DOI:
10.1039/D0RA04648E
(Paper)
RSC Adv., 2020,
10, 26594-26603
Collagen-functionalized electrospun smooth and porous polymeric scaffolds for the development of human skin-equivalent
Received
26th May 2020
, Accepted 7th July 2020
First published on 15th July 2020
Abstract
Electrospun polymer fibers have garnered substantial importance in regenerative medicine owing to their intrinsic 3D topography, extracellular matrix microenvironment, biochemical flexibility, and mechanical support. In particular, a material's nano-topography can have a significant effect on cellular responses, including adhesion, proliferation, differentiation, and migration. In this study, poly(L-lactic acid) (PLLA), a biodegradable polymer with excellent biocompatibility was electrospun into fibers with either smooth or porous topologies. The scaffolds were further modified and biofunctionalized with 0.01% and 0.1% collagen to enhance bioactivity and improve cellular interactions. Human keratinocytes (HaCaTs) and fibroblasts (human foreskin fibroblasts-HFF) were cultured on the scaffolds using a modified co-culture technique, where keratinocytes were grown on the dorsal plane for 5 days, followed by flipping, seeding with fibroblasts on the ventral plane and culturing for a further 5 days. Following this, cellular adhesion of the skin cells on both the unmodified and collagen-modified scaffolds (smooth and porous) was performed using scanning electron microscopy (SEM) and immunofluorescence. Distinct outcomes were observed with the unmodified smooth scaffolds showing superior cell adhesion than the porous scaffolds. Modification of the porous and smooth scaffolds with 0.1% collagen enhanced the adhesion and migration of both keratinocytes and fibroblasts to these scaffolds. Further, the collagen-modified scaffolds (both porous and smooth) produced confluent and uniform epidermal sheets of keratinocytes on one plane with healthy fibroblasts populated within the scaffolds. Thus, presenting a vast potential to serve as a self-organized skin substitute this may be a promising biomaterial for development as a dressing for patients suffering from wounds.
Introduction
Three-dimensional (3D) synthetic frameworks, often described as scaffolds, constructs, or matrices engineered using biomaterials, are indispensable for tissue engineering and regenerative medicine. Such scaffolds possess the ability to support cellular attachment, proliferation, and growth, which eventually can lead to new tissue formation. Several approaches have been established for the fabrication of biomaterial-based scaffolds. Most recently, fiber (nano/micron)-based scaffold systems have been explored as scaffolds for tissue engineering applications.1–3 Electrospun fibers with their nano/micron-sized fiber diameter, high porosity, and large surface area mimic the native physiological microenvironment of tissues. Electrospun fibers offer excellent mechanical stability and superior bioactivity on a macroscale level, whereas, at a nanoscale level, they offer biomimetic tissue microenvironments that support cellular attachment, proliferation, migration, and differentiation of cells.
Surface topography, surface chemistry, hydrophilicity, and functionality of nanomaterials are a few factors that support the use of scaffolds for tissue engineering applications.4–6 Owing to the rapid development of fabrication technologies, alterations in topography has garnered immense attention. The topography is important for cellular recognition of a tissues micro-environment and is important for the development of biomimetic materials used for the development of vascular stents, bone implants, and wound dressings.7 Surface topography also influences events including cellular adhesion, proliferation and migration, as well as affecting cell phenotypes.8 Surface chemistry is another significant factor that influences substantial variations in the bio-nano interface, owing to the selective binding of cells with functional groups on the surface of biomaterials.9,10 Several immobilization approaches have been exploited to improve nanomaterials' surface chemistry to assist protein adhesion to enhance and accelerate cellular adhesion. Bioactive molecules, when used in scaffolds, need to mimic the extracellular matrix (ECM), the supportive structure that surrounds the tissue cells, which is made up of many proteins and proteoglycans gathered in an ordered structure.11–14
Electrospun fibers of poly(L-lactic acid) (PLLA) and PLLA blends have been used in a wide variety of biomedical applications such as dressings for wounds, scaffolds for tissue (bone, nerve, skin) engineering, drug release and dental applications.7,15,16 PLLA is a biodegradable and biocompatible polymer that hydrolyzes to lactic acid, a biocompatible metabolic by-product.17,18 PLLA scaffolds offer large surface area, native extracellular properties, hydrophobicity, and superior mechanical strength. However, from a biochemical perspective, PLLA cannot be compared with other natural biomaterials due to its low hydrophilicity, which stems from its hydrocarbon backbone, limiting cell contact and spreading before the development of ECM.19 Also, PLLA does not have any recognizable biochemical binding sites or bioactive functional moieties, such as amine or thiol groups to conjugate with ECM components.20–22 Therefore, biomimetic approaches aimed at immobilization of native protein components of the ECM, such as collagen, gelatin or fibronectin, in synthetic polymer scaffolds, would be expected to enhance cell growth and cell-signaling similar to that observed in native tissues.19 One of the methods that has been used to immobilize collagen is gamma irradiation; however, when collagen is coupled to PLLA through gamma irradiation, degradation of PLLA with a reduction in its mechanical properties is observed.23 Other approaches, such as the use of crosslinkers, plasma treatment, aminolysis, hydrolysis, treatment with phosphorous pentachloride has also been reported as ways to modify the PLLA surface to attract biomolecules.24–27 In most of these approaches, the reactive group was not long-lasting, and hence the functionality was lost. In other studies, reactive groups were introduced, and proteins were attached to the PLLA scaffold using a three-step complex by grafting maleic anhydride, which was then reacted with diamines, followed by coupling to proteins.28,29 Alida et al. also grafted collagen onto PLLA using maleic anhydride, and scaffolds were subsequently fabricated by electrospinning. This approach led to the promotion of good cell adhesion.30 He et al. also demonstrated enhanced cell growth, proliferation, and attachment of human coronary artery endothelial cells on collagen-coated poly(L-lactic acid)-co-poly(3-caprolactone) nanofibers.31 In another recent study by Muniyandi et al., porous PLLA fibers were modified with ECM components, including collagen, fibronectin, gelatin, and poly(L-lysine).12 Adult human cardiac fibroblasts (AHCF) were subsequently cultured on the protein modified and unmodified porous fibers and showed cellular adhesion and proliferation regardless of the surface modifications.
Chronic, non-healing wounds affect the health of millions of people worldwide and pose a substantial clinical challenge that could be addressed by the development of improved dressings that promote tissue regeneration.32 Protein modified PLLA scaffolds are ideal candidates for the development of the next generation of wound dressings. As skin consists of several differentiated layers, a platform that permits the growth of different types of cells near to each other, at the same time and in distinct layers, would mimic the in vivo microenvironment leading to improved tissue repair. We herein report the design and feasibility of a modified co-culture system for keratinocytes and fibroblasts using electrospun scaffolds with different topographies (smooth and porous) in conjunction with surface modifications as a potential skin equivalent and wound dressing model. The biomaterials designated for supporting skin cell growth in the present study are synthetic polymer, poly(L-lactic acid) (PLLA)-based, and the surface is modified with collagen to mimic the native extracellular matrix of the skin. It was anticipated that the tissue-like construct would create a 3D organotypic culture, signifying epithelial differentiation, morphology, and proliferation similar to that of skin.
Experimental section
Fabrication of collagen modified smooth and porous electrospun nanofibers
Electrospun smooth poly(L-lactide) (PLLA) and porous scaffolds were fabricated as previously reported with slight modifications.12,33 For smooth fibers, PLLA, Mw of ∼80
000–100
000 (Polyscience Inc, Warrington, PA, USA) was dissolved in 1,1,1,3,3,3-hexafluoro-2-propanol (HFIP) (Fujifilm Wako Pure Chemical Corporation, Japan) to obtain a 14.5% w/v of PLLA solution, using magnetic stirring for 4 h. The solution was allowed to rest for another 4 h at room temperature (RT) (25 °C). PLLA fibers were fabricated using an electrospinner (Nanon-O1A MECC Co. Ltd. Fukuda, Japan). The electrospinning process was performed at RT, at a flow rate of 0.5 mL h−1 and at an applied voltage of 15 kV. A grounded plate that was placed at a distance of 12 cm from the tip of the needle was used as a collector. For fabricating porous electrospun PLLA scaffolds, 11.5% PLLA solution was prepared using a binary solvent system of chloroform
:
dimethyl formamide (9
:
1) and stirred for 24 h. The solution was further allowed to rest for another 4 h before the electrospinning process. The polymer solution was filled in a 10 mL syringe with a blunt-ended 18-gauge needle. Electrospinning was performed at a potential difference of 15 kV with a flow rate of 2 mL h−1. The grounded plate was placed at a distance of 12 cm from the tip of the needle and was used as the collector. The air humidity and temperature conditions during spinning process were about 40–50% and 25 °C, respectively. The mat from the collector was detached, dried to remove any residual solvents and stored for further characterization and studies.
To improve the surface functionality and compatibility of the smooth and porous PLLA scaffolds for cell culture, physical adsorption method of collagen via simple drop coating was performed. Collagen I (Sigma Life Science) solution at a concentration of 0.01% and 0.1% was used. Previous studies have used 0.01% collagen12 so this concentration was chosen alongside a 10-fold increase in collagen to maximize the effect. Pre-wetted electrospun nanofibers (smooth and porous) were treated with collagen solution and incubated at 37 °C for 30 minutes. After incubation, the solution was aspirated and conditioned with culture media for cell culture studies.
Characterization of nanofibers
Morphology of electrospun nanofibers was studied using a scanning electron microscope (SEM) (JSM-7400, JEOL Ltd., Tokyo, Japan). Aluminium foil was placed on the collector and electrospinning was processed for several minutes. The foil was loaded onto the SEM stub and coated with platinum using a platinum coater and observed by placing it in SEM. The SEM was operated at 5 kV range and the fiber diameters were measured based on SEM micrographs.
Cell culture
Human keratinocytes (HaCaT) from The American Type Culture Collection (ATCC), Virginia, USA and human foreskin fibroblasts (HFF) from CellBank Australia, New South Wales, Australia were maintained in Dulbecco's Minimum Essential Medium (DMEM) supplemented with 10% fetal bovine serum and penicillin–streptomycin antibiotic (pen strep-10
000 U mL−1).
Cell adhesion on PLLA mats
The PLLA scaffolds (smooth, porous) were sterilized by exposing them to UV light for 30 min and washed with sterilized PBS three times. Sterilized PLLA mats were conditioned with collagen and then with culture medium inside the biosafety cabinet prior to cell seeding. HFF cells (1 × 106) were seeded on respective PLLA mats (1 × 1 cm). The cell seeded mats were maintained for 5 days with media changed every 48 h.
Morphology of the cells on PLLA
The morphology of the adhered HFF cells after 5 days culture was evaluated by staining cytoskeletal actin filaments and cell nuclei. Cells were fixed with 4% paraformaldehyde, washed thrice in PBS and permeabilized using 0.1% Triton X-100 for 5 min in ice bath. Cells were rinsed with PBS and incubated for 20 min with Alexa Fluor 488 conjugated phalloidin (Thermo Fisher Scientific) followed by NucBlue (Thermo Fisher Scientific) and visualized using fluorescence microscopy (Olympus).
Co-culture of cells on unmodified and collagen modified scaffolds
A modified co-culture with keratinocytes (HaCaT) and fibroblasts (HFF) was performed with cells on either side of the scaffolds (unmodified-smooth and porous scaffolds without any treatment, 0.01% and 0.1% of collagen modified smooth and porous scaffold). A sterile stainless steel-O ring was placed over the scaffold and the center of the ring filled with DMEM inside a culture plate. HaCaTs were seeded at cell density of 1 × 106 cells at the center of the O-ring with the scaffold to prevent floating of scaffold in the culture media, and cultures were maintained in vitro for 5 days. Culture media was changed every 48 h. After 5 days of incubation, the PLLA mats with the HaCaTs were inverted, cell side down so that the cells were in contact with the medium. HFFs (1 × 106) were then seeded onto the other side of the PLLA mats. HFFs were cultured for another 5 days to adhere and spread. Culture medium was changed every 48 h.
SEM analysis of adhered cells in scaffold
The morphology of the adhered cells in co-cultured PLLA scaffolds was examined by scanning electron microscopy (SEM). The scaffolds were rinsed in PBS and fixed in 4% paraformaldehyde. Samples was dehydrated in increasing concentrations of ethanol (30%, 50%, 70%, 90% and 100%), dried with hexamethyldisilazane (HMDS) and gold coated. The samples were viewed under a SEM (Zeiss Merlin FEG SEM) and digital images were taken.
Immunostaining of HaCaTs and HFFs seeded on electrospun scaffolds
The scaffolds with HaCaTs and HFFs on either side (unmodified-smooth and porous, 0.1% collagen modified smooth and porous and negative control) were washed thrice with PBS, fixed with 4% paraformaldehyde for 20 min and rinsed with PBS, followed by permeabilization in ice cold MeOH, incubated in freezer for 15 min. This was followed by rinsing in PBS and samples were treated with Tween 20 (0.5%) in PBS 10 min and blocked with 3% goat serum overnight at 4 °C. The scaffolds were then washed with PBS and incubated with primary antibodies in blocking buffer overnight at 4 °C. The primary antibodies used in this study were mouse monoclonal antibody-Laminin V-P3E4 (1
:
200 Santa Cruz 13587 Lot#K15512), mouse monoclonal antibody-Keratin 14- LL002 (1
:
200 Neomarkers CA Lot 115P1308T), mouse monoclonal antibody-Vimentin V9 (1
:
200 Santa Cruz Lot #H2112) and rabbit polyclonal antibody-E Cadherin H-108 (1
:
200 Santa Cruz Lot#12012). After washing the samples with PBS, secondary antibodies-Alexa fluor 488 conjugated goat anti rabbit (Invitrogen-Lot 1981125) and Alexa fluor 568 conjugated goat anti mouse (Invitogen-Lot 2014175) (1
:
200) were added to scaffolds for 60 min at room temperature (25 °C) followed by nuclear stain NucBlue (Thermo Fisher Scientific). Negative controls were maintained without adding primary antibodies. The images were acquired using an Olympus fluorescent microscope.
Results
An electrospinning technique was used to fabricate PLLA smooth and porous scaffolds. Following this, the surface functionalization of the polymeric scaffolds was attained by a simple drop casting method with collagen I. The unmodified and collagen-modified electrospun smooth and porous PLLA scaffolds were then examined for the assembly of skin cells (HaCaTs and HFFs) into a skin equivalent.
Fabrication and characterization of electrospun scaffolds
Two different solvent systems were chosen for the fabrication of smooth and porous fibrous scaffolds. A single solvent system with HFIP was used for fabricating the smooth and uniform PLLA fibers. A binary solvent system containing chloroform and DMF was used for the fabrication of porous fibers.12,34 The choice of the solvent system significantly influenced the generation of pores in the fiber. A schematic representation of the electrospinning set-up for both smooth and porous fibers is presented in Fig. 1a.
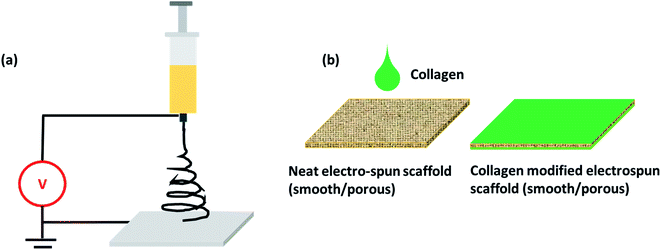 |
| Fig. 1 (a) Schematic of electrospinning set-up and (b) collagen functionalization on PLLA scaffolds. | |
SEM images revealed the smooth and porous surface morphologies of electrospun PLLA mats fabricated under different synthesis conditions (Fig. 2). Unmodified smooth PLLA fibers exhibited randomly distributed fibers with an average diameter in the range of 900–1200 nm (Fig. 2-i). However, unmodified porous fibers exhibited continuous structures and good interconnected porous architectures with fiber diameters ranging from 1000–1300 nm and pores in the range of 40–60 nm (Fig. 2-iv). Surface modification of the electrospun scaffolds was attained by drop-casting one of the major ECM component-collagen in two different ratios (0.01% and 0.1%) (Fig. 1b and 2-ii, iii, v and vi). Surface treatment with collagen slightly altered the morphology and porosity of both the smooth and porous fibers. The morphology of the smooth fibers after collagen treatment appeared rough and deposits of collagen as aggregates and ultra-fine fibrous structures (in the range of 50 nm) were visible from SEM micrographs. However, the aggregates and fibrous structures were comparatively fewer when a lower concentration of collagen (0.01%) was used for the modification. We observed a similar roughness in porous fibers and some of the pores were coated with collagen, again increasing the roughness, which we anticipated might provide extra grip and superior cellular attachment. Functionalization of the fibers (smooth and porous) with collagen was achieved using physical adsorption, which is a straightforward and extensively used strategy to immobilize proteins on surfaces. The SEM observations provide evidence for varied surface topology and surface chemistry of fabricated smooth and porous fibers, which is significant for cellular interaction.
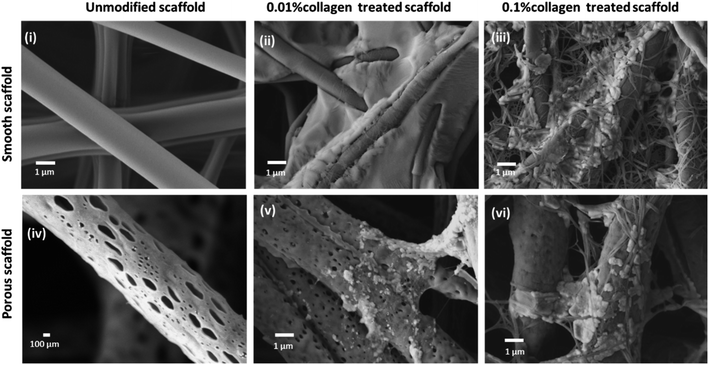 |
| Fig. 2 Morphological and structural characterization of unmodified and collagen modified electrospun PLLA scaffold. SEM micrographs depicting the morphological features of unmodified smooth and porous and collagen-modified smooth and porous electrospun scaffolds: (i–iii) SEM of unmodified smooth, 0.01% collagen-treated smooth scaffold and 0.1% collagen-treated smooth scaffold (scale bar = 1 μm); (iv–vi) SEM of unmodified porous (scale bar = 100 nm), 0.01% collagen-treated porous scaffold and 0.1% collagen treated porous scaffold (scale bar = 1 μm). | |
Cell adhesion on electrospun PLLA scaffolds
The behavior of individual cell types and cell–nanomaterial interactions in response to nanotopography has attracted attention owing to potential roles in events like wound healing, bone and neural regeneration. Herein, cultured HFFs, were added to the electrospun scaffolds (unmodified smooth and porous scaffolds, 0.01% collagen-treated smooth and porous scaffolds and 0.1% collagen-modified smooth and porous scaffolds) for 5 days and observed for cell adhesion. Actin cytoskeletal structures stained after 5 days in culture exhibited significant differences in the adherence pattern on scaffolds with different topography and surface chemistries. HFFs exhibited an elongated shape with a spread cytoplasm and cytoplasmic extensions that attached to the fibers of unmodified smooth scaffolds and an overall distribution of HFFs were observed on the surface of the smooth mat (Fig. 3a-iv). Although collagen modification supported the HFFs, the morphology of the cells was different when the concentration of collagen was increased. The cells exhibited spindle-like elongated pattern when seeded on smooth fibers modified with 0.01% collagen (Fig. 3a-v). HFFs were observed with extensively spread cytoplasm when grown on smooth mats modified with 0.1% collagen (Fig. 3a-vi). However, unmodified porous mats exhibited significantly different cellular morphology. The cells were not healthy, and the morphology was not retained as seen from SEM micrographs (Fig. 3a-i and c). The collagen modification improved the adhesion of the HFFs. We also speculate that collagen modification resulted in changes to the surface properties and development of ultra-fine fibers, which might also have supported cellular outgrowth. As the concentration of collagen was increased from 0.01% to 0.1% the cells regained their distinctive HFF morphology (Fig. 3a-ii and iii). We also performed nuclei counting which demonstrated the adherence pattern on porous mats vs. smooth mats. The number of nuclei were significantly lower in unmodified porous mats with the number increasing with increased concentration of collagen (Fig. 3b).
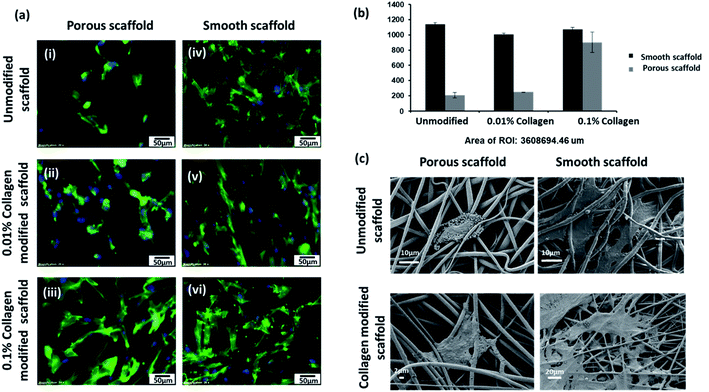 |
| Fig. 3 Adhesion of HFF cells in porous and smooth electrospun PLLA scaffold. (a) Fluorescent images (actin cytoskeleton stained) of HFF cells adhered to electrospun scaffolds (i) unmodified porous scaffold, (ii) 0.01% collagen treated porous scaffold and (iii) 0.1% collagen treated porous scaffold; (iv) unmodified smooth, (v) 0.01% collagen treated smooth scaffold and (vi) 0.1% collagen treated smooth scaffold (scale bar = 50 μm); (b) nuclear count of adhered HFF cells on unmodified porous and smooth and collagen modified smooth and porous with area of interest; (c) morphology of adhered HFF cells under SEM on unmodified porous, smooth (scale bar = 10 μm), 0.1% collagen modified porous (scale bar = 2 μm); and 0.1% collagen modified smooth electrospun scaffolds (scale bar = 20 μm). | |
Co-culture of keratinocytes and fibroblasts on electrospun scaffolds
To generate skin-equivalent on the scaffolds, we cultured HaCaTs on the dorsal side of the scaffolds and cultured HFFs on the ventral side through a modified co-culture technique (Fig. 4).
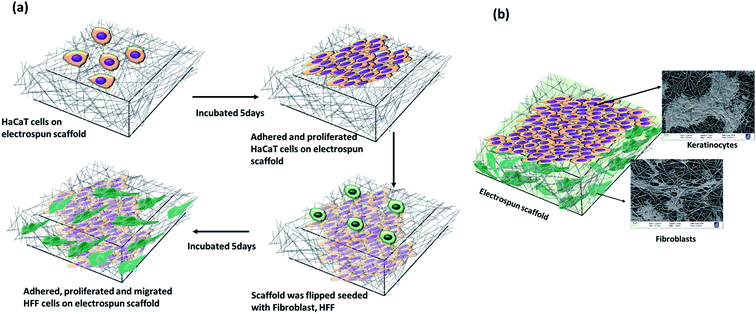 |
| Fig. 4 (a) Schematic of co-culture of HaCaT and HFF cells on either side of electrospun PLLA scaffolds. (b) Adhered and proliferated cells on the electrospun scaffold and their corresponding SEM images of HaCaT and HFF cells. | |
We observed different adhesion patterns on the unmodified scaffolds with different topologies (smooth and porous). We anticipated good adhesion of cells on the porous fibers owing to the roughness of the scaffolds. However, the adhesion of both cell types (HaCaT and HFF) in the porous scaffolds was poor (Fig. 5a-i and iv) compared to the smooth scaffolds. As the concentration of collagen modification increased, we observed an enhancement in the adhesion and proliferation of cells on the porous fibers indicating the positive effects of surface modification. Spreading of HaCaTs to form uniform layers or patches of cells with good cell–cell interaction could be observed through SEM micrographs (Fig. 5a-ii and iii). Collagen-modified fiber samples also showed HaCaTs with well-spread cell bodies. Almost the entire surface of the scaffold (in 0.1% collagen modified porous scaffold) was covered with HaCaTs (10 days of growth in scaffold) and the cells did not shed off from the scaffold indicating enhanced cell–cell interactions and cell–scaffold interactions. Also, additional anchorage spots (interconnected spaces with very fine fibers) owing to collagen modifications may have aided the adhesion of cells. HFFs infiltrated into the 3D fibrous matrix and appeared as elongated shaped cells with spindle morphology and cytoplasmic extensions (Fig. 5a-v and vi) that attached to the fibrous matrix and exploited the nanopores to extend, adhere, elongate and migrate. In smooth fibers, both the unmodified and collagen modified scaffolds supported both cell types (Fig. 5b). However, collagen modification demonstrated superior cell attachment (HaCaT and HFF) than the unmodified fibers suggesting the beneficial effects of having ECM in the environment (Fig. 5b-ii, iii, v and vi). These cellular behaviors confirm the prospects of collagen-modified electrospun scaffolds (smooth and porous) as potential wound dressing biomaterial.
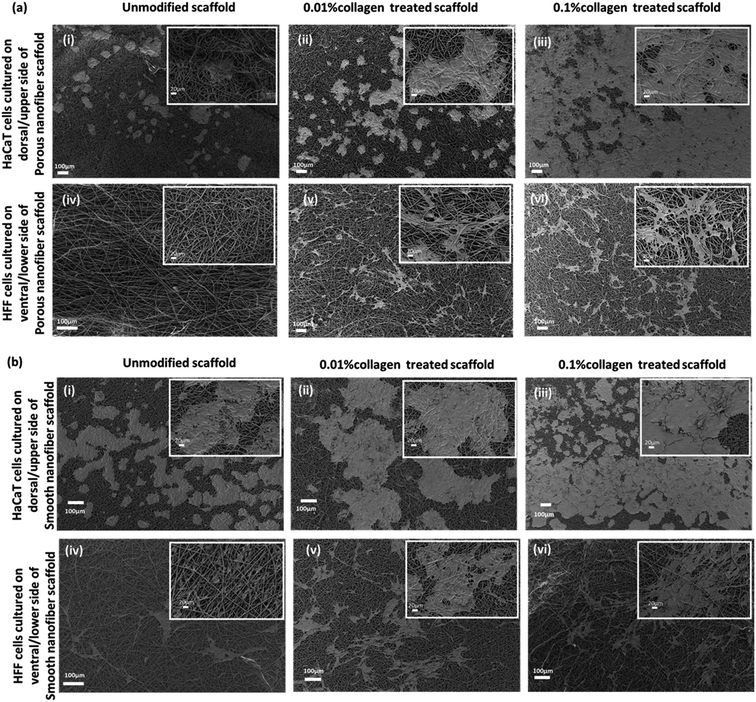 |
| Fig. 5 Scanning electron micrographs of co-cultured skin cells on electrospun PLLA scaffolds unmodified porous, smooth and collagen modified porous and smooth scaffolds. (a) SEM of HaCaT and HFF cells on porous scaffold; (i) HaCaTs on unmodified porous scaffold, (ii) 0.01% collagen treated porous scaffold, (iii) 0.1% collagen treated porous scaffold; (iv) HFFs on unmodified porous scaffold, (v) 0.01% collagen treated porous scaffold, and (vi) 0.1% collagen treated porous scaffold respectively (b) SEM of HaCaT and HFF cells on smooth scaffold; (i) HaCaTs on unmodified smooth scaffold, (ii) 0.01% collagen treated smooth scaffold, (iii) 0.1% collagen treated smooth scaffold, (iv) HFFs on unmodified smooth scaffold, (v) 0.01% collagen treated smooth scaffold, and (vi) 0.1% collagen treated smooth scaffold. Scale bar = 100 μm. Insets are magnified versions of the corresponding surface, scale bar = 20 μm. | |
Immunostaining of adhered keratinocytes and fibroblasts on electrospun scaffolds
Immunohistochemistry revealed that the skin cells adhered well and established skin like self-assembly on nanofibrous scaffolds (Fig. 6). Keratinocyte markers, including K14, laminin V and E-cadherin were used to confirm the self-assembly of an epithelial layer on the nanofiber scaffolds while HFFs cultured on the ventral side were stained for vimentin. Positive staining for K14, which is observed in actively dividing basal keratinocytes, was seen in all scaffolds, however the staining was more robust, especially in collagen-modified scaffolds. Staining confirmed the presence of cells as scattered clusters in unmodified smooth or porous fibers, while they were seen as a layer on the collagen-modified scaffolds (both smooth and porous). Laminin V is an essential protein found in the basement membrane and is required for epidermal cell attachment. Positive staining for laminin V was observed in the HaCaT layers of the scaffolds indicating epidermal attachment was occurring. The interaction between cell–cell and cell–ECM significantly controls the fate of HaCaTs and their differentiation. Tight junctions in the HaCaT layer were demonstrated by E-cadherin staining. The HFFs on the scaffolds were stained for vimentin, which is an intermediate filament typically expressed in mesenchymal HFFs. The vimentin staining revealed characteristic spindle shape of the HFFs. Although the cells on the unmodified scaffolds expressed these markers, collagen modification resulted in uniform presence of K14, laminin V and E-cadherin for HaCaTs and vimentin for HFFs, especially on the porous scaffolds. Collagen modified porous and smooth PLLA scaffolds supported the adhesion and proliferation of HFFs.
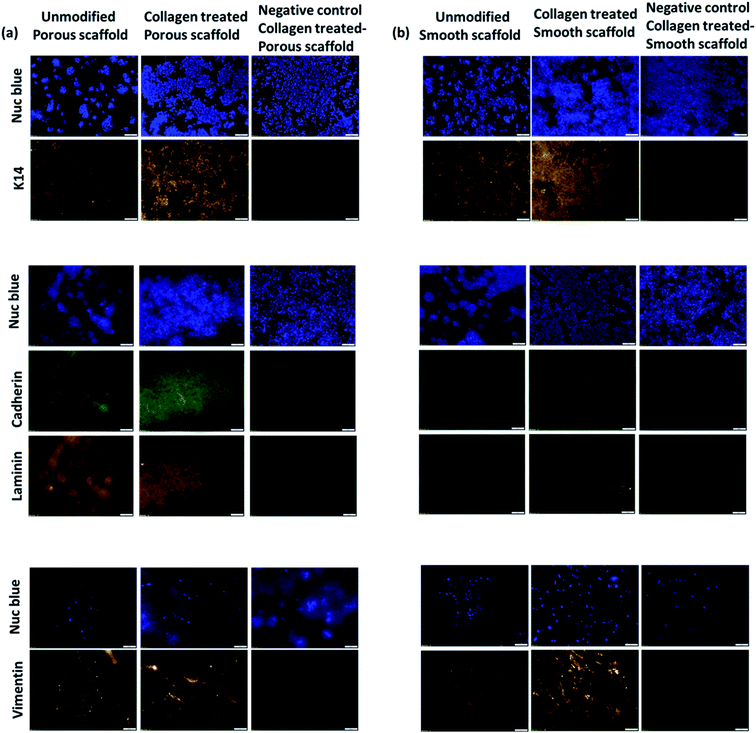 |
| Fig. 6 Immunofluorescence of co-cultured HaCaT and HFF cells in electrospun PLLA scaffolds. Panel (a) corresponds to cells cultured in porous scaffold including unmodified scaffold and 0.1% collagen modified scaffold. A negative control was maintained without the treatment of primary antibodies. HaCaT cells were stained with primary antibodies for cytokeratin 14, cadherin, laminin V and HFF cells were stained with primary antibodies for vimentin, followed by the treatment of fluorescently labelled secondary antibodies. Corresponding nuclei were stained with NucBlue. Panel (b) corresponds the same immunofluorescence staining for HaCaTs and HFF cells in unmodified smooth and collagen modified smooth PLLA scaffolds (scale bar = 100 μm). | |
Discussion
Electrospinning is an attractive and versatile technique for the processing of polymeric biomaterials into nanofibers ranging from micron to nanoscale that functions as scaffolds in tissue engineering applications. It offers the possibility of controlling thickness, and composition, as well as porosity using a moderately simple experimental setup.35 Factors including solution characteristics (solvent, viscosity, concentration, conductivity, surface tension, elasticity), spinning environment (temperature, humidity) and spinning conditions (voltage, distance between collector and spinneret, flow rate, needle gauge) influence the fabrication process required to produce fibers of desired size and topography with features including nano-pores, wrinkles and porous or hollow interiors.36 In this study we investigated the possibility of using electrospun scaffolds made of PLLA with different topologies, functionalized with an ECM component to aid the attachment of epithelial and dermal fibroblasts. PLLA is a non-toxic, biocompatible biomaterial that eventually decomposes to lactic acid which is a safe metabolic by-product. Lactic acid has been reported as an effective wound healing molecule, supporting the potential use of these scaffolds for wound applications.37 We chose a single solvent and binary solvent system to fabricate smooth and porous fibers respectively. The formation of nanopores and changes in internal porosity in electrospun fibers with binary solvent systems of chloroform and DMSO has previously been developed through a non-solvent induced phase separation (NIPS) mechanism.34 Pores are generated when the binary solvents of chloroform and dimethyl sulfoxide (DMSO-slow evaporating and high boiling point, 189 °C) evaporate at the center of the fibers resulting in the formation of non-solvent droplets that eventually combine and dry to form internal pores. In our study we used a similar high boiling point solvent-DMF (boiling point-153 °C) to undergo NIPS which resulted in the formation of pores in the electrospun fibers.
Surface topography describes features including gradual undulations, spikes or pores that exist on the surface of a material.38 In this context the nanopores present in the electrospun fibers demonstrated good interplay between the scaffold and subsequent cellular interactions. Porous fibers mimic the extracellular matrix in native tissue and the pores present in the fibers enable cellular attachment, which is significant for the regeneration of damaged tissue.39 The topographical effect of the scaffold was enhanced by the modification with ECM component-collagen that created a platform for cellular interactions. Cell adhesion and co-culture of skin cells on the scaffolds (unmodified porous, unmodified smooth, collagen-modified porous and smooth) demonstrated superior attachment of cells based on functionalization. Attachment of keratinocytes and fibroblasts in unmodified smooth scaffolds was better than in the porous scaffolds where cellular attachment and proliferation were observed to be limited. The reduced cellular attachment to the porous scaffolds might be attributed to the higher hydrophobicity of the porous PLLA fibers. In a recent study, 0.01% collagen was used to modify PLLA fibers, and the effect on adult human cardiac fibroblast (AHCF) cells determined. Cellular adhesion and proliferation was observed on all fibers regardless of the surface modifications.12 However, in our study which used skin cells, the effect of collagen was more prominent. Here, collagen modification significantly improved the cellular attachment to the scaffolds, suggesting the important role of this ECM component in supporting these cellular interactions. Enhanced cellular attachments were observed as the collagen concentration increased by ten-fold (0.01% to 0.1%). In addition, the collagen modification resulted in ultra-fine fibers, which were able to modulate the surface topography of both the porous and smooth scaffolds. These ultra-fine fibers may have further contributed to the enhanced cellular attachment observed with this collagen modification.
Cells embedded in scaffolds, especially fibroblasts tend to spread well with a distinctive spindle-like morphology and develop 3D cellular networks in scaffold matrices.11,13 In contrast, keratinocytes prefer 2D scaffold surfaces as they have apical-basal polarity. In this study, co-culture of keratinocytes (HaCaTs) and fibroblasts (HFFs) was found to produce a potentially useable skin-substitute material. Both the keratinocytes and fibroblasts were able to attach to the collagen-modified porous and smooth scaffolds. The keratinocytes cultured on the collagen-modified scaffolds expressed basal cytokeratin 14 filaments and formed a tight and uniform layer of epithelial cells expressing E-cadherins and laminin V. Fibroblasts cultured on the collagen-modified scaffolds were found to express vimentin and were well integrated throughout the matrix. This study correlates with other comparable reports that focused on the co-culture of keratinocytes and fibroblasts on collagen modified, collagen blended or other ECM component modified electrospun polymeric scaffolds.9,13,14,30 Our results have proven that native collagen-modified porous and smooth scaffolds with fine 3D microenvironments are ideal for the co-culture of keratinocytes and fibroblasts. Although we modified the scaffold with collagen, over time it could have disintegrated, however we anticipate that when cells are established within the scaffolds, they would be able to secrete and/or remodel collagen enabling the production of new ECM while the polymer scaffold provides optimum structural and mechanical support. Similar results were reported when collagen and other ECM components were modified to PLLA and polycaprolactone (PCL) scaffolds.9,13,14,30 These observations signify that collagen-modified scaffolds could function as a potential skin-substitute supporting a variety of cells and could probably function as an efficient dressing biomaterial for rapid wound healing.
Summary
Surface topography and chemistry of biomaterials have substantial effects on cell responses including cell adhesion, spreading, proliferation, migration and differentiation. In this context, PLLA scaffolds of different topologies (porous and smooth) were fabricated using electrospinning and functionalized with one of the major ECM mimetic proteins-collagen. Although we anticipated superior cell adhesion on the porous scaffolds owing to their surface topology, we found that cell adhesion was actually superior on the smooth scaffolds. However, when the porous scaffolds were modified with collagen, enhanced cell adhesion and migration of keratinocytes and fibroblasts was observed. The cells grew in a highly organized pattern, similar to in vivo conditions within the 3D microenvironment of the scaffolds. Keratinocytes self-assembled to form a thick and uniform epithelial-like construct while fibroblasts infiltrated into the fibrous 3D matrix and continued to proliferate and migrate to establish cellular interactions. In the case of the smooth scaffolds, collagen modification further improved cellular adhesion, signifying the importance of ECM components for cellular activities. Collagen modified electrospun nanofiber scaffolds may therefore be a potential biomaterial that can be used as promising skin substitute supporting a variety of cells to accelerate wound healing. It could be also developed into an efficient wound dressing material for the treatment of skin wounds including chronic wounds and burns.
Conflicts of interest
The authors declare no conflict of interest.
Acknowledgements
This study was supported by the University of South Australia's Early Career International travel award to ARG. The author's thank Professor Toru Meakawa, Professor Tatsuro Hanajiri, Bio-Nano Electronics Research Center, Toyo University, Japan for their support rendered to conduct the work at BNERC. AJC is supported by NHMRC senior research fellowship GNT#1102617. We further acknowledge Microscopy Australia and Dr Nobuyuki Kawashima for technical support.
References
- M. P. Nikolovaa and M. S. Chavali, Bioact. Mater., 2019, 9, 271–292 CrossRef PubMed.
- K. Sharma, M. A. Mujawar and A. Kaushik, Front. Mater., 2019, 5, 172 CrossRef.
- P. Deb, A. B. Deoghare, A. Borah, E. Barua and S. D. Lala, Mater. Today: Proc., 2018, 5, 12909–12919 CAS.
- P. Viswanathan, E. Themistou, K. Ngamkham, G. C. Reilly, S. P. Armes and G. Battaglia, Biomacromolecules, 2015, 16, 66–75 CrossRef CAS PubMed.
- G. Yazgan, R. I. Dmitriev, V. Tyagi, J. Jenkins, G. M. Rotaru, M. Rottmar, R. M. Rossi, C. Toncelli, D. B. Papkovsky, K. M. Weber and G. Fortunato, Sci. Rep., 2017, 7, 158 CrossRef PubMed.
- R. S. Kurusu and N. R. Demarquette, Int. Mater. Rev., 2019, 64, 249–287 CrossRef CAS.
- D. Kanmaz, H. A. K. Toprakci, H. Olmez and O. Toprakci, Mater. Sci. Res. India, 2018, 15, 224–240 CAS.
- X. M. Mo, C. Y. Xu, M. Kotaki and S. Ramakrishna, Biomaterials, 2004, 25, 1883–1890 CrossRef CAS PubMed.
- X. Ren, Y. Feng, J. Guo, H. Wang, Q. Li, J. Yang, X. Hao, J. Lv, N. Ma and W. Li, Chem. Soc. Rev., 2015, 44, 5680–5742 RSC.
- S. H. Ku and C. B. Park, Biomaterials, 2010, 31, 9431–9437 CrossRef CAS PubMed.
- N. T. Dai, M. K. Yeh, D. D. Liu, E. F. Adams, C. H. Chiang, C. Y. Yen, C. M. Shih, H. K. Sytwu, T. M. Chen, H. J. Wang, M. R. Williamson and A. G. Coombes, Biochem. Biophys. Res. Commun., 2005, 329, 905–908 CrossRef CAS PubMed.
- P. Muniyandi, V. Palaninathan, S. Veeranarayanan, T. Ukai, T. Maekawa, T. Hanajiri and M. S. Mohamed, Polymers, 2020, 12, 451 CrossRef CAS PubMed.
- M. Bacakova, J. Pajorova, A. Broz, D. Hadraba, F. Lopot, A. Zavadakova, L. Vistejnova, M. Beno, I. Kostic, V. Jencova and L. Bacakova, Int. J. Nanomed., 2019, 14, 5033–5050 CrossRef CAS PubMed.
- A. Polini, S. Pagliara, R. Stabile, G. S. Netti, L. Roca, C. Prattichizzo, L. Gesualdo, R. Cingolania and D. Pisignano, Soft Matter, 2010, 6, 1668–1674 RSC.
- Y. Zhang, C. T. Lim, S. Ramakrishna and Z. M. Huang, J. Mater. Sci.: Mater. Med., 2005, 16, 933–946 CrossRef CAS PubMed.
- S. Agarwal, J. H. Wendorff and A. Greiner, Polymer, 2008, 49, 5603–5621 CrossRef CAS.
- Y. Ramot, M. Haim-Zada, A. J. Domb and A. Nyska, Adv. Drug Delivery Rev., 2016, 107, 153–162 CrossRef CAS PubMed.
- M. Jafari, Z. Paknejad, M. R. Rad, S. R. Motamedian, M. J. Eghbal, N. Nadjmi and A. Khojasteh, J. Biomed. Mater. Res., Part B, 2017, 105, 431–459 CrossRef CAS PubMed.
- N. R. Richbourg, N. A. Peppas and V. I. Sikavitsas, J. Tissue Eng. Regener. Med., 2019, 13, 1275–1293 CrossRef CAS PubMed.
- S. Yang, K. F. Leong, Z. Du and C. K. Chua, Tissue Eng., 2001, 7, 679–689 CrossRef CAS PubMed.
- P. N. Thanki, E. Dellacherie and J. L. Six, Appl. Surf. Sci., 2006, 253, 2758–2764 CrossRef.
- H. Shin, S. Jo and A. G. Mikos, Biomaterials, 2003, 24, 4353–4364 CrossRef CAS PubMed.
- Y. Yang, M. C. Porte, P. Marmey, A. J. El Haj, J. Amédée and C. Baquey, Nucl. Instrum. Methods Phys. Res., Sect. B, 2003, 207, 165–174 CrossRef CAS.
- N. Tambe, J. Di, Z. Zhang, S. Bernacki, A. El-Shafei and M. W. King, J. Biomed. Mater. Res., Part B, 2015, 103, 1188–1197 CrossRef CAS PubMed.
- M. Asadian, K. V. Chan, M. Norouzi, S. Grande, P. Cools, R. Morent and N. De Geyter, Nanomaterials, 2020, 10, 119 CrossRef CAS PubMed.
- T. I. Croll, A. J. O'Connor, G. W. Stevens and J. J. Cooper-White, Biomacromolecules, 2004, 5, 463–473 CrossRef CAS PubMed.
- M. Cui, L. Liu, N. Guo, R. Su and F. Ma, Molecules, 2015, 20, 595–607 CrossRef PubMed.
- Y. F. Luo, Y. L. Wang, X. F. Niu, J. Pan and L. P. Shi, Chin. Chem. Lett., 2014, 15, 521–524 Search PubMed.
- J. Pan, Y. Wang, S. Qin, B. Zhang and Y. Luo, J. Biomed. Mater. Res., Part B, 2005, 74, 476–480 CrossRef PubMed.
- A. Ospina-Orejarena, R. Vera-Graziano, M. M. Castillo-Ortega, J. P. Hinestroza, M. Rodriguez-Gonzalez, L. Palomares-Aguilera, M. Morales-Moctezuma and A. Maciel-Cerda, Tissue Eng. Regener. Med., 2016, 13, 375–387 CrossRef CAS PubMed.
- W. He, Z. Ma, T. Yong, W. E. Teo and S. Ramakrishna, Biomaterials, 2005, 26, 7606–7615 CrossRef CAS PubMed.
- R. G. Frykberg and J. Banks, Adv. Wound Care, 2015, 4, 560–582 CrossRef PubMed.
- M. P. Prabhakaran, L. Ghasemi-Mobarakeh, G. Jin and S. Ramakrishna, J. Biosci. Bioeng., 2011, 112, 501–507 CrossRef CAS PubMed.
- C. Huang and N. L. Thomas, Eur. Polym. J., 2018, 99, 464–476 CrossRef CAS.
- K. Jayaraman, M. Kotaki, Y. Zhang, X. Mo and S. Ramakrishna, J. Nanosci. Nanotechnol., 2004, 4, 52–65 CAS.
- N. Bhardwaj and S. C. Kundu, Biotechnol. Adv., 2010, 28, 325–347 CrossRef CAS PubMed.
- P. Porporato, V. Payen, C. Saedeleer, V. Préat, J.-P. Thissen, O. Feron and P. Sonveaux, Angiogenesis, 2012, 15, 581–592 CrossRef CAS PubMed.
- H. Assender, V. Bliznyuk and K. Porfyrakis, Science, 2002, 297, 973–976 CrossRef CAS PubMed.
- H. Y. Li, Y. C. Xu, H. Xu and J. Chang, J. Mater. Chem. B, 2014, 2, 5492–5510 RSC.
|
This journal is © The Royal Society of Chemistry 2020 |
Click here to see how this site uses Cookies. View our privacy policy here.