipment, which can measure and feedback the temperature of the rock in real time. Each group of samples was heated from normal temperature to 100 °C, 200 °C, 300 °C and 400 °C respectively with the same heating rate of 50 °C min−1 and then kept up for 5 minutes before cooling for morphology and mechanical property tests. The schematic diagram of the microwave heating path is shown in Fig. 4.
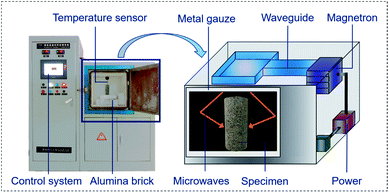 |
| Fig. 3 Schematic of the experimental design and microwave system. | |
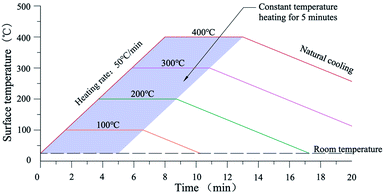 |
| Fig. 4 Schematic diagram of the microwave heating path. | |
The micro structure of basalt samples are observed by SEM (Shimadzu, JMS-7001F) and EPMA (GENESIS XM, EDAX), and the fractures are statistically analyzed by image processing in MATLAB. The uniaxial strength, elastic modulus and Poisson's ratio are measured by uniaxial test system (Shimadzu, YSSZ), fracture toughness is tested by the method of Type I static fracture toughness recommended by ISRM.
3. Results and discussion
3.1 Structural evolution of basalt by microwave irradiation
The micro-morphologies of basalt at different temperatures is demonstrated in Fig. 5. It is shown that fractures of basalt increases and different types of fractures appeared successively with the microwave temperature.
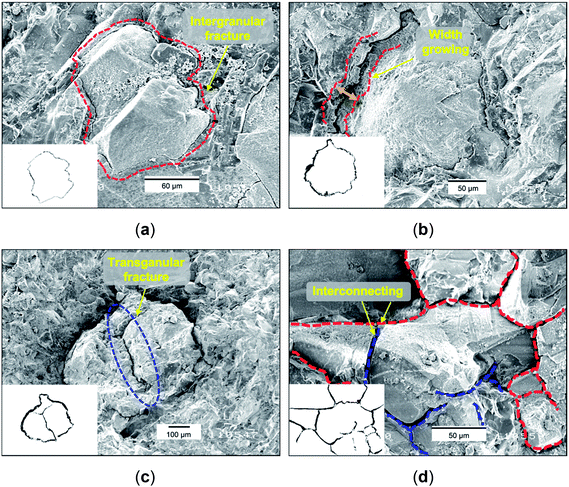 |
| Fig. 5 The SEM morphologies of irradiated basalt at different temperatures: (a) 100 °C; (b) 200 °C; (c) 300 °C; (d) 400 °C. | |
A large quantity of slender fractures appeared at 100 °C, and the fracture path basically propagates along the grain boundary of minerals (Fig. 5a). Moreover, it is shown that these fractures generally occur at the interface between feldspar and enstatite grains, namely intergranular fractures (Fig. 6). The dielectric losses of enstatite in basalt are higher than those of feldspar, so enstatite has a better ability to absorb microwave and would be heated up faster. In addition, the thermal expansivity of enstatite is higher than feldspar, and the thermal expansion of enstatite would be larger than feldspar. Thermal shear stress between the enstatite and feldspar grains would appear and increase with irradiation temperature, and intergranular fracture occurs when the thermal stress exceeds the bonding strength of the grains, due to the difference in dielectric properties and thermal parameters between different minerals.32
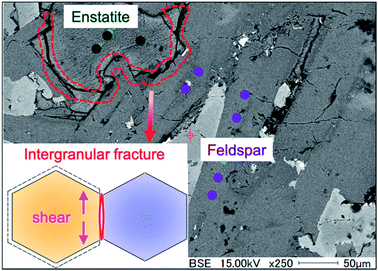 |
| Fig. 6 Schematic diagram of intergranular fracture in basalt by microwave irradiation. | |
Fig. 5b showed the width of intergranular fracture increases significantly at 200 °C, and the grain is almost enveloped by the intergranular fracture. The thermal stress between enstatite and feldspar further increased with temperature, which caused the width and length of the intergranular fractures rapidly expand.
The intergranular fractures increase slower at 300 °C. Meanwhile, the other type of fractures which penetrated into the mineral grains appears, namely transgranular fractures (Fig. 5c). It is analyzed that the grains continue to thermally expand and squeeze each other with irradiation temperature, and thermal compressive stress is generated between grains. The penetrated fracture inside the grain occurs when the thermal compressive stress reaches the breaking strength of the grain (Fig. 7). Although the transgranular fractures are less than that of intergranular fractures, they exert a interconnecting effect to the intergranular fractures.
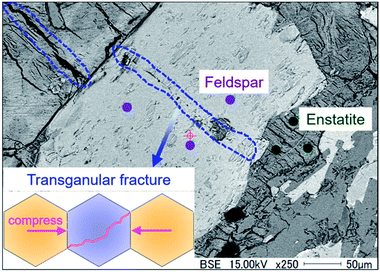 |
| Fig. 7 Schematic diagram of transgranular fracture in basalt by microwave irradiation. | |
At 400 °C, the size of two kinds of fractures increases continuously, and large quantities of fractures interconnect to form a complex fractures network (Fig. 5d). At this time, the rock is severely damaged, even breaks into fragments.
Furthermore, the variations of fracture density with surface temperature are obtained by image extraction and statistical techniques (Fig. 8). Both the length and width of the fractures increase with surface temperature, and the length density of the fractures grows fastest at 100 °C, while the width of the fractures grows fastest at 200 °C.
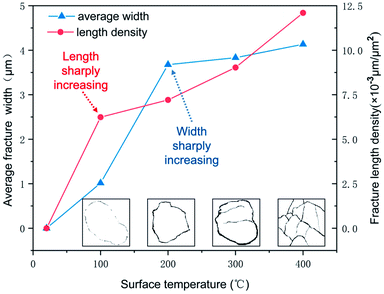 |
| Fig. 8 Effect of surface temperature on the thermal fractures of basalt. | |
3.2 Effects of microwave irradiation on mechanical properties of basalt
3.2.1 Effect of microwave irradiation on uniaxial compressive strength of basalt. The experimental results show that microwave irradiation exerts a significant decrease effect on the uniaxial compressive strength of basalt (Fig. 9). The uniaxial compressive strength decreases fastes at 100 °C, indicating that the variation of compressive strength is consistent with the fracture length density. This is because the fracture length density is the reflection of the total quantity of fractures, the more fractures inside of basalt, the lower compressive strength of basalt.
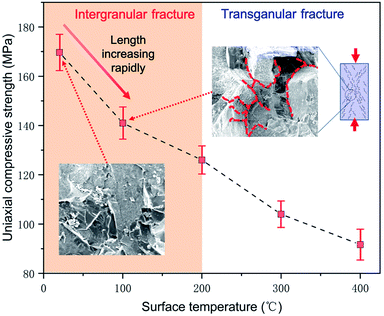 |
| Fig. 9 Variation of uniaxial compressive strength of basalt with surface temperature. | |
3.2.2 Effect of microwave irradiation on fracture toughness of basalt. Fig. 10 shows that the fracture toughness of basalt also decreases with surface temperature. The fracture toughness declines the fastest at 100 °C, but then declines gently. In fact, the fracture toughness of rock is very sensitive to internal fractures, the more fractures in the microwaved basalt, the lower its fracture toughness is. The results also suggest that the quantities of fractures, especially the length density of intergranular fractures, have remarkable influence on the fracture toughness of microwaved basalt.
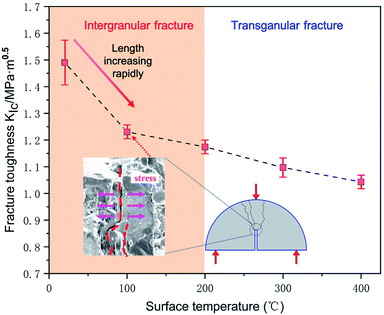 |
| Fig. 10 Variation of fracture toughness of basalt with surface temperature. | |
3.2.3 Effect of microwave irradiation on elastic modulus of basalt. Fig. 11 shows that the elastic modulus of basalt obviously decreases with basalt surface temperature. The elastic modulus of basalt decreases most sharply at 200 °C and then decreases slowly. The elastic modulus is closely related to the width of fractures in the rock. According the microstructure characteristics of the microwaved basalt, there are many intergranular fractures with the large width at 200 °C. So the intergranular fractures with larger width would be compressed gradually and the strain of the rock would be larger in the compressive test, causing the elastic modulus to decrease drastically. While above 200 °C, intergranular fractures increase slower and more transgranular fractures generated. But the quantities and size of transgranular fractures are relatively small, results in a limited degree of compression in the test, so the elastic modulus decreases slowly over 200 °C.
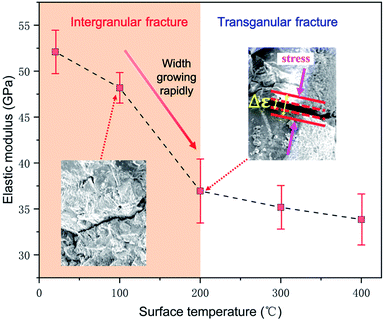 |
| Fig. 11 Variation of elastic modulus of basalt with surface temperature. | |
3.2.4 Effect of microwave irradiation on Poisson's ratio of basalt. The Poisson's ratio of basalt increases with the microwave irradiation temperature, but it increases significantly over 200 °C (Fig. 12). Transgranular fractures appear and intersect with the intergranular fractures above 200 °C, beginning to form complex propagations of fractures in all directions. Therefore, the mineral grains are more likely to be compressed and slip along the radial direction for lacking of radial restraint, resulting in a larger radial deformation, which causing the Poisson's ratio increases rapidly. So the Poisson's ratio of microwave irradiated basalt is not only affected by the quantity and size of fractures, but also closely related to the fracture type and distribution.
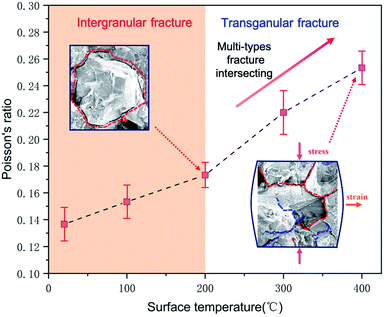 |
| Fig. 12 Variation of Poisson's ratio of basalt with surface temperature. | |
Precisely because of the significant difference in the microwave absorption performance of the two minerals, drastic temperature stresses are generated between the two minerals inside whole the rock concurrently, causing more micro-fractures at lower temperatures by microwave irradiation than ordinary heat method. According to the experimental results of this paper, the mechanical properties of basalt decline over half of the maximum at the irradiation temperature 400 °C by microwave, while the traditional heat method requires nearly 800 °C to achieve that.33–35 So rock breaking assisted by microwave could help increase the efficiency of rock excavation and prolong the life of cutting tools, reduce the engineering cost, especially for hard rock excavation.
Furthermore, for intergranular fracture does not need to break the crystal bond, the unit consumed energy of intergranular fracture would cause more micro cracks than that of transgranular fracture. And according the experimental results, the mechanical properties of the microwaved rock are mainly caused by intergranular cracks, so it could be inferred that 200–300 °C is the most economical microwave heating temperature when most intergranular fractures are formed.
4. Conclusions
The evolution of structure and mechanical property of microwave irradiated basalt is investigated based on changes of morphology, mineral characteristics and mechanical performance. In this study, some basic conclusions can be drawn as follows:
(1) Thermal stress is rapidly generated due to the differences of the absorption and thermal expansion among the internal components in basalt under the microwave irradiation. Obvious structural damage of the microwave irradiated basalt is observed, and the main damage modes of basalt are transgranular fractures and intergranular fractures, but the latter one dominated.
(2) Intergranular fractures rapidly generate at 100 °C, and increase continuously with basalt surface temperature. Transgranular fractures rapidly generate above 200 °C, then increase slightly with the surface temperature. The length density of the fractures grows fastest at 100 °C, while the width of the fractures grows fastest at 200 °C. The intergranular and transgranular fractures propagate rapidly and seriously intersect each other over 400 °C, which results in rock failure.
(3) Microwave irradiation exert a significant effect on the decrease of the uniaxial compressive strength and fracture toughness of basalt. The uniaxial compressive strength and fracture toughness decrease fast at 100 °C. Length density of the fractures is the main factor inducing the decrease of basalt strength and fracture toughness. The compressive strength and fracture toughness of microwaved basalt decreases with the increasing of the length density of fractures.
(4) The elastic modulus is closely related to the width of fractures in the rock, and the elastic modulus decreases fastest at 200 °C. The Poisson's ratio of basalt is significantly improved by microwave, and it increases rapidly over 200 °C. Poisson's ratio is not only affected by fractures size, but also closely related to fractures type and distribution.
Clear from these experiments and analyses are that the thermal stress caused by the difference in microwave absorption of minerals is the main reason for the fractures of the microwaved rock, and the length, width and intersection of the thermal cracks significantly affect the mechanical properties of rock. Further research of the quantitative relationship between the microwave energy absorbed by the rock and the thermal stress distribution is helpful to deepen the breakage mechanism of the microwaved rock and promote the application of microwave in rock excavation engineering.
Conflicts of interest
There are no conflicts to declare.
Acknowledgements
This work is supported by National Natural Science Foundation of China (No. 51674122 and 51874310). The support of the Yue Qi Young Scholar Project, China University of Mining & Technology, Beijing is also acknowledged.
References
- P. Flegner, J. Kačur, M. Durdán, M. Laciak, B. Stehlíková and M. Pástor, Significant damages of core diamond bits in the process of rocks drilling, Eng. Failure Anal., 2016, 59, 354–365 CrossRef.
- K. Miyazaki, T. Ohno, H. Karasawa, S. Takakura and A. Eko, Performance evaluation of polycrystalline diamond compact percussion bits through laboratory drilling tests, Int. J. Rock Mech. Min. Sci., 2016, 87, 1–7 CrossRef.
- X. Ren, H. Miao and Z. Peng, A review of cemented carbides for rock drilling: an old but still tough challenge in geo-engineering, Int. J. Refract. Met. Hard Mater., 2013, 39, 61–77 CrossRef CAS.
- H. Zhang, Z. Guan, Y. Liu, D. Liang and Y. Xu, A novel tool to improve the rate of penetration by transferring drilling string vibration energy to hydraulic energy, J. Pet. Sci. Eng., 2016, 146, 318–325 CrossRef CAS.
- S. W. Kingman and N. A. Rowson, Microwave treatment of minerals-a review, Miner. Eng., 1998, 11, 1081–1087 CrossRef CAS.
- W. Wei, Z. Shao, Y. Zhang, R. Qiao and J. Gao, Fundamentals and applications of microwave energy in rock and concrete processing – a review, Appl. Therm. Eng., 2019, 157, 113751 CrossRef.
- P. Hartlieb and B. Grafe, Experimental Study on Microwave Assisted Hard Rock Cutting of Granite, BHM Berg- Hüttenmännische Monatsh., 2017, 162, 77–81 CrossRef CAS.
- F. Hassani, P. M. Nekoovaght and N. Gharib, The influence of microwave irradiation on rocks for microwave-assisted underground excavation, J. Rock Mech. Geotech. Eng., 2016, 8, 1–15 CrossRef.
- H. Satish, J. Ouellet, V. Raghavan and P. Radziszewski, Investigating microwave assisted rock breakage for possible space mining applications, Min. Technol., 2013, 115, 34–40 CrossRef.
- T. Shepel, B. Grafe, P. Hartlieb, C. Drebenstedt and A. Malovyk, Evaluation of cutting forces in granite treated with microwaves on the basis of multiple linear regression analysis, Int. J. Rock Mech. Min. Sci., 2018, 107, 69–74 CrossRef.
- J. Zeng, Q. Hu, Y. Chen, X. Shu, S. Chen, L. He, H. Tang and X. Lu, Experimental investigation on structural evolution of granite at high temperature induced by microwave irradiation, Mineral. Petrol., 2019, 113, 745–754 CrossRef CAS.
- X. Li, S. Wang, Y. Xu, W. Yao, K. Xia and G. Lu, Effect of microwave irradiation on dynamic mode-I fracture parameters of Barre granite, Eng. Fract. Mech., 2020, 224, 106748 CrossRef.
- P. Hartlieb, F. Kuchar, P. Moser, H. Kargl and U. Restner, Reaction of different rock types to low-power (3.2 kW) microwave irradiation in a multimode cavity, Miner. Eng., 2018, 118, 37–51 CrossRef CAS.
- P. Hartlieb, M. Toifl, F. Kuchar, R. Meisels and T. Antretter, Thermo-physical properties of selected hard rocks and their relation to microwave-assisted comminution, Miner. Eng., 2016, 91, 34–41 CrossRef CAS.
- A. Y. Ali and S. M. Bradshaw, Bonded-particle modelling of microwave-induced damage in ore particles, Miner. Eng., 2010, 23, 780–790 CrossRef CAS.
- A. Y. Ali and S. M. Bradshaw, Confined particle bed breakage of microwave treated and untreated ores, Miner. Eng., 2011, 24, 1625–1630 CrossRef CAS.
- S. W. Kingman, K. Jackson, S. M. Bradshaw, N. A. Rowson and R. Greenwood, An investigation into the influence of microwave treatment on mineral ore comminution, Powder Technol., 2004, 146, 176–184 CrossRef CAS.
- V. Rizmanoski, The effect of microwave pretreatment on impact breakage of copper ore, Miner. Eng., 2011, 24, 1609–1618 CrossRef CAS.
- D. N. Whittles, S. W. Kingman and D. J. Reddish, Application of numerical modelling for prediction of the influence of power density on microwave-assisted breakage, Int. J. Miner. Process., 2003, 68, 71–91 CrossRef CAS.
- P. Hartlieb, M. Leindl, F. Kuchar, T. Antretter and P. Moser, Damage of basalt induced by microwave irradiation, Miner. Eng., 2012, 31, 82–89 CrossRef CAS.
- R. Meisels, M. Toifl, P. Hartlieb, F. Kuchar and T. Antretter, Microwave propagation and absorption and its thermo-mechanical consequences in heterogeneous rocks, Int. J. Miner. Process., 2015, 135, 40–51 CrossRef CAS.
- G. M. Lu, Y. H. Li, F. Hassani and X. W. Zhang, The influence of microwave irradiation on thermal properties of main rock-forming minerals, Appl. Therm. Eng., 2017, 112, 1523–1532 CrossRef CAS.
- D. A. Jones, S. W. Kingman, D. N. Whittles and I. S. Lowndes, Understanding microwave assisted breakage, Miner. Eng., 2005, 18, 659–669 CrossRef CAS.
- T. Monti, A. Tselev, O. Udoudo, I. N. Ivanov, C. Dodds and S. W. Kingman, High-resolution dielectric characterization of minerals: a step towards understanding the basic interactions between microwaves and rocks, Int. J. Miner. Process., 2016, 151, 8–21 CrossRef CAS.
- M. Omran, T. Fabritius, A. M. Elmahdy, N. A. Abdel-Khalek, M. El-Aref and A. E.-H. Elmanawi, XPS and FTIR spectroscopic study on microwave treated high phosphorus iron ore, Appl. Surf. Sci., 2015, 345, 127–140 CrossRef CAS.
- Y. D. Hong, B. Q. Lin, C. J. Zhu and H. Li, Effect of microwave irradiation on petrophysical characterization of coals, Appl. Therm. Eng., 2016, 102, 1109–1125 CrossRef CAS.
- K. Teimoori, F. Hassani, A. P. Sasmito and A. G. Madiseh, Experimental Investigations of Microwave Effects on Rock Breakage Using SEM Analysis, 17th International Conference on Microwave and High Frequency Heating, Valencia, Spain, 2019 Search PubMed.
- J. Li, R. B. Kaunda, S. Arora, P. Hartlieb and P. P. Nelson, Fully-coupled simulations of thermally-induced cracking in pegmatite due to microwave irradiation, J. Rock Mech. Geotech. Eng., 2019, 11, 242–250 CrossRef.
- M. Toifl, R. Meisels, P. Hartlieb, F. Kuchar and T. Antretter, 3D numerical study on microwave induced stresses in inhomogeneous hard rocks, Miner. Eng., 2016, 90, 29–42 CrossRef CAS.
- R. S. John, A. R. Batchelor, D. Ivanov, O. B. Udoudo, D. A. Jones, C. Dodds and S. W. Kingman, Understanding microwave induced sorting of porphyry copper ores, Miner. Eng., 2015, 84, 77–87 CrossRef CAS.
- Y. Wang and N. Djordjevic, Thermal stress FEM analysis of rock with microwave energy, Int. J. Miner. Process., 2014, 130, 74–81 CrossRef CAS.
- E. Jerby and Y. Shoshani, Localized microwave-heating (LMH) of basalt-Lava, dusty-plasma, and ball-lightning ejection by a “miniature volcano”, Sci. Rep., 2019, 9, 12954 CrossRef.
- H. Tian, T. Kempka, S. Yu and M. Ziegler, Mechanical Properties of Sandstones Exposed to High Temperature, Rock Mech. Rock Eng., 2015, 49, 321–327 CrossRef.
- L. N. Y. Wong, Y. Zhang and Z. Wu, Rock strengthening or weakening upon heating in the mild temperature range?, Eng. Geol., 2020, 272, 105619 CrossRef.
- L. Zhang, X. Mao and A. Lu, Experimental study on the mechanical properties of rocks at high temperature, Sci China Ser E, 2009, 52, 641–646 CrossRef.
|
This journal is © The Royal Society of Chemistry 2020 |
Click here to see how this site uses Cookies. View our privacy policy here.