DOI:
10.1039/D0RA04287K
(Review Article)
RSC Adv., 2020,
10, 33782-33835
Recent advances and future perspectives of sol–gel derived porous bioactive glasses: a review
Received
13th May 2020
, Accepted 2nd September 2020
First published on 11th September 2020
Abstract
The sol–gel derived porous bioactive glasses have drawn worldwide attention by virtue of the convenience and flexibility of this versatile synthesis method. In this review, the recent advances in sol–gel processed porous bioactive glasses in biomedical fields, especially for bone tissue regeneration applications have been comprehensively reviewed. Generally, it is envisaged that the morphology and chemical compositions of sol–gel derived porous bioactive glasses significantly affect their biological properties. Therefore, the controlled synthesis of these porous glasses is critical to their effective use in the biomedical fields. With this context, the first part of the review briefly describes the fundamentals of the sol–gel technique. In the subsequent section, different approaches frequently used for the sol–gel synthesis of porous glasses such as microemulsion and acid-catalyzed based synthesis have been reviewed. In the later part of the review, different types of sol–gel derived bioactive glasses namely silica, phosphate and silica–titania based glasses along with organic–inorganic hybrids materials have been discussed. The review also discusses the chemical, surface, mechanical and biological properties and further highlights the strategies to control the pore structure, shape, size and compositions of sol–gel derived bioactive glasses. Finally, the review provides a detailed discussion about the bone tissue regeneration application of different types of sol–gel derived bioactive glasses and presents future research perspectives.
1. Introduction
In recent years, the solution–gelation (sol–gel) processing technique has gained increasing attention across various scientific disciplines because of the wide range of potential applications of the resulting materials. Generally, it is recognized as a wet chemistry-based synthesis technique which offers promising and flexible approaches to obtain a varied type of novel and functionalized materials such as glasses, ceramics and organic/inorganic hybrids with different architectures at low temperatures and mild chemical conditions.1,2 Sol–gel technique is the most dynamic, reliable and environmentally friendly bottom-up synthesis method which has received tremendous interest in diverse research fields such as nanotechnology, optoelectronics, semiconductors, medicines, biotechnology as well as separation science.3 In particular, the sol–gel method is very useful, highly attractive and versatile because of its simplicity, low cost and the diversity of high purity materials of varied configurations such as monoliths, nanoparticles, thin films, foams, fibers etc., that can be produced from the same composition directly from the solutions.1 Furthermore, it is the most exploited technique used for the synthesis of metal oxides and metal oxides based nanocomposites.4 By varying the synthesis parameters in the sol–gel process, homogenous materials with tailored properties such as good chemical and thermal stability, good mechanical strength, good optical transparency and controlled porosity can be obtained.5 The final product obtained via sol–gel synthesis is explicated by the mesoporous texture which is inherent in sol–gel materials.1 Moreover, this technique allows the direct synthesis of high purity multi-component materials without the use of powder intermediates or using high-cost vacuum-based processing techniques.6,7 These advantages make the sol–gel method a promising synthesis route to prepare different types of functional materials with varied structures and porosity.8
A typical sol–gel synthesis method consists of two distinct phases; i.e. solution and gelation. In the first phase, small molecules (precursors) get converted into a colloidal solution (sol) which are generally obtained via controlled hydrolysis and condensation of metal alkoxide precursors or organic/inorganic salts within the solution.8,9 In the second phase, the polycondensation reaction occurs which leads to the formation of a rigid and highly interconnected three dimensional (3D) network (gel) comprising discrete particles or polymer chains due to the addition of a catalyst (acid or base).2,8,9 The structure of the resulting gel depends on the catalyst and this is due to the relative rates of the hydrolysis and condensation reactions.10 Hence, understanding the kinetics of hydrolysis and condensation reactions is the key to conquer the sol–gel synthesis method. In general, a clear and stable solution composed of hydrolyzed monomers having low condensation rates is required for the gelation process.10 Thus, these processes are affected by the number of experimental parameters such as pH of the solution, temperature, reactants concentration and the presence of additives that could be controlled in the sol–gel synthesis.9 The processing temperature in the sol–gel method is generally very low, more often very close to room temperature which further minimizes the thermal volatilization and deterioration of entrapped species and also allows control over the production of novel glass compositions.11 Thus, the sol–gel method renders the possibility to control the physicochemical properties of the resulting material by carefully varying the experimental parameters affecting the various synthesis steps.12 Furthermore, the sol–gel process exhibits several unique merits over other conventional synthesis methodologies. Among the key advantages of the sol–gel method is the ability to produce organic–inorganic hybrid materials in addition to the low production cost as compared with other vacuum-based synthesis methods which are comparatively expensive.13 The other ascendancy of the sol–gel process includes the synthesis of highly pure and homogeneous multi-component systems with controllable kinetics of various chemical reactions namely hydrolysis, condensation, nucleation and the evolution of primary colloidal particles to achieve microstructure with special shape and size distribution.12,14 Besides, the sol–gel method facilitates controlling all these parameters and results in the synthesis of tailor-made homogeneous materials with controlled homogeneity at the molecular scale.14
The adaptability of the sol–gel method allows to manipulate the material characteristics which are required for a particular application. In this context, this technique is a promising tool for obtaining bioactive materials (biomaterials) for numerous biomedical applications owing to its environmental friendliness, low-temperature processing and intrinsic biocompatibility of the synthesized materials.1,15 During the last decade and a half, the demand for the biomaterials have grown significantly and the intense research interest is attributed to their wide range of applications in the healthcare and medical industries, for example; in regenerative medicines, implantable devices, wound healing therapies, tissue engineering, plastic surgeries, drug delivery systems and orthopedic disorders.16–21 In particular, sol–gel derived biomaterials have been recently investigated for the prevention of prosthetic joint infections,22,23 bone cements,24,25 artificial tissue and ligaments,26,27 dental implants,28,29 tissue engineering30–32 and drug delivery.33,34 The biomaterials are anticipated to enhance the natural tissue regeneration, thereby stimulating the restoration of structural, functional, metabolic, biochemical and biomechanical properties.35,36 The growing interest in sol–gel derived biomaterials is due to their potential to form excellent contact and strong chemical bonding with the surrounding tissues.37,38 Furthermore, the sol–gel method was utilized to immobilize biologically active compounds or biomolecules via entrapment or encapsulation throughout the sol–gel derived matrix.39–41 Hence, sol–gel derived materials with a high specific surface area provide good biocompatibility while their external surface enables them to be functionalized easily using suitable biomolecules.
Sol–gel based bioactive glasses have been extensively explored as a promising and highly porous scaffold material for bone tissue regeneration applications owing to their exceptional osteoconductivity, osteostimulation and degradation rate.42–45 These bioactive glasses develop strong bonds with the bone through the formation of hydroxyapatite (HA) or hydroxycarbonate apatite (HCA) layer on the surface by releasing Si, Ca, P and Na ions and stimulate the formation of bone tissues when implanted in the living body.46 Mesoporous bioactive glasses (MBGs) are the latest development of sol–gel derived glasses exhibiting large surface area and porosity with the capability of being functionalized with a broad spectrum of moieties.47 The development of MBGs using the sol–gel method provides higher bonding rates and exceptional degradation or resorptive properties can be achieved.47,48 Since their inception in 2004, the research on the MBGs for the bone tissue regeneration application has grown tremendously.48 An ideal scaffold material for bone tissue regeneration should possess good osteoconductivity, biodegradability and good mechanical properties in addition to a highly porous structure.49 Bioactive glasses with macroporous structures can promote cell infiltration, nutrient delivery, bone ingrowth and vascularization.50 The surface roughness and the micro or mesoporosities were also proven equally important as they influence the ability of a material to stimulate apatite nucleation and cell attachment.50–52 The most suitable materials for bone tissue regeneration/repair application are the one who mimics the natural bone structure and presents specific surface chemistry functions.53 In that context, porous inorganic materials,54,55 calcium phosphates (CAPs) and bioactive glass scaffolds have been developed.56,57 Porous Si–Ti based materials are also fascinating materials for bone tissue regeneration because both Si–OH and Ti–OH surfaces were found to promote HA surface nucleation for in vitro bio-mineralization.58
Hence, this review briefly describes the basic chemistry involved in the sol–gel processing of porous bioactive glasses. Besides, different methods of sol–gel synthesis of porous glasses namely microemulsion and acid-catalyzed synthesis have also been discussed. Moreover, the main focus of this review is to give a comprehensive overview of recent advances in sol–gel derived porous bioactive glasses of different types and compositions for bone tissue regeneration applications. Finally, the preparation strategies of porous scaffolds from sol–gel derived glasses for bone grafting and tissue engineering applications have been discussed.
2. Sol–gel synthesis of porous glasses
The sol–gel process is a facile and highly efficient method for synthesizing porous bioactive glasses since it offers the possibility to tune their properties which can be influenced by some parameters such as hydrolysis ratio, gelation time, aging, drying and calcination temperature etc. The density, pore-volume, specific surface area and porosity of glasses are influenced by the synthesis method employed. As compared with the conventional melt quench synthesis, the sol–gel synthesis method allows the production of glasses with higher purity, high specific surface area and intrinsic porous structure owing to the advantages of low-temperature processing.59 High porosity and high specific surface area of sol–gel derived glasses is normally associated with enhanced degradability and bioactivity but lower mechanical stability. Glasses which possess suitable degradation rate, appropriate mechanical properties, the ability to promote the formation of HA layer, as well as the capability to stimulate biologically beneficial responses are desirable for bone grafting application.60 The formation of the HA layer facilitates a strong bond between the living tissues and the implants.61 Moreover, the formation of HA layers is the characteristic of all the inorganic materials used in the development of orthopedic implants, bone replacements and bone tissue engineering.62 The glasses obtained using the sol–gel method have been utilized as bioactive materials in several applications such as for the encapsulation of proteins, enzymes and biomolecules for controlled drug delivery and bone tissue regeneration because these glasses are biocompatible and possess excellent bioactivity.63 Moreover, it was realized that the molecular structure as well as the enhanced textural properties such as pore size which is associated with the high surface area, negative surface charge and higher dissolution rate are the real key for enhanced bioactivity of sol–gel glasses.64 Thus, based on this technique, numerous strategies were developed for the synthesis of porous glasses. These strategies and synthesis methods are described in the following sections.
2.1 Microemulsion based sol–gel synthesis
Since their inception, the interest in the microemulsions has grown significantly in academic as well as industrial research due to their distinctive properties such as very low interfacial tension, large interfacial area, high thermodynamic stability and the ability to stabilize immiscible liquids.65 Generally, microemulsions are known as an isotropic, homogeneous and thermodynamically stable liquid mixtures comprising of three phases namely oil, water and surfactant.63,66 The oil phase generally consists of long-chain hydrocarbons whereas the surfactants can be defined as the long-chain organic molecules having a hydrophilic head and lipophilic tail.67 At the microscopic level, the surfactant molecules form an interfacial film which separates the aqueous and oil phase. The main difference between the conventional emulsions and the microemulsions is that shear effects are required for the formation of conventional emulsions while microemulsions can be formed by directly adding the components which are further stabilized using surfactant.66 There are three types of microemulsions, (i) oil dispersed in water (O/W), (ii) water dispersed in oil or reverse (W/O) and (iii) intermediate bicontinuous structure type microemulsions which can turn reversibly from one type to another.66,68 Microemulsion technique is an ideal method for synthesizing inorganic nanoparticles with minimum agglomeration.69,70 However, the key drawback associated with this technique is the low yield and the requirement of a large quantity of oil and surfactants.63 For the synthesis of microemulsion based sol–gel glasses, the aqueous phase consists of silicate and metal ion precursors in addition to catalysts.71 The silicate precursors undergo hydrolysis and condensation reaction within the water droplets serving as reactors.66 The water droplets more often collide with each other via Brownian motion and unite together to form bigger droplets. The droplets interact with each other due to collision which is unfavorable for achieving homogenous glass composition.68 The surfactant stabilizes the microemulsion droplets while the oil phase is served as a barrier thereby preventing the agglomeration of nanoparticles.66 The synthesized glass thus exhibits homogeneous dispersions and compositions but they may not be uniform in size due to the breakage of microemulsion drop during their collision.72 Moreover, vigorous washing is essential to get rid of the excessive surfactants and the oil phase before drying and calcination thereby avoiding the conversion of organic residues into nanoparticle aggregations.66
The microemulsion based sol–gel synthesis of MBGs has been demonstrated by several authors by employing hexadecyltrimethylammonium bromide (CTAB) as the surfactant.73–76 In sol–gel processing, surfactants are typically used to reduce shrinkage, prevent cracking and to avoid supercritical drying.77 Generally, CTAB surfactant is employed as a pore-forming agent in the sol–gel synthesis of MBGs.78 The pore size, pore volume as well as the particle size of MBGs can be customized by using different solvents and by varying CTAB concentration.73,74 Recently, Wang and Chen76 reported a facile method for the synthesis of hollow mesoporous bioactive glasses (HMBGs) with controllable shell thickness and excellent monodispersity in the microemulsion system comprising cyclohexane, ethanol and water. CTAB was added to cyclohexane to form microemulsion droplets and also used as a surfactant as well as the template for mesoporous structure.76 The author demonstrated the synthesis of HMBGs with different shell thicknesses as well as different cavity sizes simply by varying the CTAB concentration. Furthermore, the microemulsion technique also contributed to good monodispersibility of HMBGs. The mechanism of HMBGs formation is depicted in Fig. 1.76 The droplets of oil in water microemulsions were formed when CTAB and cyclohexane were mixed with the solution containing water and ethanol which offered reaction vehicle for the synthesis of HMBGs. First, tetraethyl orthosilicate (TEOS) was dissolved in cyclohexane and later triethylphosphate (TEP) and calcium nitrate tetrahydrate (CN) were mixed with the microemulsion system. The hydrolysis and the condensation of the prepared sol were carried out at the oil–water interface using ammonia as a catalyst. The microstructure was formed due to the gathering of the sol particle in the CTAB micelle layer.76 The HMBGs were formed once the organics and nitrates are removed via calcination. Fig. 2 depicts the microstructure of synthesized HMBGs showing good monodispersibility (Fig. 2a) as well as a rough surface (Fig. 2b). The rough surface of HMBGs is beneficial for drug loading. The TEM image depicted in Fig. 2c demonstrates the hollow microstructure of synthesized HMBGs with a large cavity of the particles which can be useful for the loading of bioactive molecules. Fig. 2d–f shows the magnified TEM images of HMBGs prepared using the different concentrations of CTAB.76 From TEM images it was observed that the shell thickness and the cavity size can be tuned by varying the CTAB content. In particular, increased shell thickness was observed with increased CTAB concentration.76
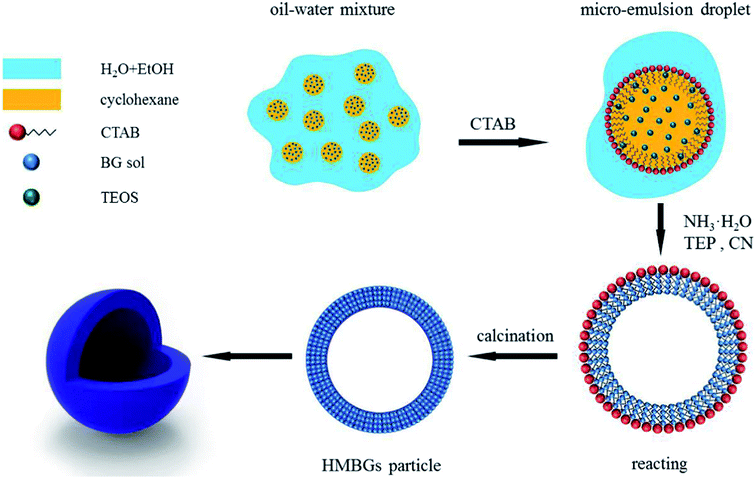 |
| Fig. 1 Mechanism of HMBGs formation. Reproduced with permission from ref. 76. Copyright 2017, Elsevier. | |
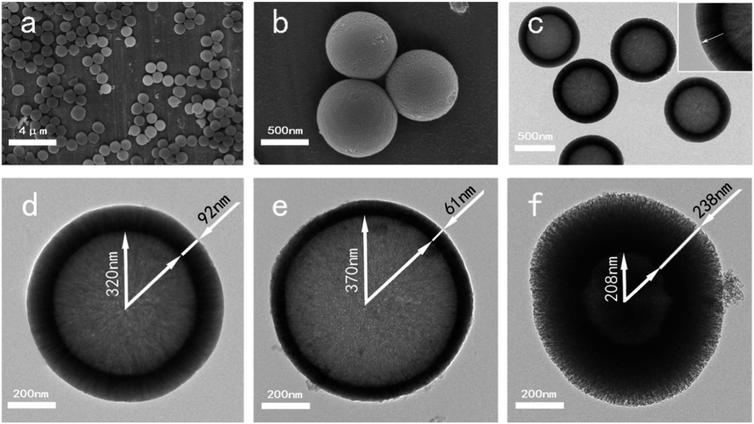 |
| Fig. 2 (a and b) SEM and (c) TEM images of synthesized HMBGs. TEM images of HMBGs with different CTAB concentrations are shown in (d–f). Reproduced with permission from ref. 76. Copyright 2017, Elsevier. | |
Using a similar approach, Wang et al.79 prepared HMBGs nanoparticles in presence of CTAB, cyclohexane, ethanol and water-based emulsion where CTAB played a key role in modulating the interior mesoporous structure, morphology and the dispersion of the HMBGs nanoparticles. The CTAB concentration was varied as 2, 4 and 6 mM and the corresponding HMBGs nanoparticles synthesized were named HMBG-1, HMBG-2 and HMBG-3 respectively.79 The TEM micrographs depicted in Fig. 3 demonstrate that all HMBGs nanoparticles exhibited a hollow structure with different shell property thereby influencing their drug release behaviours. The HMBG-1 nanoparticles exhibited compact shell structure while HMBG-2 showed a peculiar microstructure comprising several penetrative tunnels.79 Moreover, the HMBG-3 demonstrated several mesoporous tunnels similar to HMBG-2. The synthesized HMBGs nanoparticles exhibited a high specific surface area (749.619 m2 g−1) and excellent drug loading efficiency (55.1%) with stable drug release behavior and excellent drug storage ability owing to their hollow structure and the penetrative mesopores on the shell.79 The in vivo studies further revealed that HMBGs nanoparticles can promote bone tissue regeneration with enhanced bone repair capability.
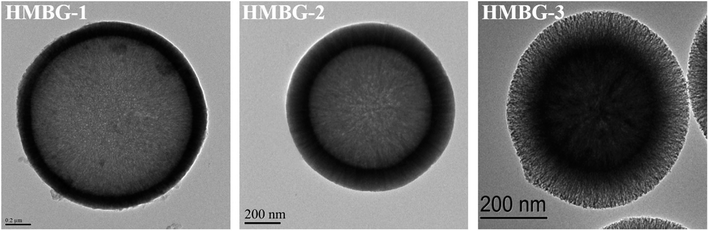 |
| Fig. 3 TEM images of synthesized HMBGs nanoparticles. Adapted from ref. 79. Copyright 2019, Wang, Pan and Chen. | |
Hu et al.80 reported CTAB assisted facile method for the sol–gel synthesis of HMBGs microspheres with tailorable cavity sizes. The authors demonstrated that CTAB acted as a structure-directing agent and favoured the synthesis of HMBGs with hollow mesoporous structure, high surface area and homogeneous particle size. In this study, the size of HMBGs particles and the cavity sizes were determined by CTAB concentration. Therefore, the CTAB concentration was varied as 3.3, 4.6 and 5.9 mM and the corresponding HMBGs synthesized were named as HMBGs-1, HMBGs-2 and HMBGs-3 respectively.80 The authors demonstrated that with an increase in CTAB concentration, the particle size of HMBGs decreased and the morphology changed from hollow spheres to solid spheres. The average particle size for the synthesized HMBGs was reported to be 294 nm for HMBGs-1, 264 nm for HMBGs-2 and 187 nm for HMBGs-3.80 All the synthesized HMBGs displayed narrow particle size distribution, good dispersibility and high specific surface areas.80 Similarly, Duan et al.81 demonstrated the synthesis of HMBGs microspheres via hydrothermal self-transformation method using CTAB as a mesoporous template. Fig. 4 demonstrates the mechanism of the formation of HMBGs microspheres.81 The authors demonstrated that the solid HMBGs spheres prepared in Stöber solution can readily transform into the hollow structure after incubation in hydrothermal conditions.81 Also, the shell thickness of HMBGs microspheres can easily be controlled by adjusting the hydrothermal time. The synthesized HMBGs microspheres displayed mesoporous structure, tunable shell thickness, excellent drug loading capacity and remarkable sustained-release property.81 Besides, HMBGs microspheres exhibited narrow particle size distribution in the range of 300–650 nm and high specific surface area (444.11 m2 g−1).81 These outstanding characteristics of HMBGs microspheres make them a potential drug carrier material for bone tissue regeneration and controlled drug release.81
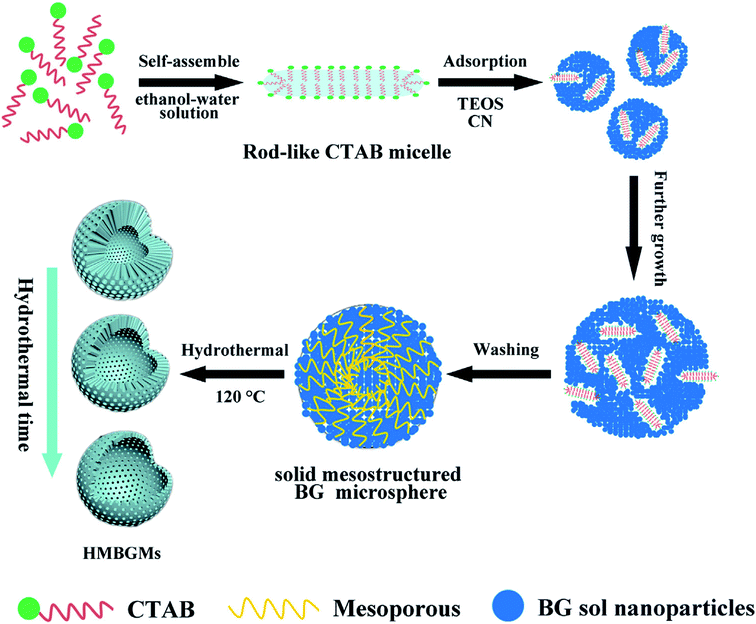 |
| Fig. 4 Mechanism of HMBGs microsphere formation. Reproduced with permission from ref. 81. Copyright 2016, Elsevier. | |
Recently, Xiao et al.82 reported novel method for template assisted sol–gel synthesis of HMBGs nanofibers which were later utilized for fabricating 3D scaffolds using bacterial cellulose (BC) and pluronic P123 as co-templates. It was emphasized that the presence of hydroxyl groups on the surface of BC acted as a catalyst and accelerated the hydrolysis and condensation reaction of precursors and as a result promoted the formation of HMBGs. Fig. 5 schematically represents the fabrication process of HMBGs nanofiber-based scaffold.82 The diameter of the synthesized HMBGs nanofibers was found to be around 40 nm with the wall thickness of 8 nm while the specific surface area of the resulting scaffold was found to be 579.0 m2 g−1. The reported diameter of the synthesized HMBGs nanofibers is the smallest among all the HMBGs nanofibers reported so far while specific surface area is the largest among all the HMBGs currently reported.82 The HMBGs scaffold exhibited nanopore sizes of 3.9 nm (pores present on the wall) and 15.1 nm (pores formed by neighboring tubes).82 The authors further emphasized that the small fiber diameter and the mesoporous structure of the fabricated scaffold with high specific surface area impart excellent bioactivity and renders the HMBGs scaffold as a promising material for controlled drug release and bone tissue engineering.82
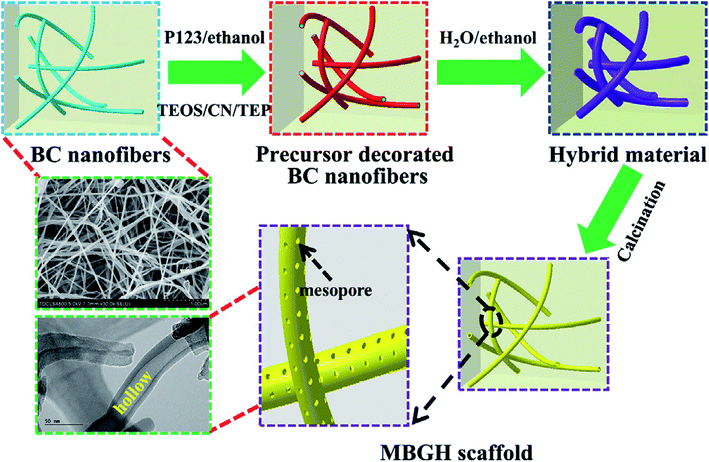 |
| Fig. 5 Schematic illustration showing the fabrication process of HMBGs nanofiber based scaffold. Reproduced with permission from ref. 82. Copyright 2019, Elsevier. | |
2.2 Acid catalyzed sol–gel synthesis
The sol–gel synthesis procedure can occur under acidic or basic conditions which eventually can influence the properties such as porosity, transparency and structure of the resulting materials. The catalyst can be selected based on the desired properties of the final product.83 It was mentioned that the hydrolysis and condensation reactions do not depend on the catalyst type employed but also heavily reliant on the solution pH.84 Strong inorganic acids such as sulphuric acid (H2SO4), hydrochloric acid (HCl) and nitric acid (HNO3) are frequently used as catalysts because they activate the hydrolysis reaction very quickly.85 Bioactive glasses can be prepared by employing a strong acid as a catalyst. However, employing base catalysts can help in inducing the particle formation as they enhance the pH value which in turn can be useful in preventing the development of bulky gel structure of bioactive glasses.86,87 In sol–gel synthesis based on acid/base co-catalysis, first TEOS is mixed with metal ion precursors under acidic conditions and later concentrated basic catalyst is added to accelerate the reaction.66 However, under acidic conditions, tiny colloidal particles are susceptible to form 3D gel network but the presence of salt decreases the stability of nanoparticles.66 Due to these reasons, bioactive glasses usually exhibit polydispersity or agglomerated morphology.86,88 Using acid/based co-catalyzed technique, monodispersed glasses can be obtained by employing weak organic acids such as citric acid (C6H8O7). However, the obtained glasses usually exhibit a rough surface.66,89 Polymeric materials which act as steric berries can also be included during the acid/base co-catalysis to improve the dispersibility of bioactive glasses.66,90 For instance, after the addition of a base catalyst, polyethylene glycol (PEG) has been added as a non-ionic surfactant to tailor the particle size as well as to improve the dispersibility of the bioactive glasses produced.91 Using this strategy, several bioactive glasses have been produced. For example; Nagayama et al.,92 synthesized lanthanum trifluoride (LaF3) doped silica glasses using hydrofluoric acid (HF) acid-catalyzed sol–gel method. HF was employed as a catalyst for sol–gel reaction and as a fluorine source. Fig. 6 depicts the photographs of cracked free dried gel (Fig. 6a) obtained after drying the aged wet gels for 6 to 7 days at 40 °C. The subsequent sintering of the dried gel for one hour at 1150 °C resulted in the formation of monolithic silica glass (Fig. 6b).92 The gel processing time was found to be one week.92
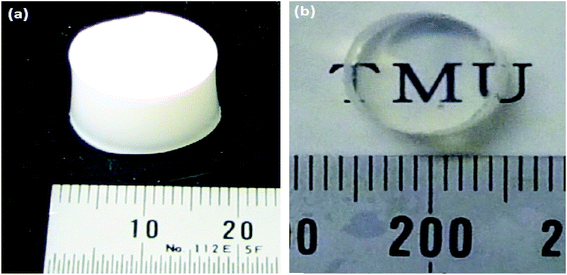 |
| Fig. 6 Photographs of (a) dried silica gel and (b) silica glass obtained by sintering at 1150 °C for one hour. Reproduced with permission from ref. 92. Copyright 2012, Elsevier. | |
3. Types of sol–gel derived glasses
3.1 Silicate based glasses
Sol–gel derived porous bioactive glasses were first time discovered by Li et al.93 in the early 1990s. Since then these bioactive glasses have been studied extensively.94–98 Silicate glasses are the most widely explored sol–gel derived bioactive glass compositions. Understanding the bioactive glass structure is important to demonstrate the role of each component on its bioactivity. The glass structure is frequently explained based on three different components and these are network formers, network modifiers and intermediate oxides.99 Usually, network formers namely silica (SiO2), phosphorous pentoxide (P2O5) and boron trioxide (B2O3) can form glasses without the necessity of additional components.99 Bioactive silicate glasses are amorphous solids that are characterized by a 3D network structure consisting of SiO4 tetrahedron building blocks which are bonded to upto a maximum of four neighboring SiO4 tetrahedra via covalent Si–O–Si bonds, usually known as bridging oxygen (BO) atoms.99,100 Usually, the tetrahedral structures are illustrated by the symbol Qn units (Q stands for quaternary), where n represents the number of BO atoms which are connected to each tetrahedron.94,98 A schematic representation of Si-tetrahedral sites of silicate glasses is given in Fig. 7. In the case of vitreous SiO2, each and every tetrahedron is connected to another tetrahedron of its four corners ascribing to four BO atoms per tetrahedron.99 On the contrary, network modifiers, change the glass structure by turning BO atoms into non-bridging oxygen (NBO) atoms.99,101 The properties of silicate bioactive glasses to a great extent depends on the portion of NBO atoms. Typically, oxides of alkali or alkaline earth metals such as Na+, K+, Ca+, Mg+ are used as network modifiers.99 The bond between the metal ion modifier and NBO is predominately ionic while the bond within Si and BO is covalent.102Fig. 8 schematically depicts a two dimensional (2D) representation of glass modifiers and network formers. The third type of a glass component is intermediate oxides (e.g. ZnO, MgO, Al2O3) which generally served as a network modifier (depolymerize the structure)103 or can be penetrated into the backbone of the glass structure (act like a network former) depending on their content.104 The polymerization of the network i.e. the average number of BO per SiO4 tetrahedron is typically described as the network connectivity of the glass.105 The network connectivity of bioactive glasses tends to be low with values typically in the range of 2 and 2.6. The higher the value of network connectivity, the more connected the network. With the addition of modifier, the Si–O bond breaks down leading to the formation of Q3, Q2 and Q1 units which share 3, 2 and 1 oxygen ions with their respective neighboring units.100 Bioactive glasses mainly consist of Q2 and Q3 units, since they contain low silica content (45–55%).106,107 A metasilicate glass having a chain or ring structure of Q2 group exhibits network connectivity of 2.0 while an increase in the connectivity leads to enhanced polymerization of silicate structure with a subsequent increase in Q3 and Q4 groups.100 The network connectivity in silicate glasses varies from 4 for pure silica glass to 2 for a chain-like structure.100 Thus, network connectivity gives information about the average polymerization of the network108 while it is also useful for estimation of properties of glasses such as crystallization tendency, glass transition temperature as well as bioactivity.105,109 For bio-inactive compositions, the network connectivity is usually greater than 3 while the network connectivity around 2–3 suggests appropriate dissolution and bioactivity.105,108
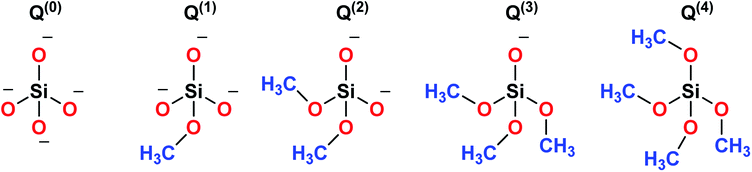 |
| Fig. 7 Schematic representation of Si tetrahedral sites of silicate glasses. | |
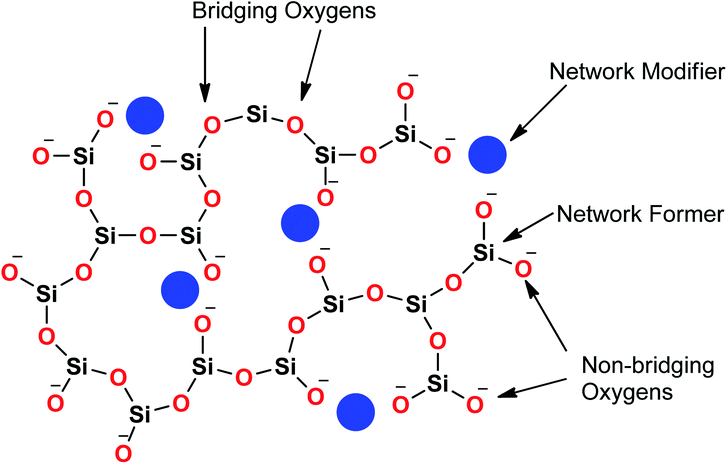 |
| Fig. 8 Schematic representation of glass network formers and modifiers. | |
Silicate glasses have been extensively investigated as inorganic bioactive biomaterials in the field of regenerative medicine since the discovery of first bioactive glass (traditionally known as 45S5 Bioglass®) of composition 45SiO2–24.5Na2O–24.5CaO–6P2O5 wt% in the early 1970s.110,111 Originally, Hench and coworkers used the traditional melting method for producing silicate-based bioactive glasses and later focused on the sol–gel method for synthesizing different glasses and glass derived ceramics.93,112 Since then, there is a growing research activity in this field where innumerable research groups across the globe are seeking novel biomaterials in varied forms and compositions.113–115 The key feature in the bioactivity of the silicate glasses is their composition which is mainly premised on silica as the glass network former which offers stability to the material. In sol–gel silicate glasses, the tetrahedral SiO4 unit condensates as scattered branches or as 3, 4 or 5 SiO4 member ring based on the stabilizing temperature.116 This confirmation leads to high microporosity resulting from the high surface area which further provides characteristic reactivity of silicate glasses.117,118 The addition of a high level of glass network modifiers namely Na2O, CaO, MgO and K2O have been demonstrated to influence the bioactivity of silicate glasses.119 Also, a relatively high CaO/P2O5 ratio was found to transform high reactivity to the glass surface in a physiological environment.120 Therefore, several bioactive glass compositions have been developed over the years having no sodium or containing other elements namely fluorine, magnesium, strontium, iron, silver, boron, potassium or zinc incorporated in the silicate network.114,121–126
Bioactive silicate glasses have instigated tremendous research interest in mineralized bone tissue engineering owing to their ability to form the HCA layer when in contact with biological fluids.127 These glasses are identified by their strong capability to react chemically with the living tissues thereby forming strong and stable bonds with them.128 The high biocompatibility and the favourable biological effects of their reaction products constituted after implantation has made silicate glasses the most promising and interesting group of biomaterials for the last five decades. However, the inferior mechanical properties of these glasses have severely limited their clinical applications.118,129 Nevertheless, silicate glasses display the majority of the chemical as well as biological properties that are relevant to an ideal grafting and scaffolding material namely high surface area, porous structure with regards to overall porosity and the pore size that can foster cell-material interaction and cell invasion.130 Studies have shown that the porous structure of silicate glasses provides a higher surface area that depicts enhanced tissue bonding rates.59 These features promoted new perspectives of silicate glasses as third-generation biomaterials for bone tissue regeneration.131 Moreover, the high reactivity of silicate glasses is the primary advantage for the repair or regeneration of periodontal tissue and bone augmentation because the reaction products acquired from such kind of glasses and physiological fluids promote crystallization of apatite layer which is analogous with the inorganic components of the bone invertebrate species.118 However, as far as scaffold preparation for tissue engineering applications is concerned, it is a drawback to have a high content of Na2O in the bioactive glass composition and thus silicate glasses with low alkali content needs to be designed with good sintering ability, enhanced bioactive properties, controlled chemical dissolution and high mechanical strength.132
The formulation of bioactive glasses using the sol–gel technique opens the possibility of increasing the range of compositions displaying bioactive behavior. The bioactivity and the biocompatibility of sol–gel derived silicate glasses based on the SiO2–CaO–P2O5 system have been widely examined for various biomedical applications.133,134 A rapid in vitro HCA formation was noticed for compositions consisting of 80 mol% of SiO2 in SiO2–CaO–P2O5 and SiO2–CaO glass systems.135,136 A comparative in vivo study of sol–gel silicate glasses based on 58% SiO2–38% CaO–4% P2O5 (58S) and 77% SiO2–19% CaO–4% P2O5 (77S) with melt derived 45S5 bioglass revealed that sol–gel glasses display similar cell response with minor changes in the environmental conditions owing to the lower content of Na+ and Ca+ cations in the glass composition. The long term in vivo studies further validated that 58S and 77S based sol–gel glasses displayed similar responses to melt derived 45S5 bioglass.137 In another study, the in vivo behavior of sol–gel silicate glasses was evaluated to check their eligibility as a material for bone substitution or repair138 while another investigation reported in vitro HCA formation correlated with in vivo behavior of the sol–gel glass.139 Lin et al.140 studied the effect of different bioactive glass compositions on cutaneous wound healing in both normal as well as streptozotocin induced diabetic rats. The bioactive glass ointments developed via mixing the sol–gel synthesized silicate glass of composition 58% SiO2–33% CaO–9% P2O5 (58S), nano bioactive glass (58S) and melt derived 45S5 bioglass powder with 18 wt% of Vaseline were employed for healing the full thickness excision wound. In all three cases, the addition of bioactive glass to Vaseline was found to improve and expedite the wound healing and vascularization process. Moreover, sol–gel derived silicate glasses exhibited significantly higher healing rates than that of melt derived 45S5 bioglass.140 Xie et al.141 synthesized different compositions (60S, 70S, 80S, and 90S) of sol–gel derived silicate bioactive glasses and found that 90S silicate bioactive glass with composition 90SiO2–6CaO–4P2O5 (mol%) displayed excellent support for the proliferation of human foreskin fibroblasts. Therefore, silicate glass particles of 90S composition were utilized as a model for systematic investigation of the wound healing related cellular response of fibroblasts. The results related to the gene expression of extracellular matrix (ECM) demonstrated that 90S silicate bioactive glass particles modified the capacity of critical ECM molecules comprising type I and III collagen, fibronectin, and tenascin-C. Furthermore, it was illustrated that the 90S silicate bioactive glass particles significantly inhibited the differentiation of fibroblasts to myofibroblasts. Finally, the authors concluded that Si4+ ions played a key role in the regulation of cell behavior during the wound healing process thereby accelerating the healing rate with minimum scaring.141 Salinas et al.142 investigated the role of P2O5 on the bioactivity (in vitro behavior) and the textural properties of three different compositions of SiO2–CaO–P2O5 sol–gel glasses. The porosimetry studies revealed that the surface area increased while the pore volume and the pore diameter was reduced as P2O5 content in glasses increased.142In vitro investigations revealed that all the three compositions were bioactive owing to the formation of the apatite layer after soaking in simulated body fluid (SBF). The glass composition with S75 exhibited the highest initial reactivity and the lowest crystallization rate of the apatite-like phase. For glass compositions with S72.5P2.5 and S70P5, the formation of an amorphous CAP layer was slower than for S75, however, the crystallization of apatite was noticed after shorter periods in SBF. Besides, after soaking for 7 days, the layer thickness was decreased with an increase in the P2O5 content in the glasses. Thus, it was found that P2O5 played a very complex role in SiO2–CaO–P2O5 sol–gel glasses where more than 10 mol% of this component leads to non-bioactive compositions.118 Moreover, several investigations123,143–145 have shown that the addition of network modifiers such as MgO into SiO2–CaO–P2O5 sol–gel glasses compositions induce changes in the apatite layer formation when they are soaked in SBF. The existence of Mg2+ cations in the glass composition reduces the apatite layer formation rate but with increased layer thickness in comparison with the glasses without MgO content.118 Also, Saboori et al.146 have shown that the quaternary sol–gel derived bioactive glass system comprising SiO2–CaO–P2O5–MgO exhibits the ability to support human fetal osteoblast cell growth. These bioactive glasses were turned out to be non-toxic and found to be compatible with the segmental defects in the goat model in vivo.146
The doping of various metal ions in the sol–gel silicate glasses has been widely studied with an aim to enhance their bioactivity in a relevant physiological environment and to stimulate the effect of bioactive glasses on osteogenesis and angiogenesis while promoting their antimicrobial properties.46 For example; Bellantone et al.147 reported in vitro bioactivity and antibacterial properties of silver (Ag) doped sol–gel silicate glasses based on the 76SiO2–19CaO–2P2O5–3Ag2O wt% with controllable degradation properties. The addition of 3 wt% of Ag in the silicate glass conferred antimicrobial properties without sacrificing its bioactivity. Ag-doped bioactive silicate glass exhibited a striking antibacterial effect against Escherichia coli with a lowest concentration of 0.2 mg (biomaterial) per mL (culture solution). Above this concentration, the bacterial growth was decreased to 0.01% of that of the control culture solution.147 Similarly, Hu et al.148 studied the potential of Ag-doped SiO2–CaO–P2O5–Ag2O silicate bioactive glass with nanoporosity (pore size ∼6 nm) and high surface area (467 m2 g−1) prepared via the sol–gel method for wound healing applications. The synthesized silicate bioactive glass containing 0.02 wt% Ag exhibited good antibacterial properties against Escherichia coli without cytotoxic effect while the antibacterial rate reached 75% in one hour and 99% in twelve hours. Furthermore, these silicate glasses successfully promoted blood clotting and obtained hemorrhage control in the animal model while the high surface area caused an exceptional hemostatic performance.148 Pratten et al.,149 performed in vitro studies to investigate the ability of Ag-doped bioactive silicate glass coating to prevent bacterial colonization on surgical sutures. The antibacterial effect was studied against Staphylococcus epidermidis with the Ag-doped bioactive silicate glass coating having the greatest effect on limiting the bacterial attachment as compared to the 45S5 Bioglass® coated and the uncoated sutures.149 Catauro et al.150 studied the effect of Ag ion addition on the antimicrobial properties of Na2O–CaO–SiO2. The sol–gel derived silicate glasses showed high antimicrobial effects against Escherichia coli and Streptococcus mutans. Similar studies were later reported by Jones et al.112 where Ag ions were added to sol–gel glass scaffolds for bone tissue engineering applications. Moreover, sol–gel based silicate glasses were doped with other metal ions such as zinc (Zn2+),151–154 and strontium (Sr2+)155–158 in order to enhance their bioactivity and biocompatibility in relevance to their tissue engineering applications.
3.2 Phosphate based glasses
The sol–gel synthesis of phosphate-based glasses is considerably more stringent than the synthesis of silicate glasses. Phosphate based glasses are inorganic polymers consisting of highly degradable tetrahedral phosphate anion (PO43−) which forms the backbone of the structure and the metal cations charge to balance the phosphate chains.159 The basic building blocks of phosphate-based glasses are the PO43− tetrahedra which is analogous to silicate glasses.160 The PO43− tetrahedra are interconnected in the glass structure via covalent bonds to form various phosphate anions as shown in Fig. 9. Phosphate based glasses are mainly based on phosphorus pentoxide (P2O5) which acts as a glass network former. P2O5 is chemically unstable and the incorporation of metal oxides improves its stability. Phosphate based glasses containing various metal oxides such as titanium dioxide (TiO2), ferric oxide (Fe2O3), copper oxide (CuO), zinc oxide (ZnO), and aluminium oxide (Al2O3) have been prepared for different end applications.161–165 However, the most commonly used oxides are sodium oxides (Na2O) and calcium oxides (CaO) which are usually employed in a specific molar ratio to synthesize biologically active glasses.
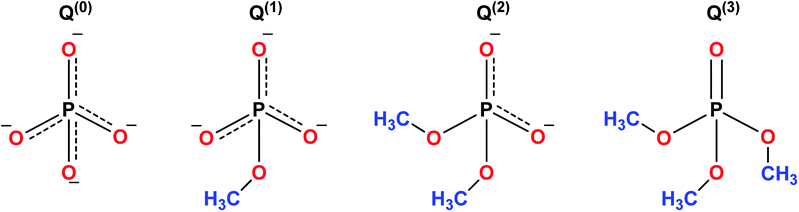 |
| Fig. 9 Schematic representation of PO43− tetrahedral sites of phosphate glasses. | |
The sol–gel derived phosphate-based glasses for the biomedical application were originally presented by Knowles.166 Later, Carta et al.,11,167 described the synthesis of ternary (P2O5–CaO–Na2O) and quaternary (P2O5–CaO–Na2O–SiO2) sol–gel derived phosphate glasses. Further, the same research group investigated these phosphate-based glasses and found structural similarities between the sol–gel derived glasses and the melt derived glasses of the same composition. Hence, indistinguishable bioactivity was anticipated for varied biomedical applications.167 Pickup et al.168,169 reported the low-temperature sol–gel synthesis of binary (P2O5–TiO2) and ternary (P2O5–CaO–Na2O) glasses. These glasses were later tested for drug delivery applications by subsequently releasing the drug molecules in aqueous medium.170 From the biomedical application point of view, phosphate-based glasses have several unique and interesting properties such as the ability to be completely dissolved in the aqueous medium. Furthermore, the dissolution rate can be easily controlled to give glasses with the dissolution rates of several orders of magnitude.166 The degradation of these glasses deviates from a few hours to years depending on the composition and the targeted applications. Phosphate-based glasses can be synthesized in different forms such as discs,171–173 microtubes,174 microspheres175 and fibers.164,176,177 Fibers can be utilized for cell transportation and device expansion,175 nerve conduit178 and as a scaffold for muscle regeneration.165 The key goal of using phosphate-based glass fibers in biomedical applications is to produce tissue-like fibrous constructs which can guide the cell growth. Copper (Cu)-containing fibers with antimicrobial properties have been used as wound dressing meshes for treating severe burns and leg ulcers.162 Phosphate based glass microspheres have also been reported for radiotherapy applications.179 The microsphere morphology provides a stable surface for proliferation and cell attachment preventing tissue damage and hemorrhage during radiotherapy.179 The phosphate-based glass fibers have fascinating properties to form microtubes and hence can be integrated with a wide range of polymers to help nutrients diffusion and ingrowth of vascularization when employed as scaffolds for hard and soft tissue regeneration.180
Phosphate-based glasses belong to the group of unique materials owing to their fully controllable bioresorbable nature and easy doping with a wide variety of ions. In recent years, various glasses have been developed with chemical compositions similar to the mineral phase of bone making phosphate-based glasses promising candidates for the development of implantable biomaterials for repair and regeneration of hard tissues.181 The stability of individual phosphate-based material depends not only on the small changes in the composition but also on the pH and the reaction.1 Phosphate-based glasses containing calcium (Ca2+) and sodium (Na+) ions are the potential materials for applications such as soft and hard tissue engineering because the ions released from them are natural components of the human body.166 In addition to actively taking part in the dissolution process of glasses, Ca is a primary constituent of bones which can trigger bone remodeling. Ca is necessary for the normal functioning of nerves, cells, muscles and bones. Ca2+ ions play an essential role in cell activation mechanisms and control various growth-related processes and cell functioning.182 Furthermore, to confer additional beneficial properties, it is possible to include other cations such as Mg2+, aluminium (Al3+), Zn2+, silver (Ag+) and potassium (K+) within the glass network besides Na+ and Ca2+.46 Also, it has been reported that the extracts of the less soluble glass compositions accelerate cell proliferation and improves gene expression.183 Besides, the cells can be attached, spread and proliferate in a controlled manner in addition to the development of a collagen-rich mineralized matrix.184
Like silicate and phosphate-based glasses, calcium phosphates (CaP) based ceramics also exhibit natural components found in the human body.1,185 However, their biological properties are different. CaP based ceramics are frequently utilized in the field of medicine as bone replacements and as implants coating on dental and orthopedic prostheses.185 HA is the frequently studied CaP material which is analogous to natural tooth and bone and it also represents the highly stable mineral phase in simple aqueous solutions (deionized water). This means that the degradation of CaP based materials in deionized water leads to the development of HA crystals.1 HA and other CaP based materials such as α or β tricalcium phosphate (TCP) are manifested by exceptional biocompatibility due to their structural and chemical resemblance with the inorganic phase of human bone. Several CaP compositions such as α-TCP or tetra calcium phosphate (TTCP) have the ability to become hard (like cement) in aqueous solution making them useful as an injectable biomaterial for treating bone defects.1 Recently, biphasic materials comprising of HA/β-TCP186 and silicon substituted HA were demonstrated for clinical applications.187 The most relevant features of these materials include their biological effect on tissues and in particular on their dissolution behavior which can be ascertained by their morphology, surface topology and chemical composition. Hence, proper designing of material creates the possibility to use CaP based materials in hard tissue regeneration.
Phosphate based glasses containing various therapeutic ions such as Ag+ (antibiotic),188 Ti4+ (promotes growth of new bone),172 fluorine (F) (helps in preventing dental caries/cavities),189 strontium (Sr2+) (taken up in a new bone as a treatment for osteoporosis)190 and cisplatin (chemotherapy drug)170 have also explored. The inclusion of these therapeutic ions certainly changes the structure of phosphate-based glasses and consequently affects their dissolution behaviour. Fig. 10 demonstrates a few selected biotherapeutic ions that can be released from CaP glasses thereby playing a key role in bone repair and regeneration.191 Ca2+ ions are well known for stimulating proliferation of osteoblasts and mineralization of ECM192 whereas Mg2+ ions promote the formation of new bone.193 Furthermore, PO43− ions are required for the deposition of CaP crystal and the mineralization of ECM,194 whereas Na+ ions are usually found in extracellular fluid.195 Other therapeutic ions such as Sr2+,196 Ag+
197 and Cu2+
162 can also be released from the CaP glasses simply by doping the glass composition with the metal oxide of interest. Moreover, both Cu2+ and Ag+ ions have demonstrated antimicrobial properties162,198 whereas Sr2+ ions prevent osteoclast activity while fostering osteogenesis of mesenchymal stem cells in vitro and in vivo.199,200
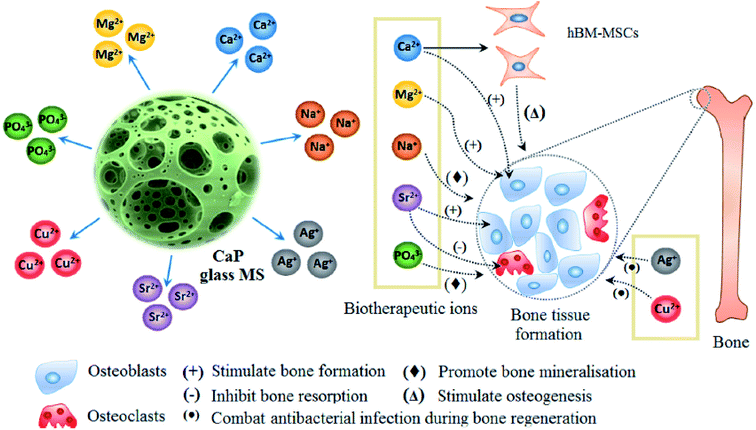 |
| Fig. 10 Releasing of various biotherapeutic ions from CaP glasses and their respective roles in the bone tissue regeneration. Reproduced with permission from ref. 191. Copyright 2018, Elsevier. | |
3.3 Silica–titania based glasses
The sol–gel chemistry-based preparation of silica glasses has been reviewed to a great extent by several authors. However, despite a large amount of work already reported on sol–gel silica-based glasses, considerably less information is available about the sol–gel glasses derived from silica–titania (Si–Ti) based binary systems, particularly for biomedical application. Over the past few years, Si–Ti binary systems have been largely used as a catalyst in the forms of amorphous oxides, crystalline titanium silicates etc.201,202 In addition to the catalytic application, one can also anticipate enhanced thermal stability, mechanical strength, resistance to alkali and zero thermal expansion upon addition of Ti in the Si network.203,204 For developing sol–gel derived Si–Ti based materials, control over hydrolysis reaction is essential for obtaining homogeneous gels. This is because the hydrolysis and the condensation reaction rates of titanium alkoxide are considerably higher than that of silicon alkoxide owing to the low electronegativity of titanium and its propensity to display several coordination states.205 To compensate for their hydrolysis rate differences, bulky alkoxy groups for titanium and methoxy or ethoxy groups for silicon have been used.206,207 In some cases, chelating reagents such as acetylacetone (Acac)208,209 have been used and in some cases, the two-step hydrolysis210 method was adopted to obtain homogeneous Si–Ti based gels. Konishi et al.211 demonstrated the formation of a well-defined interconnected gel network with Ti system with macroporous morphology using the phase separation method. The phase separation is generally induced by the organic polymer present in the system. The phase separation also enables control over gel morphology to a great extent.212 Zhu et al.201 illustrated the preparation of Si–Ti based mesostructured monoliths via the sol–gel method combined with the liquid crystalline templating approach. The authors suggested that the synthesized monoliths could be used as excellent support for gold catalysts.
Ruzimuradov213 reported the synthesis of Si–Ti monoliths with bicontinuous macropores by sol–gel method combined with phase separation using various titanium precursors. TEOS, titanium(IV) isopropoxide (TIP), titanium tetrabutoxide (TBOT), titanium tetrachloride (TiCl4) and titanium sulfate (Ti (SO4)2) was used as the source for Si and Ti respectively. Furthermore, Acac was utilized as a modifier to prevent the reactivity of TIP. PEG was utilized as a polymeric constituent to instigate phase separation. It was observed that the Si–Ti based monoliths comprise a bimodal porous structure with homogeneously dispersed Ti in the Si network. Fig. 11 and 12 depicts the microstructure of the dried gels obtained using different types of titanium precursors. For all the compositions, the interconnected macroporous structure was observed when the transitional structure during phase separation was frozen due to sol to gel transition of inorganic constituents.213 Nevertheless, a large difference in phase separation tendency and the dispersion of Ti in the Si network was observed with different titanium precursors used. Furthermore, for titanium alkoxide system the interconnected porous structure was observed at 30 °C with Ti content of 7.5 wt% (Fig. 11a and c) and 11.2 wt% (Fig. 11b and d) and no phase separation was noticed at 50 °C. When TiCl4 and Ti(SO4)2 were used as titanium precursors, the gels with macroporous structures were obtained at 50 °C with the 7.5 wt% Ti content (Fig. 12a and c), 14.7 wt% (Fig. 12b) and 18.2 wt% (Fig. 12d). Also, the authors observed that the phase separation tendency largely decreased when titanium alkoxides were incorporated into the pure silica sol–gel system. On the other hand, when the titanium salts were utilized, the phase separation propensity changed a bit as compared with the pure silica system.
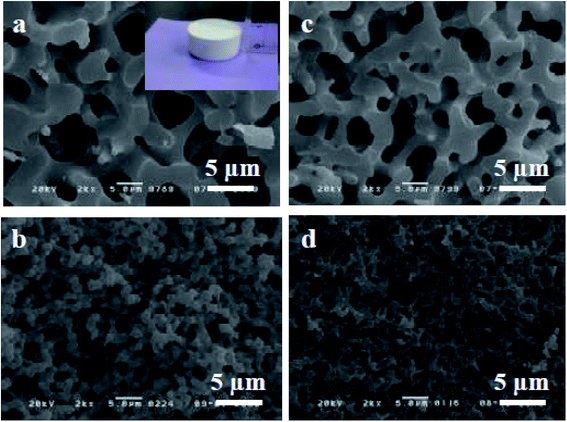 |
| Fig. 11 SEM micrographs of dried gels prepared using (a and b) TIP–Acac and (c and d) TBOT. The figure inset shows the photograph of the synthesized Si–Ti based monolith. Adapted from ref. 213. Copyright 2011, IOP Publishing Ltd. | |
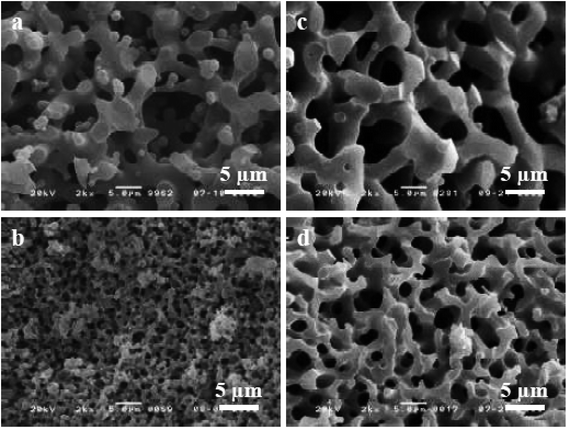 |
| Fig. 12 SEM micrographs of dried gels prepared using (a and b) TiCl4 and (c and d) Ti(SO4)2. Adapted from ref. 213. Copyright 2011, IOP Publishing Ltd. | |
In another study, the same group of authors demonstrated the synthesis of macroporous Ti–Si monolith through co-gelation, two-step hydrolysis and Acac complex methods.205 The authors also investigated the effect of different Ti precursors on the propensity of phase separation and the homogeneity of the resultant Ti–Si based gels. Fig. 13 depicts SEM micrographs of Si–Ti based monoliths prepared using three different methods. All the three specimens showed similar morphology where macropores were arranged bi-continuously. For these three samples, it was considered that the phase separation occurs via spinodal decomposition within the sol–gel reaction. In spinodal decomposition, the morphology became a superposition of an infinite number of sinusoidal compositional waves having a constant wavelength and randomly oriented in 3D space.205 Besides, the macroporous morphologies were obtained for the specimens synthesized using co-gelation and two-step hydrolysis method when the reaction temperature was between 25–30 °C.
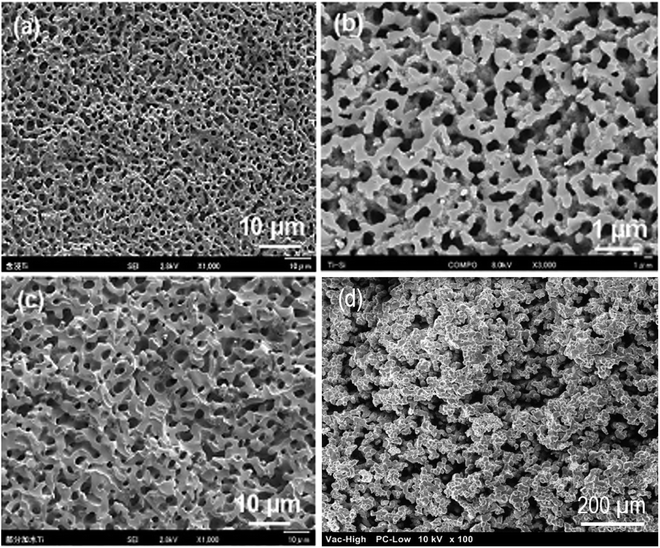 |
| Fig. 13 SEM micrographs of fractured surface of Ti–Si based samples prepared with (a) impregnation (b) co-gelation, (c) two step hydrolysis with 0.6 g PEG and 11 g water. Adapted from ref. 205. Copyright 2012, Springer Nature. (d) SEM micrograph of Si–Ti sol–gel glasses prepared via similar method using PEG as phase separating agent and HNO3 as catalyst. | |
Recently, our group has demonstrated a similar approach for the sol–gel synthesis of Si–Ti based porous glasses where PEG has been used to induce phase separation, HNO3 have been utilized as a catalyst while TEOS and TIP has been employed as a source for Si and Ti respectively.214 The SEM micrograph of the prepared Si–Ti based dried gel is shown in Fig. 13d which revealed the interconnected macroporous network structure. Such type of microstructure is generally observed due to the occurrence of phase separation during sol–gel reaction.205,214
Nakanishi et al.215 prepared Ti–Si based gels with a well-defined interconnected porous structure using the phase separation method. The author prepared these samples by a co-gelation method where acidic water was added to a mixed alkoxide solution of TMOS and TBOT. However, the dispersion of Ti in the Si network was not studied in detail but it was expected that the dispersion of Ti in macroporous Ti–Si based materials can be modified by changing the synthesis method.205 In another investigation,216 hierarchically organized Si–Ti monoliths with the high specific surface area were synthesized by the sol–gel technique under purely aqueous conditions using 3-[3-{tris(2-hydroxyethoxy)silyl}propyl]acetylacetone as a precursor modified by ethylene glycol. Aravind et al.217 illustrated the synthesis of crack-free Si–Ti-aerogel monolith with a high specific area and mesoporous structure for catalytic applications. A 3D printing method in combination with the sol–gel technique has also been reported for the synthesis of optically transparent Si–Ti glasses.218 The characterization of 3D printed Si–Ti glass demonstrated that their properties including chemical composition, refractive index, optical transmission and thermal expansion coefficient are comparable to commercially available Si–Ti glasses.218 The addition of Ti to Si decreases the thermal expansion and increases the refractive index of the resulting glass. Moreover, it has been shown that the addition of Ti to Si improves the network flexibility and the free volume of the glass while its tetrahedral structure was preserved.219 Besides, Si–Ti glasses have also been used for the fabrication of optical parts such as mirrors, optical waveguides220 and gradient index glass optics.221
Si–Ti based aerogels are actively used as a catalyst due to prominent mesoporosity and also due to the homogeneous dispersion of Ti in the Si network. The effectiveness of Si–Ti based catalysts greatly depends on the molecular level dispersion of Ti atoms, large surface area as well as pore diameters in the mesoporous range.77 The high-temperature treatment induces considerable Si and Ti segregation which nullifies the porous structure established during sol–gel processing. In the literature, there are several strategies suggested for the sol–gel synthesis of Si–Ti based aerogels. The Si–Ti based aerogels synthesized by co-gelation of alkoxide have a surface area of 400–700 m2 g−1, a pore size of 10–30 nm, a pore volume of 2–3 cm3 g−1 and pore densities within 0.34–0.38 g cm−3 range.217,222 Supercritical drying at low temperatures has been suggested to produce aerogels with low micro porosity and high surface area and amorphous mixed oxide aerogels.223 Deng et al.222 and Xu et al.,224 reported the synthesis of Si–Ti based aerogels which exhibit high mechanical strength and high porosity with Ti/Si molar ratio of 1
:
5. The addition of Ti to a great extent modify the pore structure of the gel system. Pure Ti aerogels possess a surface area of ∼100 m2 g−1. It was found that the higher content of Ti in the Si–Ti based aerogels yields low surface area at all the compositions, which could be either due to decreased pore accessibility or due to the occupancy of Ti in the aerogel pores.217,225 Another reason for the decrement of specific surface area with increment in Ti content could be the poor interactions between TiO2 and SiO2 in the Ti–Si based aerogels and the high amalgamation of TiO2 particles in the gel.222
The sol–gel technique has also been utilized for the synthesis of bulk Si–Ti based glasses. Deng et al.226 reported colloidal sol–gel method and succeeded in preparing bulk Ti–Si based glasses with large crack free specimens in different shapes such as glass rods (50 mm length, 5 mm diameter) and glass disc (5 mm thick, 40 mm diameter). A dense and transparent glass was obtained which is the same as the glass obtained using the melt quenching method. In another study, Satoh et al.227 demonstrated the synthesis of Ti–Si based bulk glasses using silicon and titanium alkoxides. Recently, El-Bashir228 fabricated Si–Ti based glass monoliths doped with Nd3+ ions. The synthesized glasses were recommended as a potential material for photo-resistive and photo-capacitive sensor applications. Guangwu and Yangang229 reported the synthesis of Si–Ti based aerogel monolith with a Ti content of 26% by mass and drying under supercritical conditions using ethanol as a drying agent. Fig. 14 demonstrates the synthesized crack free monoliths of Si–Ti based aerogels with a density of 0.135 g cm−3. The results from SEM analysis revealed that Si–Ti based aerogel was more compact than the pure silica aerogel with discontinuous microdomains of ordered porosity. Besides, the Si–Ti based aerogel revealed broad pore size distribution which was reasoned to be due to ethanol supercritical drying.229
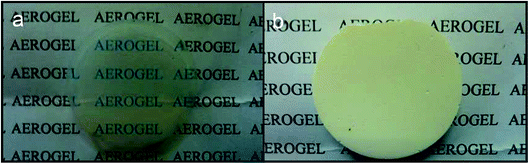 |
| Fig. 14 Photographs of sol–gel derived (a) Si (b) Si–Ti based aerogel monoliths. Adapted from ref. 229. Copyright 2016, IEEE. | |
3.4 Organic–inorganic hybrids
Sol–gel based organic/inorganic hybrid materials are rapidly becoming a fascinating research field in materials science. In the past decades, there has been tremendous progress in the synthesis of new organic/inorganic hybrids for various specific applications.230 Hybridization is a multifaceted strategy by which multifunctional materials can be designed and produced with the synergistic effect of both organic and inorganic components leading to improved performance.231 The organic/inorganic hybrid materials are comprised of interpenetrating networks (IPNs) of various organic and inorganic phases with the scale of each phase in the range of 1 to 100 nm. These phases have nanoscale interaction with each other and enable the whole material to become a single phase contrary to traditional nanocomposites.96,232 This facet of hybrid materials is accountable for highly controllable degradation rates and the potential for tailoring the mechanical properties as per the specific application needs. Furthermore, the molecular level dispersion of constituents facilitates an improved interaction with the cells resulting in instantaneous cell adhesion on the surface of the material.196 For the synthesis of organic/inorganic hybrids, a polymeric component is induced in the sol–gel method right after the hydrolysis of TEOS. This allows the development of an inorganic network in the vicinity of organic molecules leading to molecular level interactions.233 The gelation of organic and inorganic phases occurs simultaneously and results in the formation of 3D IPNs.1 Therefore, this process can be employed for producing elastic hydrogels, flexible rubber and glasses with mesoporous structures, good physicochemical stability, high biocompatibility and reduced shrinkage.234
The organic/inorganic hybrid materials can be categorized as Class I and Class II hybrids depending on the type of interaction between organic and inorganic phases.235 Class I hybrids represent the materials exhibiting non-covalent or ionic–covalent bonds within the organic and inorganic phases. In this case, the constituents of the hybrid interact with each other via weak hydrogen bonding interactions, van der Waals forces, π–π interaction or electrostatic forces. Class I hybrid materials can be obtained via approaches such as hydrolysis and condensation of alkoxides in the presence of organic polymers and blending alkoxide and organic constituents. Few examples of Class I hybrids are polyphosphazene-metal oxide,236 silica–poly-(N,N′-dimethyl acrylamide) (PDMAAm),237 silica–polyvinylpyrrolidone (PVP),238 polydimethylsiloxane (PDMS)–silica,239 polymethylmethacrylate (PMMA)–silica240 and poly(n-butyl methacrylate)–TiO2 hybrids.241 The drawback of Class I hybrids for tissue engineering applications is the absence of chemical bonds within the organic and inorganic phases that leads to rapid dissolution in presence of water because of the chain separation caused by water molecules.235 Class II hybrids represent the materials in which the organic and inorganic constituents interact mutually via strong covalent or ionic–covalent bonds.1 Crosslinking is the main feature of Class II hybrids. Generally, coupling agents are utilized for forming covalent bonds between a polymer matrix and the silicate network.235 For the synthesis of these hybrids materials, the polymer is first functionalized using a coupling agent before it is introduced in the sol–gel process.96Fig. 15 schematically represents the possible interaction mechanism where the polymer matrix is incorporated at the beginning of the process to form inorganic silica chains around the polymeric molecules which lead to the formation of a hybrid having molecular level interaction.235 It has been suggested that the molecular level interactions within organic and inorganic phases lead to controlled and uniform biodegradation with tailored mechanical properties.242 Moreover, the molecular level interaction also suggests that the cells will contact the organic and inorganic phases simultaneously when they interact with the surface of the hybrid and thereby retaining the biological properties of bioactive glass.235
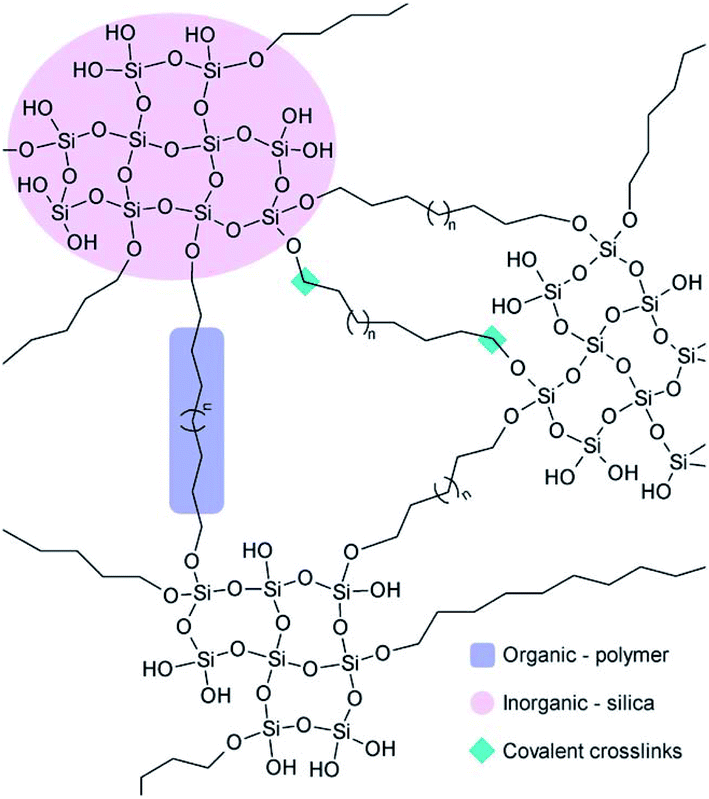 |
| Fig. 15 Schematic representation of polymer–silica Class II hybrids. Adapted from ref. 235. Copyright 2011, the Royal Society of Chemistry. | |
As far as biomedical applications are concerned, bioactive glasses represent the most proficient inorganic phase for obtaining hybrid structures. The bioactive glasses are generally comprised of binary SiO2–CaO system or SiO2–CaO–P2O5 and SiO2–CaO–Na2O ternary systems having a good bioactive response and identical degradation rates. The addition of sol–gel derived glass particles into a suitable polymer matrix is a promising strategy to ameliorate the performance of the base polymer, especially mechanical strength and biological activity.233 For instance, Kamitakahara et al.,243 investigated the biological activity and mechanical behaviour of PDMS–CaO–SiO2 hybrids prepared with the varied calcium content. These hybrids were reported to form HA in SBF solution when tested in vitro. In another study, Sanchez-Tellez et al.244 studied the sol–gel derived SiO2–CaO–P2O5 hybrids for bone tissue regeneration. Several other synthetic or natural biopolymers such as PVA,245,246 PEG,247 gelatin,248 chitosan249,250 and poly(ε-caprolactone) (PCL)251,252 were also used in the preparation of hybrids for biomedical applications. Such kinds of hybrids are generally synthesized by blending a polymer solution with silica sol followed by gelation. Recently, Catauro et al.253 investigated the sol–gel derived PCL/zirconium oxide (ZrO2) hybrids for implants coatings. In another study based on a gelatin–SiO2 hybrid system, an improved apatite forming ability and osteoblast biocompatibility were observed.254,255 Besides, nanostructured chitosan–siloxane hybrids also showed improved HA forming ability with fascinating photoluminescent properties.256 Ohtsuki et al.257 synthesized bioactive organic/inorganic hybrid comprising 3-methacryloxypropyltrimethoxysilane (MTMOS) and 2-hydroxyethyl methacrylate (HEMA) using sol–gel technique. These bioactive hybrids demonstrated the apatite formation ability when mixed with calcium chloride (CaCl2) and recommended to be used as a novel material for bone repairing with both biological and mechanical behaviour closely matching with the conventional PMMA cement.258 Thus, from these studies, it has been ascertained that the bioactivity or biodegradability of the sol–gel synthesized inorganic/organic hybrid materials can be controlled by varying the compositions of organic and inorganic phases which ultimately results in the better material properties.
4. Properties of sol–gel derived porous glasses
4.1 Mesoporous structure
The intrinsic characteristic of the sol–gel process is the production of materials with micro and mesoporous structures. In general, for sol–gel based glasses the porous structure is established either during synthesis or by successive treatment. On the basis of ascendant pore size and as per the classifications of IUPAC, the porous materials are classified as; (i) microporous materials possessing pore diameter up to 2.0 nm (ii) mesoporous materials exhibiting pore sizes in the range of 2 to 50 nm and (iii) macroporous materials comprising pore sizes greater than 50 nm.259,260 The defining properties of any mesoporous material are most often the pore size and structure. The pores can be isolated or can be interconnected with the homogeneous (similar) or heterogeneous (dissimilar) shape and size distributions. The silicate materials acquired by the traditional sol–gel technique are most often manifested by mesopores with an average pore size in the range of 10–20 nm.261 The small size mesoporous materials can be synthesized via sol–gel method combined with supramolecular chemistry in which surfactant is generally employed as a mesopore template.233,262 Using this approach, the mesopore size can be finely tuned which is very much crucial especially for the materials to be designed for biomedical applications namely drug delivery systems.263 This route is generally adapted for developing MBGs with a highly controlled mesopore size.264 Primarily for biomedical applications, the larger pores can be advantageous to fit in certain cell types for the promotion of tissue engineering or regeneration activities while smaller surface pores can be advantageous to control the drug release or the release of small biological components or biomolecules.191
The surfactants are frequently used in the synthesis of mesoporous silica where they are served as a template for in situ polymerization of ortho-silicic acid. Typically, the surfactant is a kind of liquid crystalline mesophase comprising amphiphilic molecules which form micelles in the water-containing medium.233 The pore size can be under the influence of several factors such as the chain length of the surfactant employed within the synthesis or the inclusion of auxiliary organic molecules for attaining more efficient control over pore dimensions.233 These methods create the possibility to incorporate large molecules and influence their release rate as the pore size also has a negative impact on the diffusion of drugs which are loaded in the delivery medium.265 Several features such as pore interconnectivity, pore shape and size distribution of the pore need to be considered for the characterization of porosity. The pore size should be regulated to meet the requirements of various applications as depicted in Fig. 16.1 The pore structure and size control is pivotal for producing the living cell substitutes since porosity features are critical in ascertaining the existence of the interaction between the living cells and the surrounding environment.233Fig. 17 demonstrates that the surface area, pore diameter, pore volume and the surface functionalization of pore using organic molecules can have an impact on the loading and release rate of biomolecules.1 For certain drug delivery systems, it can be beneficial to have a bilateral pore size distribution (formation of dual mesoporous material) which can be achieved using binary surfactants having different molecular weight during the fabrication of surfactant constituted mesostructures.1
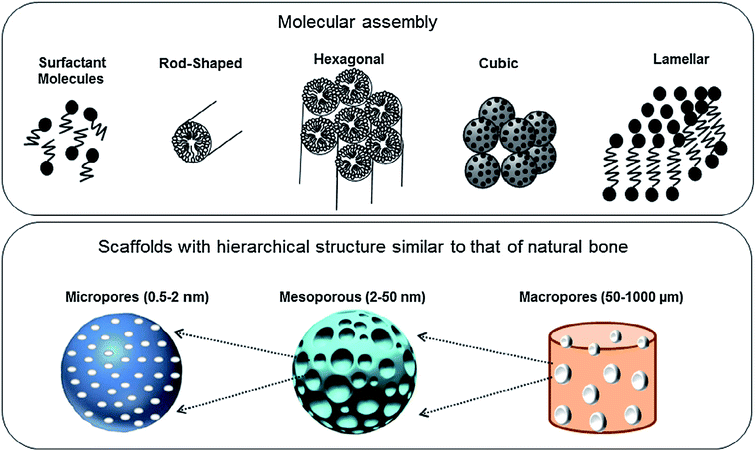 |
| Fig. 16 Molecular assembly and different levels of pores created to design scaffolds for bone tissue repair. Adapted from ref. 1. Copyright 2016, Elsevier. | |
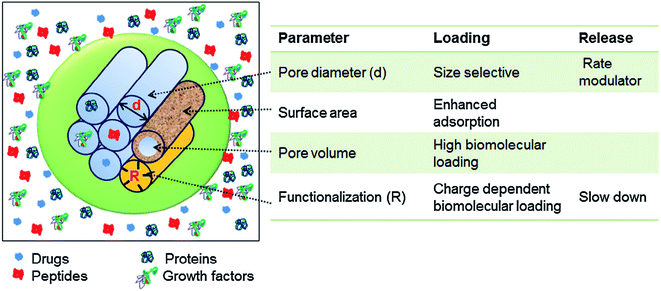 |
| Fig. 17 Various parameters controlling the loading capacity and the release rate of biomolecules in mesoporous materials. Adapted from ref. 1. Copyright 2016, Elsevier. | |
Hollow mesoporous bioactive glass (HMBG) spheres have also been produced by template-assisted or microemulsion based sol–gel synthesis.266,267 The hollow structure is capable of providing large specific surface area and voids for bioactive glasses which is especially fascinating for drug delivery applications. For achieving hollow mesoporous architectures, co-templates are most often investigated.76,91,266 Mesoporous shells can be developed using templates such as CTAB or pluronic P123 and the voids in the bioactive glasses can be created by hard templates such as polymeric particles268 or soft templates such as microemulsion drops and micelles.76 Furthermore, porosity in fact can be controlled by altering the type of template. For example; radial MBGs were obtained by sol–gel synthesis using cetyl pyridine bromide (CPB) as a template269 whereas hexagonal bioactive glass spheres were obtained using pluronic F127 as templates for bone grafting and drug delivery applications.270 Thus, MBGs with relatively large pore sizes can be realized by the microemulsion assisted sol–gel method.73
4.2 Morphology, shape and size
Using the sol–gel process, materials with a broad range of shapes and morphologies can be generated at the micro and macro scale. For example, thin films, bulk glasses, porous foams, fibers, microspheres and nanoparticles etc. The morphological features such as the size and shape of mesoporous glasses are essential and need to be controlled significantly for their efficient biomedical applications. Mesoporous glasses with small particle size stimulate apatite formation at a faster rate when exposed to body fluid which is favorable for applications such as orthopedic implants coating. The small size also facilitates cellular uptake thereby it is useful for drug delivery or delivery of biologically active ions. Furthermore, mesoporous glasses with small sizes also exhibit a larger surface to volume ratio which enables them to be incorporated with polymer matrices to form polymer nanocomposites.233 The size of the bioactive glass can be adjusted by the processing parameters used in the synthesis. The size of bioactive glass particles is inescapably due to the concentration and the time at which the precursors are included in the synthesis process.271,272 Also, the use of organic species tenders a manageable approach for adjusting the size of bioactive glasses. By merely varying the concentration of such organic species, the size of the bioactive glass can be easily controlled. The particle size also gets affected by the kind of template used during the synthesis.273 In microemulsion based sol–gel synthesis of bioactive glasses, the particle size can be adjusted by fine-tuning of microemulsion droplets, for example by changing the concentrations of the catalyst.73
The sol–gel derived bioactive glass particles are generally spherical. The spherical shape tenders suitable flow properties for directly contacting the body fluids. Nevertheless, non-spherical particles are also useful for certain biomedical applications.67 For instance; nanoparticles with rod-like shape and high aspect ratio can have more efficient cell attachment than spherical nanoparticles making them beneficial for specific anticancer drug delivery.274 Furthermore, when employed as a bioactive reinforcing filler, rod or fiber-like bioactive glass particles demonstrate enhanced mechanical properties in comparison with the spherical shape particles.275 Liang et al.73 reported a synthesis of two different types of MBGs (i) spherical shaped bioactive glasses with radial mesostructure and (ii) pineal shape mesoporous glasses with lamellar mesostructure via the sol–gel process combined with liquid template method. Recently, Li et al.276 described the fabrication of MBGs with distinctively different shapes where CTAB was used as a template for shaping mesoporous glasses. The high concentration of CTAB resulted in the formation of mesoporous glasses with rod-like shapes and low concentrations of CTAB tend to form spherical shaped particles. The addition of surfactants such as CTAB, pluronic P123 and pluronic F127 is crucial in achieving well-ordered structures. It was reported that the structure controlling agents have an influential role on the shape and size of mesopore, surface area as well as pore volume of MBGs.1 Usually, CTAB induced mesoporous glasses exhibits smaller pore size (2–3 nm) as compared with P123 or F127 induced glasses (4–10 nm). Moreover, P123 induces a 2D hexagonal mesoporous structure, whereas F127 induces a worm-like mesoporous structure.48
A number of chemical and structural modifications to sol–gel bioactive glasses have been introduced to achieve improved biocompatibility and mechanical integrity. For example; El-Meliegy et al.277 reported enhanced bone metabolism, in particular osteoblastic proliferation, differentiation and calcification through the presence of iron oxides (below the toxic level in the human body). The authors designed 3D scaffolds by combining Fe2O3-doped sol–gel bioactive glass (SiO2–CaO–Na2O–P2O5) with chitosan and obtained varied scaffold compositions using the freeze-drying technique. It was found that the interconnected open porosity was in the range from 64% to 75%. Furthermore, it was noted that, the presence of Fe2O3 up to 10 wt% led to the reduction in the porosity of formed bioactive glass scaffolds, however, the crosslinking between the bioactive glass and the polymer matrix was increased. In addition to that, the density and the compactness of the scaffolds led to an enhancement of mechanical strength. Besides, the authors also proved that the higher concentrations of bioactive glass and Fe2O3 in the scaffolds increase the cell viability as compared with the normal cells and pure chitosan scaffold.277 In another study, the same group of authors demonstrated that the incorporation of hematite (Fe2O3) in the bioactive glass/chitosan scaffolds leads to an enhanced drug release for delivering 2% chlorhexidine gluconate (CHX).278 Furthermore, the in vitro drug release and antibacterial studies revealed that incorporation of hematite completely removed the bacterial growth after 14 days. Moreover, the scaffold implantation treating dental infections resulted in outstanding osteoinduction ability.278
Recently, attempts have also been made using the sol–gel route to fabricate 3D foam scaffolds as a prominent group of biomaterials for tissue engineering applications. These 3D foam scaffolds can be developed to form hierarchically structured macro and mesopores that can be found in many tissues. The advantage of this type of scaffolds is that they exhibit combined properties of conventional glass-based scaffolds with unique qualities of mesoporous materials. Thus, the fabrication of highly porous 3D scaffolds using bioactive glass composition can be useful in bone tissue regeneration thereby taking advantage of both glass compositions as well as the mesoporous morphology. Baino et al.279 demonstrated excellent apatite-forming ability on iron (Fe) doped sol–gel silicate glasses with multiscale hierarchical porosity ranging from the macro (50–600 mm) to the mesoscale (4–20 nm). It was noted that the pore volume, specific surface area and mesopore size decreased with increase in Fe content. Furthermore, the fabricated foams exhibited promising ferromagnetic properties and high biocompatibility which could be beneficial for simultaneously exploiting their ability to treat bone cancer via hyperthermia thereby promoting bone regeneration.279 Of course, the bone-bonding ability of bioactive glasses depends on biodegradability, the dissolution rate of its ions, formation of new bone tissues and the stimulation of osteoblasts upon soaking in SBF.280 The inclusion of iron oxide is beneficial because it does not induce cytotoxicity and the osteoblast can be grown and proliferated. However, the growth of the HA like layer was slower with increasing iron oxide content. The initial mechanism that was thought to induce bone formation is reduced due to the replacement of Ca2+ with Fe ions in the glass network.280 Mesquita-Guimarães et al.281 emphasized the importance and the influence of macroporosity of bioactive glass on cell viability and proliferation. The authors fabricated zirconia-based open-cell foams using the replica technique followed by coating via immersing in the sol–gel solution of 58S bioactive glass. Fig. 18a demonstrates the SEM micrographs of coated scaffolds immersed three times in fresh sol–gel solution while Fig. 18b show SEM micrograph of coated scaffolds immersed twice during 8 h of condensation.281 It was reported that reducing the pore size from 700 to 322 μm led to a 100% increase in cell proliferation. The enhanced cell viability and proliferation tendency was demonstrated by fluorescence microscopy using 4,6-diamidino- 2-phenylindole (DAPI) and calcein staining of MG-63 cells after two days of cultivation as illustrated in Fig. 18c.281 Another interesting method was reported by Ni et al.282 in which the authors reported about the sol–gel synthesis of bioactive CaO–SiO2–Ag2O glasses loaded with porous anodic alumina (PAA) by a sol-dipping method followed by calcination of the gel-glasses. The results from this study revealed that the combination of the nanoporous structure of PAA and materials impregnated into the pores is a promising technique for forming a coating with multifunctional properties. Fig. 18d shows the typical PAA microstructure consisting of ordered hexagonal cells with a uniform nano-pore in the cell center.282 The results from the pore loading investigation (Fig. 18e and f) revealed that the pore filling can be accomplished using CaO–SiO2 and CaO–SiO2–Ag2O bioactive glasses by a simple mechanism which can be influenced by pressure. Moreover, an in vitro antimicrobial activity test indicated that the CaO–SiO2–Ag2O/PAA system was highly effective in preventing the growth of both Escherichia coli and Staphylococcus aureus bacteria. The enhanced antimicrobial ability is mainly ascribed to the released Ag ions and increased pH values.282 In another study, macroporous nanocomposite scaffolds based on gelatin/bioactive-glass/silver nanoparticles were developed from an aqueous solution of gelatin by freeze-drying method followed by crosslinking using genipin at ambient temperature. From a structural point of view, the macro-scale 3D inter-connected porosity was obtained with pore size ranging from 350 to 635 μm as shown in Fig. 18g–i. Furthermore, by increasing the concentration of silver nanoparticles, the pore size and porosity also increased. As expected, significant prevention of bacterial (Staphylococcus aureus, Escherichia coli) growth and reduction of the biofilm formation on the scaffolds was revealed. Furthermore, the antibacterial effect was improved with an increase in the concentration of silver nanoparticles. It was proved through the viability studies of the hMSC on the prepared scaffold that all the scaffolds were cytocompatible.283 Moreover, Ag ions can be released from sol–gel-derived SiO2–P2O5–CaO–Ag2O glasses depending on the chemical composition, specific surface area and the diffusion of the biogenic ions present thereby affecting the degree of cytotoxicity.284
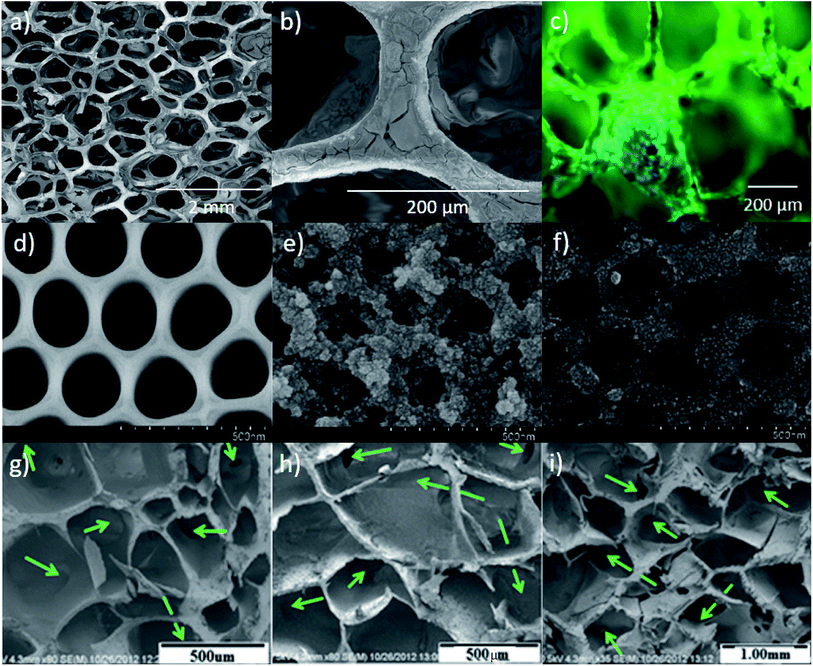 |
| Fig. 18 (a) SEM micrographs of coated scaffolds with three immersions in fresh sol–gel solution. (b) Coated scaffolds with two immersions during 8 h of condensation. (c) Coated scaffolds after 2 days of cultivation. Reproduced with permission from ref. 281. Copyright 2019, Elsevier. (d–f) Micrographs showing surface structure of (d) PAA (e) CaO–SiO2/PAA and (f) CaO–SiO2–Ag2O/PAA. Reproduced with permission from ref. 282. Copyright 2016, Elsevier. (g–i) SEM micrographs of gelatin/nanosilver/bioactive glass scaffolds with different concentrations (g) BGA0% (h) BGA20% and (i) BGA40%. Adapted from ref. 283. Copyright 2014, American Scientific Publishers. | |
In a similar study, Jalise et al.285 reported that gelatin and strontium (Sr) based bioactive glasses fabricated via freeze-drying technique exhibit an interconnected porous structure with an average diameter of 100–300 μm. Furthermore, increasing the Sr concentration leads to a decrease in the average pore size. The fabricated Sr-containing scaffolds with 15% Sr content revealed excellent stiffness (five times greater than glasses without Sr content) and high porosity (>80%). Moreover, in vivo angiogenesis studies confirmed superior cell infiltration and stimulated neovascularization in scaffolds containing 15% Sr ions in the bioactive glass as compared with the glass without Sr content. Shaltooki et al.286 successfully produced a nanocomposite scaffold made of chitosan-coated polycaprolactone (PCL) and 45S bioactive glass (5, 10 and 15 wt%) with 7 wt% Sr using solvent casting technique. Porous nanocomposite scaffolds containing 15 wt% of bioactive glass particles was optimized and coated with chitosan layer which revealed no cytotoxic effect, acceptable degradation behavior and enhanced bioactivity, alkaline phosphatase activity and cell attachment.286 Such modification can be beneficial in controlling the engineering properties of bioactive glasses. Amudha et al.,287 proved that cell viability and proliferation can be significantly enhanced in 45S5 bioactive glass by Sr ions doping. The doping of a small quantity (0.2 wt%) of Sr led to significant changes in the physicochemical, mechanical, surface, drug release and biological properties. For Sr doped glasses, the pore volume and the surface area were improved to 96 and 108% respectively as compared with Sr free glasses. Besides, the interconnected porous structure facilitated the controlled and sustained drug release of about 58% in 720 h. Furthermore, the mechanical strength of the bioactive glass was significantly improved after Sr doping. In another investigation, Zamani et al.288 prepared a composite scaffold based on alginate and bioactive glass (60S) having 80% porosity. The incorporation of bioactive glass particles containing zinc and magnesium into the alginate scaffold led to an improvement of mechanical properties and antibacterial efficiency. Furthermore, the results of in vitro tests exhibited good MG-63 cell response (viability, attachment and proliferation) and osteoblast differentiation. Moreover, the ion release capability of the scaffold was evaluated after 60 days of incubation in PBS solution using inductively coupled plasma (ICP) spectroscopy which revealed enhanced antibacterial efficiency against Escherichia coli and Staphylococcus aureus bacteria.
Hong et al.289 fabricated ultrathin MBG hollow fibers via electrospinning technique by employing high molecular weight polyethylene oxide (PEO) as a phase separating agent. During electrospinning, quick solvent evaporation and PEO stimulated phase separation process played an important role in the development of bioactive glass fibers with hollow cores and mesoporous walls. In another study,290 the fabrication of scaffolds with flexible cotton wool-like 3D structures based on 70 mol% silica and 30 mol% CaO (70S30C) has been reported by combining sol–gel and electrospinning method. The fascinating part of this study was the achievement of three orders of porosity i.e. pores on the fibers, pores between the fibers and pores in the entangled fiber space. Thus, such hierarchical porosity can be useful in enhancing the cellular attachment and the transfer among the scaffold fibers.290 The flexible 3D fibrous structure was found ideal for packing into complex defects with larger inter-fiber spaces to promote vascularization, cell penetrations and nutrients transport throughout the scaffold. The authors demonstrated the potential of 70S30C cotton wool-like materials as a bone graft substitute especially for dental bone regeneration as shown in Fig. 19. The 70S30C based bioactive glass fibers showed excellent flexibility resulting from the large aspect ratio and the entanglement between very long fibers.290
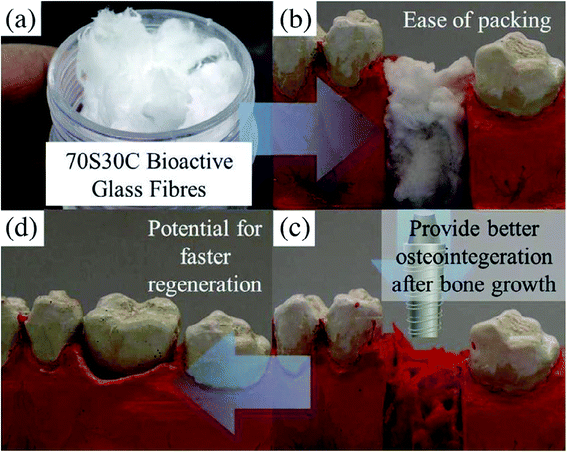 |
| Fig. 19 (a) Photograph showing 70S30C cotton wool like bioactive glass fibers. (b) Demonstration of ease of packing in a tooth extraction socket. (c) Insertion of implant after bone regeneration. (d) Crown placement. Adapted from ref. 290. Copyright 2014, Elsevier. | |
4.3 Chemical compositions
In addition to morphology, the chemical compositions of MBGs are also instrumental in determining their properties and it is one of the key aspects that determine the biological activities of glasses in a physiological environment. Thus, it is attractive to explore new applications of mesoporous glasses based on their composition and structure.67 It has been reported that the sol–gel methods make it possible to study a wider spectrum of glass composition and mesoporous structures than the melt quenching method.1 In general, the composition of the bioactive glasses can be easily adjusted thereby conferring specific properties to the materials which can be effective in fulfilling the demands of a vast variety of biomedical applications.291,292 The sol–gel process significantly expands the range of chemical composition of bioactive glasses, including conditions and preparation protocols. Faure et al.293 reported a sol–gel approach for the synthesis of the 45S5 glass by substituting HNO3 by C6H8O7. The use of C6H8O7 as a catalyst for the sol–gel synthesis of 45S5 powder has been shown to significantly reduce the concentration (5 mM) of acidic solution which is required to catalyze the hydrolysis reactions of TEOS and TEP. It was demonstrated that the in vitro bioactivity level of the sol–gel powder was higher than that of the commercial Bioglass® which can help in speeding the process of bone growth formation by avoiding high pH or temperature conditions.293 The improved bioactivity is associated with the enhanced porosity and the specific surface area of the sol–gel derived powders. Lopes et al.294 demonstrated that the C6H8O7 works as an effective molecular template formed by molecular network elevated from intermolecular forces (e.g. hydrogen bonding) resulting from chemical interactions between the COOH and hydroxyl groups (water, ethanol, P–OH, Si–OH).294 The authors further ascertained that the C6H8O7 assisted sol–gel route is an interesting and promising approach to modify the synthesis protocol to control phase segregation during drying and to achieve high structural homogeneity.294 In another study, Thibault et al.,295 prepared polymer/bioactive glass 45S5 composites comprising boronic acid (PBA) functionalized chitosan by a freeze-drying technique and demonstrated the potential of PBA to improve the bioactivity of the resulting composite. It was ascertained that chitosan–PBA samples exhibit interconnected porosity with pore size ranging from 32 to 476 μm. Moreover, it was revealed that sol–gel modified bioactive glass 45S5 containing PBA exhibit no toxicity for mouse Sertoli (TM4), human embryonic kidney 293 (Hek293) and human bone marrow/stroma (HS-5) cells, as compared with composites prepared using unfunctionalized chitosan.295
The hypothesis behind choosing the chemical compositions of glasses is that the bone comprised collagen fiber, bone cells and HA crystals, the implant material should be capable of forming the HA layer on the surface in the biological fluids.296 Since their discovery, most of the research work being carried out on bioactive glasses is dealing with the compositions that form interfacial bonding with the tissue. The formation of the HA layer on the surface in vitro or in vivo is considered as an indicator of the glass bioactivity.297 The interaction of HA crystals with cellular processes leads to glass bonding with the surrounding tissues. The role of the bioactive glasses in bone tissue regeneration is associated with its proficiency to release the critical concentration of calcium and silicon ions at the rate required for cell proliferation and differentiation.298 Calcium is an essential element of bioactive glasses which plays a pivotal role in inducing osteogenic differentiation of cells.46 The controlled dissolution and the ion release rates along with the glass network structure are the fundamental criteria for designing novel glass compositions for specific applications. The durability can also be adjusted easily from almost inert to quickly dissolving glasses by simply changing the compositions. The wide range of future applications also rests upon adjusting the glass compositions containing specific inorganic ions that can have controlled effects on the cellular process. The release rate of the therapeutic ions, overall glass dissolution kinetics and on surface HA formation depends on the chemical durability of the glass in the target applications.
Recently, tremendous research interest has been shown in designing phosphate and borate-based glass compositions. Hench et al.,296 explored phosphate-containing silicate glasses based on Na2O–CaO–P2O5–SiO2 compositions fulfilling the criteria of tissue bonding. CaO and P2O5 deliver essential constituents of HA (Ca5(PO4)3OH), namely Ca+ and PO43− ions whereas Na2O and SiO2 consist of elements that are abundantly available in the human body. The higher ratio of CaO/P2O5 enables the ion release from the material surface when soaked in body fluid and forms the HCA layer very rapidly. This facilitates cell proliferation on the implant surface by sustaining the ion concentration.296 Borate based glass composition is reported to form HA faster than silicate-based compositions.299 Li et al.93 demonstrated sol–gel derived Na2O–CaO–SiO2 glass compositions which shows bioactivity within far greater compositions than that of melt derived glasses. Since then various compositions of bioactive glasses were thoroughly examined.94–96,98 Fluoride based bioactive silicate glasses were investigated for bone tissue regeneration and also regarded as a potential material for dental applications.99,300 Numerous other oxides such as B2O3, Fe2O3, Al2O3, ZnO, TiO2, BaO, Li2O, CuO and CoO etc. have been included in Na2O–CaO–P2O5–SiO2 glass systems.46,119,301–304 CaO can be replaced with MgO or CaF2 and Na2O can be replaced with K2O without affecting the bone bonding. However, the dissolution rate of the glass can be affected. Furthermore, bioactive glasses were doped with several elements including Ag, Cu and gallium (Ga) to study their antimicrobial properties.162,198,305 Ga doped bioactive glasses have been reported to be promising for wound healing applications.305 The doping effect of the different elements on the cellular processes has been reviewed by several authors.46,306,307 Li et al.308 illustrated that the incorporation of Mg, Zn or Cu as a substitute for Ca2+ affects the glass bioactivity in a sequence of Cu < Mg < Zn. Ag-doped bioactive glasses demonstrate enhanced bioactivity because Ag+ is highly mobile than Na+ thereby it is convenient to exchange with H+ ions.309 Zn and Mg show almost the same effects and they can be useful in preventing bone resorption by activating the proliferation, osteoblasts differentiation and bone mineralization ability.310 Zn exhibits anti-inflammatory properties and can be used in the fabrication of glass-based dental cement and polyakenoate orthopedics showing antimicrobial effects,311 while Mg was found to be useful in promoting bone cell adhesion.312
4.4 Chemical and surface properties
The physical as well as chemical features of sol–gel derived bioactive glasses can be fine-tuned by altering or modifying their surface functional groups. The surface properties of biomaterials play an important role in enhancing their interaction with the surrounding tissues. The surface functionalization of sol–gel based materials is vital in view of their applications in biological fields owing to its advantages such as greater adsorption capacities for biomolecules or drug loading, enhanced inclination to supply these biomolecules to particular targets such as cells or tissues and enhancement in the overall biocompatibility.7 For example; different functional groups can have an impact on the cell adhesion by simply altering the surface hydrophilicity or hydrophobicity.313 The surface functional groups can also influence the protein absorption proficiency of the material and subsequently affects the cell adhesion.314 The surface functionalization of bioactive glasses is a favorable approach to convert the present surface into a more desirable composition or architecture to create opportunities for the preparation of functional biomaterial that can be useful for biological applications.315 Generally, the surface functionalization of bioactive glasses is intended for enhancing the biological response (bioactivity) as well as for improving the biocompatibility of glass particles with other phases.316 The surface functionalization of bioactive glass can be carried out by physical approach (altering surface topography) or chemical/biochemical approaches (adsorption of molecules via atomic layer deposition (ALD), covalent grafting of biomolecules or drugs etc.).317 By employing different functionalization strategies, the surface reactivity of bioactivity glass can be exploited easily to obtain newly customized functional characteristics such as antibacterial, anticancer and antioxidant properties of bioactive glasses.318
Among various strategies described in the literature, silanization is an efficient and widely applied method for covalent modification of bioactive glass surface.319,320 The main purpose of silanization is to establish bonds within the interface between the inorganic constituents and organic biomolecules so as to enhance the bone tissue interaction. Silanization is also used to ameliorate the dispersion stability of inorganic particles in a diverse range of liquids and for anchoring the drug immobilization.320 The reaction conditions such as reaction time, type of solvent, temperature, nature and concentration of alkoxysilanes should be carefully chosen in order to avoid the formation of a polymerized thick network of silane on the surface.320 As a result, the chemical bonds are formed between alkoxysilane and the material surface which can be hydrolyzed under a suitable environment. The hydrolyzed silicon alkoxide contains Si–OH groups condensed with the hydroxyl groups existing on the surface of the material whereas the alkyl chain having functional groups namely carboxyl, epoxy, amine, vinyl, chloro, thiol, cyanide or phenyl are harnessed for further functionalization.320–322 The amino (–NH2) groups are responsible for the electrostatic interactions and covalent bonding with negatively charged functional groups of various molecules such as DNA and protein.320 The most frequently used silane for the surface functionalization and for introducing amino group on the bioactive glass surface is 3-aminopropyltriethoxysilane (APTES) which is also known as a protein coupling or protein binding agent.320 Owing to the plenty of silanol present on the surface of bioactive glass, the surface functionalization using APTES can be achieved within the sol–gel synthesis of bioactive glasses under controlled conditions323 or via adsorption through solution.324,325 Verne et al.324 reported an optimized procedure for surface functionalization of bioactive glasses in order to use the amino groups for binding proteins. Using in vitro test, it was demonstrated that the bioactivity of glass was unaffected when APTES was used as a surface modifying agent.323,326 Moreover, the grafting of APTES has been achieved from various solvents such as toluene,327,328 ethanol324,329,330 and water.326,331,332 Sometimes glutaraldehyde (GA) is also used with APTES for surface functionalization331,333 while the silane molecules can be directly implemented during the sol–gel processing of bioactive glasses.334 In another study, APTES was found useful in promoting the formation of the HCA layer without affecting the bioactivity of glasses.335 Besides, APTES have been successfully utilized for the functionalization of sol–gel derived bioactive glass (58S) in order to improve its cytocompatibility.87
Zhang et al.,336 developed functionalized MBGs which were subjected to the surface functionalization using APTES and triethoxysilylpropyl succinic anhydride (TESPSA). From in vitro studies, it was observed that the synthesized bioactive glasses significantly promoted the proliferation as well as osteogenic differentiation of rabbit bone marrow stromal cells. However, this effect was more pronounced in the bioactive glasses functionalized with APTES than with TESPSA. In vivo studies revealed that the bioactive glasses functionalized with APTES can facilitate a higher level of bone regeneration in comparison to unmodified and TESPSA functionalized bioactive glasses. It was mentioned that the presence of the amino groups on the bioactive glass surface is expected to play a crucial role in enhancing cell proliferation and differentiation.320 The surface amino groups of bioactive glasses are less hydrophilic than carboxyl groups and the surfaces containing amino functional groups with balance hydrophilicity and hydrophobicity are beneficial for cell adhesion. Although bioactive glasses demonstrate favorable bioactivity and biodegradability, the relative brittleness and the lack of in situ mouldability limit their applications. Hence, polymer/bioactive glass composites have been prepared as biomaterials with improved properties.337–340 Recently, bioactive glasses have been reinforced with polylactide (PLA) matrix to develop composites for bone tissue repairing.341 In a similar study, APTES was used as a coupling agent for the surface functionalization of bioactive glasses thereby enhancing the interface between poly(L-lactide) (PLLA) and bioactive glass particles.342 It was reported that the APTES functionalized bioactive glass particles are homogeneously dispersed in the polymeric phase without any agglomeration as compared with non-functionalized glass particles. Aina et al.323 reported new formulations to develop APTES functionalized bioactive glass using maleic anhydride (MA) or cis-aconitic anhydride (CAA) in combination with cysteamine and 5-aminofluorescein as model molecules for stimulating the drug. Fig. 20 schematically represents the procedure for the synthesis of APTES and MA functionalized bioactive glass and models for cell binding and protein absorption.320 In another study, it was described that the GA enables the control over release kinetics of proteins and sustain the native protein structure completely when used as a protein-binding agent.333,343,344 In a similar investigation, it was stated that the surface functionalization of a bioactive glass substrate using APTES and GA was not effective enough to stimulate variations in the methemoglobin and 5-methylaminomethyl-uridine which form enzyme structure.333,344 However, GA is reported to improve the stability of hemoglobin attachment and instigates polymerization on the surface of Ag-doped bioactive glasses.345
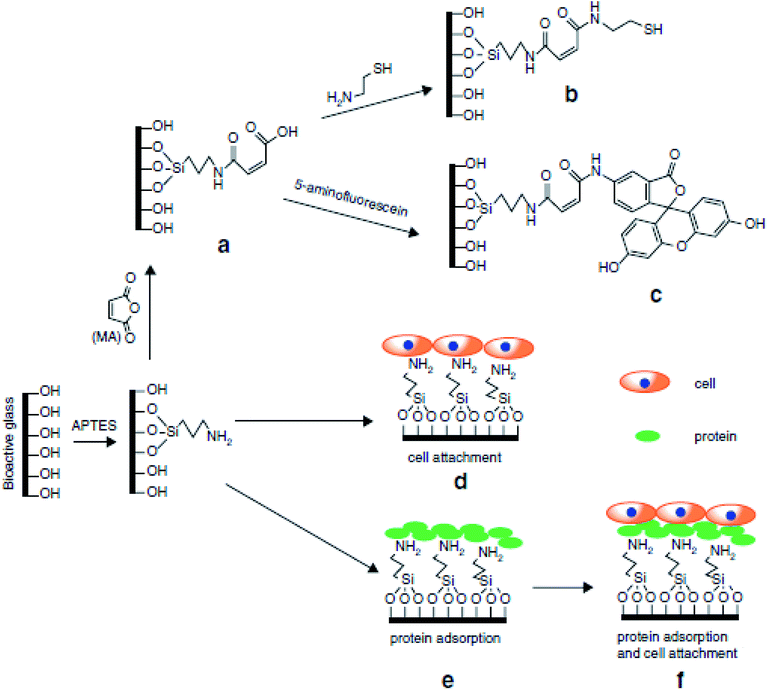 |
| Fig. 20 (a) Procedure for preparation of APTES and MA functionalized bioactive glass. (b and c) Preparation of APTES and MA functionalized bioactive glass with cysteamine and 5-aminofluorescein conjugate. (d–f) Models for cell binding and protein absorption. Adapted from ref. 320. Copyright 2017, Springer. | |
The surface functionalization of bioactive glasses can also be performed by immobilizing various biological species namely proteins and cells on their surfaces. For example; surface functionalization using proteins enhances the bone integration of bioactive glasses. The biomolecules can be bonded to the bioactive glass surface by either electrostatic and van der Waals forces (weak physical interaction) or by covalent or ionic bonding.320 The binding of biomolecules can be affected by various physicochemical characteristics such as chemical composition, pH or dissolution behavior, microstructure, crystallization degree, hydrophobicity, surface reactivity, surface roughness, zeta potential and particle size etc., of bioactive glasses.346 Furthermore, the grafting of biomolecules onto the surface of bioactive glasses was studied by various researchers to improve their bioactivity, cell adhesion and differentiation for bone regeneration application. For this purpose, collagen,332 lysine,347 ipriflavone,327 soybean peroxide,348 bovine serum albumin,327,349,350 α-amylase,327,349 BMPs,324,351 alkaline phosphate (ALP)325,329 and commercially available mixture of enamel matrix proteins (Emdogain®)352 have been considered. Laminin was adsorbed on the APTES surface-functionalized bioactive glass foams and its sustainable and controlled release from modified scaffolds was achieved over a 30 day period making it beneficial for tissue formation.353 Furthermore, antibiotics and anti-inflammatory agents such as gentamicin,354,355 tetracycline356 and ibuprofen357 have been loaded into MBGs forming biomaterials for localized drug delivery applications. Surface functionalized bioactive glasses have also been reported for localized cancer treatment and for this purpose, various biomolecules and drugs have been proposed such as 5-aminofluorescein for doxorubicin,334 doxorubicin and cisplatin,358 dexamethasone,350 alendronate,359 curcumin360 and gallic acid.361,362 Another important approach for surface functionalization of bioactive glasses is the induction of apatite formation. For this purpose, bioactive glass has been soaked in different solutions to facilitate the CaP layer formation on its surface.363 Studies have demonstrated that the functionalization of the bioactive glass surface by bio-mineralization of the CaP layer followed by immobilization of particular proteins can improve cell adhesion, proliferation and differentiation.364 Thus, all the aforementioned studies demonstrate that various strategies can be employed for exploiting the surface reactivity of bioactive glasses for their wide range of biomedical applications.
4.5 Biological properties and biodegradation
Biodegradability is among the most significant characteristics of sol–gel derived bioactive glasses in view of their bone-bonding ability. Generally, biodegradable materials manifest higher reactivity which is closely associated with the formation of the HCA layer.365 Biodegradation is the key when bioactive glasses are utilized for the preparation of bone tissue scaffolds. Nevertheless, high degradation rates have a negative impact on cell attachment and growth and therefore prevent the progress of new bone formation.366 Thus, it has been suggested that the surface functionalization of bioactive glasses using organic functional groups (amino or carboxylic groups) can partially decrease the high degradation rates while maintaining the bioactivity. For a variety of applications, materials that degrade easily are best suited than persistent implant materials because degradable materials are resorbable into the body and can be exchanged with regenerated tissues.367 Nevertheless, managing the degradation rate is a complex process; therefore, it is necessary to understand the degradation mechanism and the determinants that affect the degradation rate of sol–gel based bioactive glasses.1 Moreover, the dissolution of glass in contact with the body fluids and the release of Ca and P ions are crucial to the bio-mineralization of glasses. The ionic dissolution products from bioactive glasses are well known for stimulating angiogenesis, osteogenesis and vascularization.368 Thus, the dissolution behavior plays a pivotal role in the bioactivity of the sol–gel glasses.
Recent research has remained focused on the molecular interaction of bioactive glass-based ionic dissolution products with their physiological surroundings so as to understand such processes to formulate smart bioactive glasses with customized properties specifically for tissue engineering. Fig. 21 gives an outline of biological responses towards bioactive glass-based ionic dissolution products. In the context of tissue engineering, numerous literature evidence indicates the importance of ionic dissolution products in understanding the behavior of sol–gel derived bioactive glasses in vivo and in vitro. A large number of trace elements including Sr, Cu, Zn, Co etc., present in a human body are well renowned for their growth-promoting effects in bone metabolism and play a major role in angiogenesis, growth and bone tissue mineralization.46 Therefore, novel research avenues are being investigated for enhancing the glass bioactivity by introducing these therapeutic ions in different bioactive glasses resulting in the modification of their dissolution behavior and improved biological performance.46 Furthermore, innovative methodologies have been suggested for advancing the fields of bioactive glass scaffolds by inducting active metal ions into the glass network for utilizing the therapeutic effects of such ions and to ameliorate the biological functioning of the materials as regards to the response of the specific host.46 As stated earlier, Ca and P are the main component of the inorganic phase of the human bone, these ions play an integral part in bone formation and resorption. However, one should know the specific ECM concentration of these ions and their interaction mechanism with bone cells and exhilarating effect on bone formation.46 This information can be useful in designing advanced scaffolds with controlled biological properties in a suitable physiological condition with tunable ion release kinetics.46
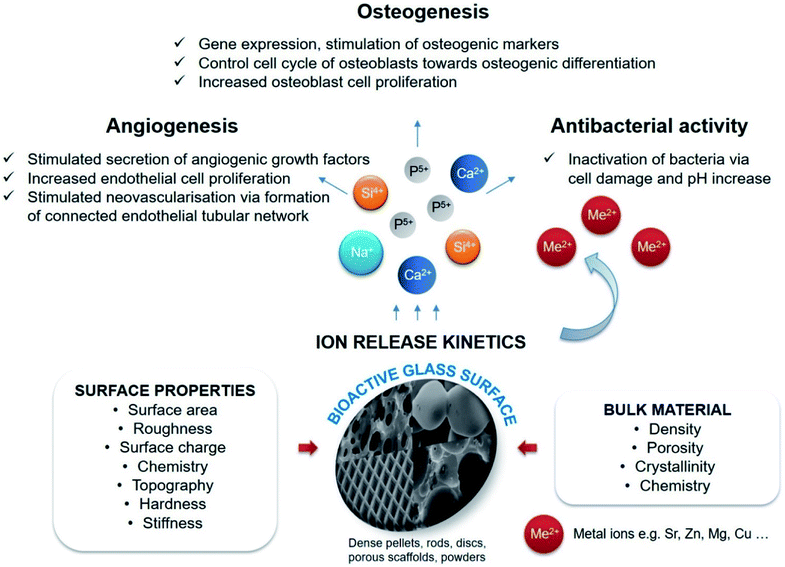 |
| Fig. 21 Schematic outline of biological response to bioactive glass based ionic dissolution products. | |
Bioactive glasses require specific dissolution rates in order to tune their in vitro and in vivo performances. The dissolution testing is generally performed by soaking the bioactive glasses in SBF, tris buffer solution (TBS), phosphate-buffered saline (PBS) and cell culture media.369–371 If the dissolution rate is very slow, the ionic concentrations are insufficient to promote cell proliferation and differentiation. On the other hand, if the rate of dissolution is too quick, the ionic concentration might be beyond the effective level.110,372 Moreover, the ionic concentration and the pH of SBF are the same as that of human blood plasma (HBP).373 Therefore, SBF is the most common solution used for soaking bioactive glasses under static conditions. Besides, the soaking of bioactive glasses in SBF is a very simple, cost-effective and rapid method of assessing the probable bioactivity. In SBF, an in vitro test could be performed in a few minutes after immersion. Hence, this procedure has been widely adopted as a basis for assessing biomaterial for bone tissue regeneration. The first step in the bioactivity mechanism is the resorption in which the bioactive material undergoes a rapid chemical reaction when in contact with physiological fluids. When soaked in SBF, the bioactive glasses exchange ions with SBF and form silanol groups on the surface thereby nucleating the HA layer.374 The formation of the HA layer takes place via two steps namely (i) formation of dicalcium phosphate (CaHPO4) by combining calcium and phosphorus ions in SBF and (ii) the conversion of CaHPO4 into HA or TCP (Ca3(PO4)2) via sequential reaction.374 As reported, the higher concentrations of silanol groups result in a quicker dissolution or bioactive response and this valuable information can be useful in designing porous sol–gel glasses having a high surface area and high silanol concentrations.93,375 It was shown that specific heat processing and relative proportions of CaO to SiO2 are the decisive factors to obtain these porous glasses with prosperous bioactive behavior.376 To date, several authors have reported the impromptu formation of the HA layer on bioactive glass surfaces comprising CaO–SiO2–P2O5 ternary systems when contacted with the biological fluids.377–379 The real developments came in regards to bioactivity kinetics with the developments of MBGs because of the high surface area and high mesoporosity, both features facilitate enhanced reaction degree between the glass and the biological fluids.380Fig. 22 compares the formation of a new apatite layer on the surface of the traditional sol–gel glass (left) and MBG (right) when in contact with the biological fluids.381 Considering that both Ca and P are the major components of the apatite layer and the inorganic phase of human bone, it is accountable for profound bonding between bioactive glass and human bone.118,381
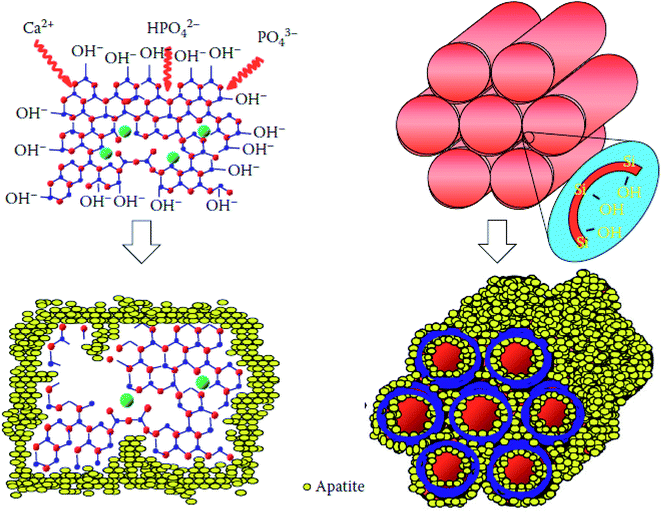 |
| Fig. 22 Demonstration of the apatite layer formation on the surface of traditional sol–gel glass (left) and MBG (right) after contacting the biological fluids. Adapted from ref. 381. Copyright 2012, CRC Press. | |
The chemical reactions occurring on the glass surface are derived from leaching, dissolution and precipitation.110 Based on such interfacial reactions, the cellular reactions give rise to the development of a chemical bond between living tissues and the bioactive glass.131 The first prompt reaction transpiring on the glass surface after implantation in vivo or soaking in SBF is the cation replacement of Ca2+, Na+ or K+ with H+ or H3O+ from the solution.382 Simultaneously, the solution pH increases. The leaching reaction generates an alkaline microenvironment wherein the solution alkalinity is emerged due to breakage of –Si–O–Si– bonds mostly by hydroxyl ions. The dissolution takes place at the glass surface and leads to the establishment of silanol (Si–OH) groups at the interface between glass and the solution. The condensation and re-polymerization of hydrated silica groups occur with the silanol group resulting in the formation of SiO2 rich layer (silica gel) on the surface. Such precipitations are promoted by the migration of Ca2+ and PO43− ions to the surface within the SiO2 rich layer to create an amorphous CaP layer at the top of the SiO2 rich layer.298 The amorphous CaP layer expands due to the inclusion of soluble CaP from the supersaturated solution.131Fig. 23 demonstrates different stages of surface reactions along with the generation of Si-rich and CaP layer at the bioactive glass surface.382 The thickness of the SiO2 rich layer increases as a result of diffusion-controlled alkali ion interchange whereas the final thickness depends on its composition. The crystallization of the amorphous CaP layer takes place by the inclusion of OH−, CO32− or F− anions from the solution to form the HCA or apatite layer substituted by fluoride ions (F-HCA) probably through similar reactions which facilitates the crystallization of Ca deficient apatite layer.382 As the apatite crystals composition is analogous with the inorganic mineral phase of bone, such reaction steps are most often utilized for characterizing biomaterials which form interfacial tissue bond. In an ideal case, the reaction proceeds until the bioactive glasses are completely substituted by new tissue.298
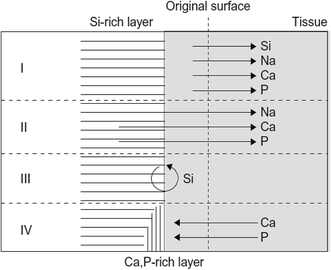 |
| Fig. 23 Different stages of surface reactions and the development of Si-rich and CaP layer at the bioactive glass surface. (I) Initially, a glass layer with few micron thickness is dissolved. (II) Later, Na, Ca, P and Si are leached out from the glass surface. (III) Si-rich layer is developed via repolymerization. (IV) Ca and P from the solution are partly leached out from the glass precipitate on the Si-rich layer. Reproduced with permission from ref. 382. Copyright 2018, Elsevier. | |
There are several ways of investigating the dissolution rate of biomaterials and one of the simplest methods is the measurement of weight loss. The dissolution rate of biomaterials can also be evaluated by quantifying the released ions concentration and the pH shift within the solution. The pH variation is greatly reliant on the capability of the solution to buffer or the local environment in vivo.370,371 As mentioned before, the dissolution reaction takes place at the interface between the surface of the bioactive glasses and the solution. Hence, the specific surface area is a critical parameter that can influence the degradation rate.383 The high surface area is responsible for the high dissolution rate in sol–gel synthesized mesoporous glasses in comparison with the melt derived glasses which are relatively dense.370 Furthermore, with an increase in pore size, the solution circulation within the pores becomes easy causing an enhanced reactivity.383 The sol–gel derived glasses frequently undergo thermal treatment to eliminate the organic phase or to improve the stability and as a consequence, the porosity and the crystallinity of glass get affected.114 Cacciotti et al.384 evaluated the dissolution rate of sol–gel derived bioactive glasses heat-treated at different temperatures. The crystalline phases were observed when the glasses were heated at 1100 °C and very slow dissolution behavior was observed as compared with the glasses heated at 700 °C. These findings demonstrated a low degree of glass crystallinity when heated at low temperatures. Besides, the dissolution behavior of sol–gel based bioactive glasses is not only affected by the aforementioned conditions but also depends on the surrounding conditions namely solution temperature, pH and composition.1 For example; the solution temperature substantially influences the degradation behavior of sol–gel synthesized silica glasses.385 A similar trend was also observed in phosphate-based glass systems.386 The solution pH also has an impact on the dissolution behavior of sol–gel derived glasses. For example; for silica glasses, the dissolution rate was enhanced with an increment in pH. This is because the Si–O–Si bonds are destroyed due to the nucleophilic attack of OH− in water containing medium.387 The dissolution rate is generally increased with an increase in pH from 2 to 8.5 because when pH > 8.5, ions which are more soluble (SiO(OH)3−) are being developed.388
The composition is also an equally important factor that helps in determining the dissolution rate of the glass. For example; Ma et al.60 reported that with partial substitution of MgO for CaO in the composition, the glass degradation rate decreases with the delay in HCA layer formation, which was ascribed to the effect of ionic field strength and diverse bonding configurations of glass. In another study, very rapid dissolution rates were observed for glasses without CaO in SBF.389 However, the dissolution rate decreases as CaO content increases and P2O5 content decreases attributing to the soluble glass species such as Na+, Ca2+ and HPO42−. In TBS there are no Ca or P sources; therefore, the apatite layer is generated only by releasing the Ca and P ions from the glasses. On the contrary, in SBF or PBS, the generation of the HCA layer is expedited and further, dissolution is restrained as the number of Ca and P ions are accommodated in these solutions.371 In addition, the biomolecules present in the solution also affect the dissolution of bioactive glasses. For example; Sepulveda et al.370 performed a comparative assessment on the dissolution behavior of bioactive glasses in the SBF solution and the culture medium. From ICP mass spectroscopy studies, it was observed that the dissolution rate of bioactive glasses was faster in SBF solution than in culture medium due to positively charged serum proteins which can be adsorbed on the surface of the material and suppress the glass dissolution. Thus, the above discussion gives a consensus view that the biocompatibility of biomaterials in the physiological environment upon implantation should be investigated. However, the factor pertaining to the degradation of biomaterial itself is one of the aspects along with the equally important factors such as the influence of materials on its in vivo environment and bioactivity needs to be considered.1
4.6 Mechanical properties
Sol–gel derived bioactive glasses are often characterized by lower mechanical resistance to dense glasses of the same compositions due to their inherent highly porous structures.1 For example; the flexural strength of TMOS based silica aerogels with 95% porosity was reported to be 0.02 MPa390 which is about 0.0002% of the flexural strength of dense silica material.391 The low mechanical strength is the key issue that is specifically serious when sol–gel derived glasses are processed as hierarchical porous scaffolds with multiscale porosity.392 It is common knowledge that bone exhibit attractive mechanical properties due to its hierarchical structure and the sol–gel glass-ceramics exhibits lower toughness in comparison with the natural load-bearing bones. Therefore, it is essential to formulate new strategies for improving the mechanical behavior of sol–gel glasses to be used as coatings or in a bulk state for bone grafting. The bioactive sol–gel glass compositions need to be modified to yield improved mechanical properties. Studies have shown that the mechanical properties of sol–gel glasses can be substantially altered by varying few processing parameters such as the type of precursor, molar ratio of water to the precursor, pH and doping agents etc.,.1 The type of precursors used for the sol–gel synthesis influences the sol–gel reactions and ultimately affects the mechanical properties of the final material. For example; it has been reported that TEOS and TMOS derived silica aerogels with the same porosity of about 50% exhibited different mechanical strength.393 Hence, irrespective of the relatively higher strength or modulus, the brittleness issue limits the wider applications of sol–gel materials.
The doping agents or additives used during the sol–gel synthesis are also accountable for the mechanical properties of the final material. For example; substituting Si or O in the silica network with other elements can enhance the mechanical properties of sol–gel derived silica glasses.1 For example; the effect of different additives such as MgO, TiO2 or CaF2 on the mechanical properties of sol–gel silica glasses was investigated by substituting Mg2+ and Ti4+ for Ca2+ or F− for O2−.394 The ionic strength of Mg2+ is greater than the ionic strength of Ca2+ ions. Thus, the substitution of Mg2+ for Ca2+ strengthens the glass network owing to reduced bond lengths.104 Likewise, the substitution of Ti4+ for Ca2+ ions enhances the glass strength by connecting with more O2− ions (Ti4+ ions can connect with four O2−).395 Consequently, Ti4+ and Mg2+ substituted glasses demonstrated improved hardness, bending strength and fracture toughness as compared with Ti4+ and Mg2+ free glasses.394 On the other hand, as a result of substituting CaO by CaF2, the F− ions disrupted the glass network and increases the crystallization tendency. Hence, CaF2 incorporated sol–gel glasses do not possess advantageous mechanical properties.1 Improved mechanical properties were reported by the incorporation of alumina, barium and calcium in the glass composition.396–398 Barium ions exhibit a larger ionic radius than silicon ions which allows the formation of a denser network in the glass structure. Barium addition increases the flexural strength of sol–gel glasses.397 The silicon and aluminum acted as a network forming elements in the sol–gel synthesis of bioactive glasses and improves compressive strength and Young's modulus respectively.396,398 The mechanical resistances were decreased when silicon content was decreased.397 Yang et al.399 incorporated B2O3 in CaO–SiO2–P2O5 bioactive glasses to improve the compressive and flexural strength of porous glass-ceramics. Ben Arfa et al.400 reported mechanical properties of Cu2+ and La3+ doped silica sol–gel glass scaffolds with the composition 67% SiO2–24% CaO–5% Na2O–4% P2O5. The compressive strength values were reported for the sintered scaffolds of varied pore sizes (300, 400 and 500 μm). A small enhancement (7–18%) in the compressive strength of La3+ doped glass was observed as compared with undoped glass. On the other hand, considerably higher enhancement in the compressive strength up to 221% was noticed with the addition of Cu2+ ions as compared with the undoped silica glass. The high values of compressive strength for Cu2+ doped glasses as compared with La3+ doped glass is ascribed to their different ionic radius. The small ionic radius and low valence of Cu2+ in comparison to that of La3+ confer higher thermal diffusivity post sintering making Cu2+ serve as an efficacious sintering assistant resulting in enhanced densification and mechanical properties.400 The maximum compressive strength value being reported is ∼14 MPa which is around the upper limit registered for human cancellous bone (2–12 MPa).401 The lower value of compressive strength was noted for the scaffolds with the largest pore size (500 μm) and the largest value of compressive strength was noted for the scaffolds with the smallest pore size (300 μm). In another investigation, an enhanced bulk, shear and Young's modulus were observed with increased CaO/P2O5 molar ratio in SiO2–CaO–Na2O–P2O5 glasses.402
The mechanical performance of sol–gel based glasses can also be improved by decreasing the pore size during the sintering process.403 Nevertheless, there are two limitations, (i) high-temperature sintering can result in vanishing of mesopores due to densification thereby making material unsuited for drug delivery applications (ii) if the biomolecules are included into the material before sintering, then the temperature needs to be optimized at a certain level to preserve biological molecules from degradation and to prevent uncontrollable crystallization process.1,233 The synthesis method also affects the mechanical properties. For example; sol–gel synthesis of bioactive glass foam delivers an enhanced porosity but a low compressive strength.404 A maximum compressive strength exceeding 5 MPa was achieved recently for sol–gel glass foams by maintaining the interconnected porous network required for vascularized bone ingrowth.403 This was achieved by optimizing the sintering process of the sol–gel foam. However, small changes in compressive strength were noticed after immersion of sol–gel glasses in SBF for several days. Jones et al.405 noted an increase in the compressive strength of 70S30C sol–gel derived glasses with an increase in the sintering temperature i.e. the compressive strength of 0.36, 0.51, 2.26 and 2.25 MPa were obtained at sintering temperature of 600, 700, 800 and 1000 °C. The compressive strength of 2.26 MPa was obtained for the scaffolds which ascribed to the decrement in the textural porosity and thereby increasing the density of the macropore wall at the sintering temperature of 800 °C. With the increase in sintering temperature to 1000 °C, the compressive strength was found to be constant although the textural porosity was disappeared. This was attributed to the changes in the glass structure as the crystallization onset temperature was increased.405
Vallet Regi et al.406 investigated the mechanical properties of glass-ceramics prepared from sol–gel glasses with a composition of 55 mol% SiO2
:
45 mol% CaO (55S45C). The mechanical properties of 55S45C were compared with the glass-ceramic containing the same amount of SiO2 but with 4% content of P2O5 (55S41C4P). In addition, the samples were examined in order to ascertain the influence of the sintering process on the mechanical properties. It was observed that the flexural strength (S) and the Weibull (m) coefficient values are higher for the phosphorous free glasses. A significantly high value of S was observed for the glasses sintered at 1100 °C (55S45C-1100). Furthermore, the S values were increased with an increase in the temperature although the increase was not significant from the statistical point of view. However, in 55S41C4P glasses, a two-fold increase in S values with sintering temperature was observed even though these S values were lower than that obtained for 55S45C glasses. Li et al.407 evaluated the mechanical properties of macroporous sol–gel bioactive glasses with the compositions of 58 wt% SiO2–33 wt% CaO–9 wt% P2O5. These sol–gel glasses demonstrated compressive strengths higher than the human cancellous bone.401 The high values of compressive strengths are beneficial in withstanding physiological stresses and minimizing stress shielding in the surrounding host bone.408
Sol–gel glasses together with organic polymers can enhance the mechanical strength and toughness of the resulting hybrid.409,410 The integration of silica IPNs within the polymer matrix at the molecular level is anticipated to improve the mechanical properties of bioactive glasses. Recently, the mechanical properties of porous scaffolds based on bioactive silica–poly(γ-glutamic acid) (PGA) hybrids have been studied.411 The authors hypothesized that by increasing the covalent coupling, the inherent mechanical properties such as compressive strength and toughness of hybrids can be improved. The elongation to failure was increased from 4.2% for the sol–gel glass to 27.5% for the hybrid monoliths. This indicates an improvement in the strain to failure and hence enhanced toughness. However, the compressive strength of the hybrids was low as compared to the sol–gel glass and to that of cortical bone.412 Nevertheless, in the context of increased toughness, elongation and extended elastic behavior are highly promising. The elastic modulus, compressive strength and elongation to failure were also reported for the hybrids in response to increased covalent coupling. The lowest coupling showed the minimum compressive strength and elongation to failure. Further increasing the covalent coupling increases the compressive strength, elastic modulus and elongation to failure.411 In another work, Li et al.413 investigated the mechanical properties of PEG/SiO2–CaO hybrids. The hybrids were prepared with IPNs of silica and PEG of two different molecular weights (PEG300 and PEG600) via the formation of Si–O–Si bonds. It was observed that the elongation to failure was greatly increased from 4.2% for sintered sol–gel glass monoliths (70S30C, 800 °C) to 35% for the hybrids. It was also noted that the mechanical properties were enhanced with an increase in inorganic ratio. Moreover, Young's modulus and compressive strength are found highest for the 50
:
50 compositions of the hybrids. The overall trend observed was 50
:
50 > 60
:
40 > 70
:
30 for hybrids containing both PEG300 and PEG600. Another aspect is that Young's modulus and compressive strength are greater for PEG600 hybrid as compared to PEG300 hybrid with the same composition. The PEG600 hybrids with 50
:
50 compositions were found to exhibit the best mechanical properties among other compositions tested. In another investigation, Bossard et al.414 studied the mechanical properties of hybrids comprising PCL and SiO2–CaO bioactive glasses. Adding PCL effectually introduced toughness to sol–gel glasses. However, the hybrids showed low stiffness as evident from their elastic modulus (0.49 ± 0.03 MPa) and yield strength of 0.036 ± 0.003 MPa. These values are significantly lower than that of trabecular bone with a similar porosity.415,416 The low stiffness of the scaffolds can be assigned to the highly flexible PCL in comparison with other bioresorbable polymers417,418 and due to weak interaction between organic and inorganic phases.62
Sol–gel hybrids based on silica IPN and biodegradable polymeric materials can result in the combination of properties such as biological activities of glasses and the toughness of the polymer matrix. Recently, Valliant et al.369 studied the mechanical properties of silica/γPGA sol–gel hybrids. Ca was incorporated into PGA by chelation while the Ca salt form of PGA (γCaPGA) was synthesized and used as calcium source as well as the biodegradable toughening component of the hybrids. The compressive strength greater than 300 MPa and the strain to failure of >26% was observed for the silica/PGA hybrids which is a considerable improvement as compared with the sol–gel glasses which were pretty brittle with the strain to failure of about 4% and the compressive strength of 66 MPa. It has been suggested that the incorporation of γPGA into the sol–gel process resulted in the elimination of brittleness of the resulting glasses. For the hybrids synthesized with γCaPGA, the maximum compressive strength was observed to be 540 MPa which is far greater than the compressive strength of cortical bone.419 Young's modulus value of the same hybrid was found to be 1.9 GPa which is far lower than Young's modulus of cortical bone.420 Chung et al.421 reported mechanical properties of star-shaped methacrylate-based polymer–SiO2 hybrids exhibiting the modulus of toughness 9.6 fold greater and Young's modulus 4.5 fold lower than the sol–gel based bioactive glasses. The main aim of this study was to ascertain the effect of polymer architecture on mechanical properties and its influence on bone cell attachment. Thus, all the aforementioned studies demonstrate the mechanical integrity of sol–gel based bioactive glasses as a potential biomaterial for bone tissue regeneration.
5. Sol–gel derived porous glasses for bone tissue regeneration
Tissue engineering has aroused tremendous interest as a prospective research field for repairing and regenerating tissues and organs which are damaged or lost due to trauma, injury or disease.119 The main purpose of tissue engineering and regeneration of medicine is to regrow unhealthy or impaired tissue using a combination of cells, bioactive agents and biodegradable scaffolds.380 The most common approach in tissue engineering is to use the biomaterials based scaffold with a specific architecture which can serve as a provisional structure for cells and leads to their proliferation and differentiation into an appropriate tissue or organ.119 Along with the cells, biomolecules such as growth factors can also be included in the scaffold to regulate the cellular functioning during tissue or organ regeneration.422,423 Dramatic growth in the tissue engineering fields has been witnessed in the past two decades and this became possible only due to innovative design and synthesis of biomaterials and fabrication of scaffolds.119 A scaffold is an essential constituent in tissue engineering for bone regeneration as it works as a template for cell interactions and the establishment of ECM of bone providing structural support to the newly formed tissue.424 The scaffold is utilized to control growth factor and cell delivery in addition to providing the structural template to load the tissue lesion.425 Preferably, the scaffold should assist in cell infiltration, cell attachment, matrix deposition and should be consists of osteoconductive mediums such as bone protein and HA providing load-bearing and initiating osteogenesis. Furthermore, the scaffolds created using biomaterials are formulated to meet the rigorous requirements which are imperative or desired for optimum tissue formation.426 The scaffold architecture is also essential in furnishing the cells with an optimized microenvironment to develop new tissue and to grant flow or dissemination of nutrients within the cells and the surroundings.426
Bioactive glasses are one of the subsets of biomaterials that have a profound influence on tissue engineering especially for hard and soft tissue regeneration because they fit perfectly into this land space as materials for producing functional 3D scaffolds. Bone tissue engineering is among the most promising medical applications of bioactive glass and glass-ceramics due to their excellent osteoconductive and angiogenic properties, mechanical strength and good degradation rates.427 By virtue of these properties, both bioactive glasses and glass-ceramics have been used in bone tissue regeneration applications over the past few decades.428–430 Always, the spotlight is on the development of new bioactive glasses and converting them into scaffolds with necessary shape and architecture.119 The tissue regeneration ability of bioactive glasses can be assessed based on their properties namely osteogenesis, osteoconductivity and osteoinductivity.431 The osteogenic potential of bioactive glasses is evaluated on the basis of cells such as mesenchymal stem cells (MSCs), osteoblasts and osteocytes involved in bone formation. The osteoconductivity is the process where scaffolds or matrix excites growth of the bone cells on its surface and osteoinductive capability can be referred as the excitation of MSCs to distinguish preosteoblast, thus beginning the bone-constructing process.431 Also, for bone tissue engineering, large interrelated pores and high porosity percentages are found imperative for attaching and growing bone-forming cells and further ingrowth of vascularized tissue.432 The surface nanoporosity was also found to foster adhesion of cells to biomaterials433 and bone ingrowth in bioactive glass scaffolds.434 The most important requirements of fabricating scaffolds for bone tissue engineering that can be capable of inciting the tissue growth are;62,96,432,435–437
(i) Biocompatibility: the scaffold has to be biocompatible, bioactive and must assist in the attachment, proliferation and cell differentiation in vivo and must not deliver any toxic by-product or persuade any harmful inflammatory reactions.
(ii) Osteogenesis: the scaffold should have the potential to bond with the host bone (osteoconduction) as well as must prevent the encapsulation of fibrous tissue. The scaffold must recruit cells into the implantation site which can be further differentiated to create new bone.
(iii) Biodegradability: the scaffold needs to be degraded at the same rate at which the new tissue is formed at the implant site and ultimately must be remodeled by osteoblast action.
(iv) Mechanical strength: the mechanical strength of scaffolds should be equal to that of bone tissue to be replaced. The scaffold meant for regeneration should provide support at the time of degradation and tissue regeneration.
(v) Porous microstructure: an ideal scaffold should have high porosity (>80%) with interrelated pores. A hierarchical porosity with a minimum diameter of 100 μm is the requisites for cell penetration, tissue ingrowth and neovascularization.
(vi) Fabrication: the scaffold should be easily produced into irregular shapes that can mimic the bone defects of an individual patient.
(vii) Commercialization: the fabricated scaffold should possess the commercialization potential. The sterilization should be carried out by following the international norms and regulations for their use in biomedical devices.
Generally, the scaffolds for tissue engineering are fabricated using both synthetic and natural polymers which are biodegradable.438,439 Biodegradable scaffolds facilitate primary structure and stability for forming a tissue but degrade as the tissue is formed. This provides a base for the deposition of matrix and tissue growth.440,441 However, the main hurdle in designing scaffolds for tissue engineering is that the majority of the materials used for this purpose are usually bio-inert and the degradable materials are often mechanically weak. Therefore, the preparation of composites containing biodegradable polymer systems and the bioactive glass becomes a feasible alternative to accomplish the need for bioactivity, degradability and mechanical properties.432 For instance; Bossard et al.414 developed scaffolds from PCL/bioactive glass hybrids for bone tissue regeneration. The scaffolds obtained from PCL/SiO2–CaO bioactive glass hybrids were highly flexible indicating that PCL has effectively introduced the toughness. The apatite formation was observed over 24 hours of soaking in SBF and the entire hybrid was changed steadily into bone-like minerals. The in vivo study confirmed the bioactivity, biodegradability and an appropriate degradation rate of the hybrid. Furthermore, efforts have also been undertaken to integrate biodegradable synthetic polymers in a biocompatible inorganic phase, for example, most frequently HA and TCP.442,443 Chen et al.444 studied the effect of Ca in the CaO–SiO2–TiO2 glass system modified by PDMS and obtained dense and homogeneous monoliths. In this study, Ca was found to excite apatite like phase on the surface of bioactive glasses when soaked in the SBF solution for about two days. These hybrids were structurally described as the network of silica–titania covalently bonded with PDMS where Ca ions are bonded ionically. An increase in the mechanical properties of the hybrid was observed owing to the existence of PDMS while Ca promoted the generation of HCA layer by supplying physical sites for HCA nucleation resulting in an improved in vitro bioactivity. In another investigation,445 a 3D porous scaffold comprising polylactide-co-glycolide (PLAGA) and bioactive glass composites has been developed. The PLAGA–bioactive glass composites were prepared as 3D porous scaffolds as well as thin films as shown in Fig. 24.445 The scaffolds were found to be biodegradable, bioactive and suitable for bone tissue engineering. The soaking test was performed in SBF to assess the bioactivity of the composite where the CaP layer was formed within seven days. Moreover, it was found that the bioactive glass particles promoted the expressions of collagen (type I) by human osteoblast-like cells. The control PLAGA scaffold displayed 31% total porosity with a mean pore diameter of 116 μm while PLAGA–bioactive glass composite exhibits 43% total porosity with a mean pore diameter of 89 μm. Furthermore, the mechanical properties of the PLAGA–bioactive glass composite scaffold were investigated and an increase in the elastic modulus up to 51.336 ± 6.1 MPa was observed as compared with the elastic modulus (26.479 ± 3.4 MPa) of control PLAGA scaffold. All these findings point towards bioactive glasses as fascinating bioactive material to foster the bioactivity of polymer-based composites for prospective bone regeneration applications.
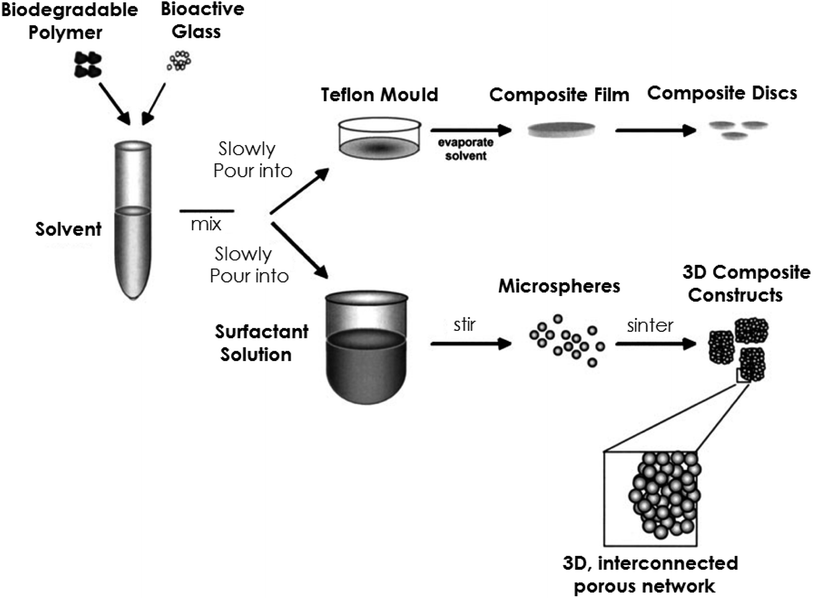 |
| Fig. 24 A schematic representation of the fabrication process of PLAGA–bioactive glass composite scaffolds. Reproduced with permission from ref. 445. Copyright 2003, John Wiley & Sons. | |
The significance of hierarchical scaffold porosity for bone tissue regeneration has been discussed by numerous researchers.432,446 Porous structures having a total open porosity greater than 80% have proven to be efficient in promoting tissue ingrowth given that they allow cell migrations, proliferation and deposition of the matrix in the open spaces.447 The existence of an interrelated pore network facilitates the invasion of blood vessels and the supply of nutrients.446 Also, the average pore size can be instrumental in bone tissue engineering where small pores prevent migration of cells and restrict the diffusion of nutrients and elimination of waste products448,449 while very wide pore restricts the cell attachment as a result of the decrease in the specific surface area.450 MBGs display a highly ordered pore structure with a pore diameter in the range of 2–10 nm having a much-closed pore size distribution. The large architectural features and the existence of silanol groups are the main reason behind the quick in vitro bioactive response of certain compositions of MBGs.451 Furthermore, MBGs are accepted as a very appropriate vehicle for controlling drug release and growth factors which can be embedded inside the pores. Fig. 25 schematically represents the important features of MBGS which make them an ideal candidate for the synthesis of 3D mesoporous scaffold for bone tissue engineering, local drug delivery of biomolecules, drugs, therapeutic ions as well as for designing and developing stimuli-responsive systems.382 Considering the properties of scaffolds for bone tissue regeneration, various strategies such as foaming, free drying, fiber bonding and rapid prototyping techniques have been proposed for designing mesoporous structure required for cell functions namely bone cell ingrowths, the supply of nutrients, vascularization and also for adhesion and growth of bone cells.452 However, it is necessary to keep in mind that the powder processing of MBGs for scaffold preparation using the above-mentioned techniques should not affect the ordered mesoporosity and bioactivity.453
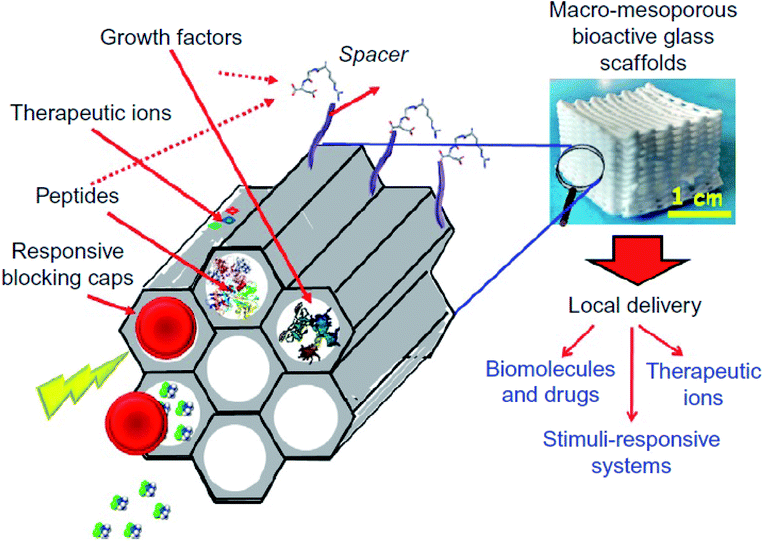 |
| Fig. 25 Schematic presentation of ordered MBGs for bone tissue regeneration. Reproduced with permission from ref. 382. Copyright 2018, Elsevier. | |
During the last ten years, MBGs have been widely explored for bone regeneration applications. Recently, Wu et al.454 reported delivery of dimethyloxallyl glycine (DMOG) using the MBG scaffold to meliorate angiogenesis and osteogenesis of human bone marrow stromal cells (BMSCs). It has been observed that MBG scaffold can imitate a hypoxic microenvironment and thus elevate the angiogenic capacity of human BMSCs. Yun et al.455 employed methylcellulose as a large pore porogen to prepare MBGs which demonstrated excellent apatite formation ability in SBF and assisted in the proliferation of MG-63 cells. In another study, Zhu et al.456 demonstrated that the MBG scaffolds with 80Si15Ca5P composition exhibit the best apatite mineralization ability and assisted in the proliferation and differentiation of BMSCs. In another investigation, MBG powders were integrated into the PCL matrix to form composite scaffolds which displayed apatite mineralization in SBF.457 The incorporation of MBG particles into PLAGA enhances the proliferation and ALP activities of human osteoblasts.458 In another investigation, the authors reported the synthesis of silk modified MBG scaffolds with substantially enhanced attachment, proliferation, differentiation and osteogenic gene expression of BMSCs.366 Moreover, various studies have shown that the integration of Fe, Sr, B, and Zr ions into MBG scaffolds can improve cell proliferation and osteogenic differentiation.459–462 Wu et al.463 in their excellent review highlights the potential of MBG particles, spheres, fibers and scaffolds as platforms for drug delivery and bone tissue regeneration. Besides, studies have also shown that both hydrophilic and hydrophobic drugs can also be effectively supplied using MBGs which are competent enough for substantially improving the proliferation, differentiation and osteogenic gene expression of human osteoblasts. These results deliver the paradigm change that osteoconductive drugs can be pre-installed in the MBG scaffold to incite both in vitro and in vivo osteogenesis for bone tissue engineering.463 Thus, achieving efficient drug delivery using the MBG scaffold is a fascinating prospect for bone tissue regeneration.
Recently, functionalized MBG scaffolds were synthesized for refined bone tissue regeneration.336 To carry out functionalization, amino and carboxylic groups were effectively grafted on to the synthesized mesoporous glasses via a post grafting process. The osteogenic capabilities of both MBG and functionalized MBG scaffolds were comprehensively investigated in vivo as well as in vitro using rabbit BMSCs. It has been discovered that both types of scaffolds could dramatically improve the proliferation and osteogenic differentiation of BMSCs. Amino functional group grafted scaffolds showed the greatest in vitro osteogenic capability as compared with the other two scaffolds by virtue of its positively charged surface. Furthermore, in vivo test results demonstrated that scaffolds grafted with the amino-functional groups could promote a higher level of bone regeneration in comparison with the other two scaffolds. It was reported that the surface characteristics and the decreased degradation rate of the amino group functionalized scaffold played a vital role in promoting bone regeneration.
The interconnected porous structure is essential for creating an effective scaffold for the migration of cells into the pores and tissue growth throughout the scaffold template. 3D scaffolds with interconnected porous structures can be manufactured using the sol–gel foaming process. The first sol–gel foam process was demonstrated by Jones et al.405,464Fig. 26 schematically presents the steps involved in the sol–gel foaming process.465 The hydrolyzed sol can be foamed by vigorous stirring in air and by including a surfactant.118 The surfactant reduces the surface tension as it is composed of molecules having polar (hydrophilic) and non-polar (hydrophobic) end groups and provides temporary stability to the foam. However, for the successful preparation of foam, it is necessary to expedite the gelation process. The gelation process can be expedited by adding HF acid so that the gelation takes place within minutes.466 On gelation, a prompt viscosity increase helps in the foaming process where the air bubbles are stabilized as the condensation reactions form the silica network around them. The generation of Si–O–Si bonds leads to shrinkage of bubble walls which brings the neighboring bubbles into close contact with each other. During aging and drying, the liquid vents from the point of bubble contacts and additional shrinkage occurs resulting in the rupture of the liquid film at the points of bubble contacts and open up the interconnects between the macropores.466 A hierarchical porous structure can be produced with interconnected macropores as shown in Fig. 27.467 There are several factors namely glass composition, processing temperature, surfactant and gelling agent concentration, and the water usage in the surfactant that can affect the pore structure.468,469 However, the most efficacious way of controlling the modal interconnect diameter is by changing the surfactant concentration while keeping all the other variables constant.468
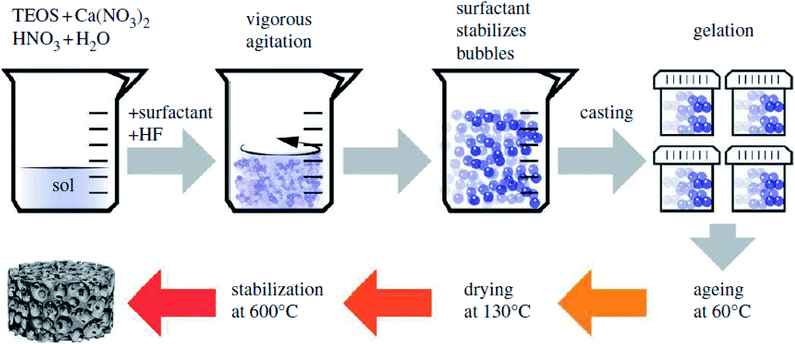 |
| Fig. 26 Schematic representation of sol–gel foaming process. Adapted from ref. 465. Copyright 2012, the Royal Society of Chemistry. | |
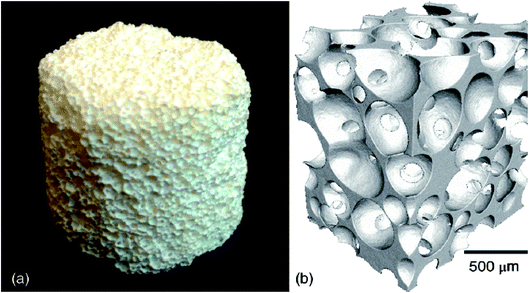 |
| Fig. 27 (a) Photograph of bioactive glass foam scaffold and (b) X-ray micro CT image. Reproduced with permission from ref. 467. Copyright 2015, John Wiley & Sons. | |
Using the sol–gel foaming method, bioactive glass scaffolds were produced having macropores of diameter up to 600 μm linked with the pore windows of diameter up to 200 μm and modal diameter greater than 100 μm.469,470In vitro test utilizing primary human osteoblasts revealed that the foam stimulates the formation and mineralization of bone nodules within fourteen days of culture.471 In another report, in vivo test demonstrated that foam scaffolds implanted in the rabbit crania promoted new bone growth at the same rate as that of the melt derived bioactive glass powder which is available commercially.472 In another study, Valerio et al.473 described the fabrication of sol–gel foam scaffolds with interrelated pores up to 500 μm, high porosity (88%) and specific surface area of 92 m2 g−1. The photograph and SEM micrograph of the synthesized glass foam is depicted in Fig. 28 where highly interconnected pores with adequate pore size can be observed.473 The macroporous glasses were examined in osteoblast cultures to assess adhesion, proliferation, collagen and ALP production. The osteoblast proliferation, as well as the collagen secretion, was reported to be higher in the presence of the foam as compared with the controlled sol–gel glass.473 Besides, the viable osteoblasts have been observed throughout the foam implying that the synthesized porous glass foams are a prosperous material for bone repair considering that they provide a favorable environment for the adhesion and proliferation of osteoblast.473 Moreover, the sol–gel foaming process has also been utilized to fabricate organic–inorganic hybrid scaffolds that can mimic the trabecular structure of bone.411
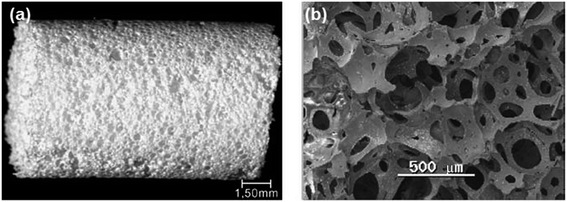 |
| Fig. 28 (a) Representation of synthesized sol–gel glass foam. (b) SEM microstructure. Adapted from ref. 473. Copyright 2005, Springer Nature. | |
In a similar study, Jones et al.474 utilized sol–gel derived bioactive silicate glasses of 70S30C (70 mol% SiO2, 30 mol% CaO) composition to prepare 3D porous foam scaffold having a hierarchical interconnected porous structure similar to the trabecular bone as depicted in Fig. 29. The bulk and true density of the fabricated scaffold was reported to be 0.25 g cm−3 and 2.71 g cm−3 respectively while the percent porosity of each scaffold was reported to be 91%.474 The fabricated scaffold supported the osteoblast growth and stimulated changes within the culture period of three weeks, as evidenced by improved ALP enzymatic activity of human osteoblasts which were cultured on the glass foam. The formation of mineralized bone nodules was stimulated without adding any supplementary factors such as ascorbic acid (AA), β-glycerophosphate and dexamethasone in the medium. The deposition of ECM was also validated by the improved production of the ECM collagen type I protein. Finally, the authors concluded that the scaffolds satisfy most of the criteria of becoming an ideal scaffold material for bone tissue engineering without the requirement of phosphate in the glass composition for in vitro mineralization of ECM on porous foam scaffold.474
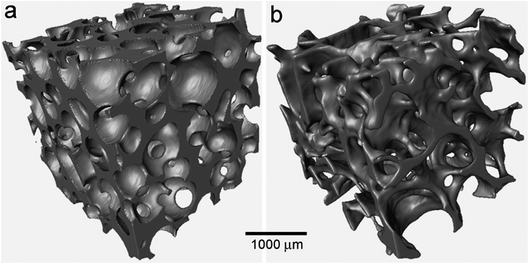 |
| Fig. 29 μCT images of (a) 3D porous scaffold prepared from sol–gel derived bioactive silicate glass and (b) human trabecular bone. Reproduced with permission from ref. 474. Copyright 2007, Elsevier. | |
6. Concluding remarks and future perspectives
The sol–gel methods have become very popular during the last two decades because of the chemical homogeneity (molecular level mixing), low processing temperatures, possibility to control size, shape and morphology of the synthesized materials. The inherent advantages of the sol–gel technique and the chemical modification of the precursors allow tailoring the material properties to a great extent. A varied set of potential materials can be synthesized by a sol–gel method providing extraordinary matrices for a wide range of organic as well as inorganic compounds. The advantages of the sol–gel technique in biomedical fields are ascribed to the possibility of synthesizing mechanically robust and chemically inert porous materials with the high specific surface area. The sol–gel technique offers a unique opportunity to form porous glass-based materials with tunable porosity as long as the synthesis parameters that influence the hydrolysis and condensation reaction involving inorganic precursors as well as the thermal treatment are precisely controlled. The low-temperature sol–gel synthesis imparts an opportunity for the integration of materials which would otherwise get spoiled due to high temperatures. For example; the sol–gel method enables the integration of biomolecules and therapeutic agents such as drugs, growth factors and proteins during the synthesis process all of which can be released in a controlled way. The evolution of sol–gel based materials for the entanglement of biomolecules has led to an enhancement in the number of materials available for different applications and the types of biomolecules which have the ability to being entrapped in an active state. There are varied options available for selecting different materials and varying synthesis parameters during sol–gel processing for both industrial and biomedical applications. However, the economic viability of scaling up of the sol–gel derived material is one of the hurdles that need to be overcome. Furthermore, the sol–gel method is a continuous process and it is quite laborious. Hence, batch to batch discrepancies might appear.
The current research in the tissue engineering field is concentrated on the development of high-performance multifunctional biomaterials i.e. materials that are capable of stimulating bone regeneration and exhibiting drug delivery capabilities with significant mechanical stability. In the framework of this research, sol–gel derived bioactive glasses have been customized as the implantable materials for bone tissue regeneration owing to their unique features namely the ability to degrade at a controllable rate, conversion to HA-like material, ability to bind firmly with the living tissues, and improving the growth and proliferation of osteoblasts. These unique properties of bioactive glasses result from their special chemical compositions. Further benefits of bioactive glasses in bone tissue regeneration include the possibility to incorporate various doping materials which show improved bone regeneration capability. Besides, the improved antibacterial or angiogenesis activity of bioactive glasses set up a bacteria-free atmosphere in implanted sites during the healing and regeneration of the soft and hard tissue. Several types of bioactive glasses with different compositions have been examined for bone tissue regeneration. Furthermore, the incorporation of therapeutic elements namely Ag, Cu, Sr, Co, Zn or Ga into the bioactive glasses has demonstrated more promising therapeutic effects than the original or un-doped bioactive glasses. Recent studies have shown the proangiogenesis potential of bioactive glasses which are proved beneficial for soft tissue repair.
The important aspect of bioactive glasses is their surface functionalization which provides increased opportunity for designing, developing and controlling the surface properties. This, in turn, influences their biological properties (biocompatibility, reactivity, bone-bonding and cell-stimulating ability) making the material more versatile. Also, it has been demonstrated that the microstructural characteristics, nucleation and HCA growth rate can be influenced by introducing functional groups on the surfaces of bioactive glasses. Moreover, surface functionalization of bioactive glasses is reported to improve their efficiency towards the delivery of hydrophobic drugs. Several strategies and challenging approaches towards surface modifications such as biological functionalization have been explored for performance optimization of bioactive glasses. Thus, surface functionalization is the key for exploiting the surface reactivity of bioactive glasses to meet various biological requirements associated with their wide range of biomedical and clinical applications.
MBGs represent the most promising family of bioactive glasses and have been emerging as fascinating biomaterial owing to their well-ordered mesoporous structure, high specific surface area and pore volumes (two-fold greater than the conventional sol–gel glass) and superior bioactivity. The improved bioactivity is ascribed to the highly ordered arrangements of mesopores with uniform size. The improved bioactivity and controlled drug delivery capability of MBGs render them an excellent starting material for manufacturing 3D scaffolds for bone tissue regeneration. However, considerable emphasis needs to be devoted to the probability of covalent grafting of osteoconductive agents (proteins, peptides, growth factors etc.) to the 3D scaffold surface which could serve as enticing signals for bone cells and assist in the bone regeneration process. In recent years, substantial progress is being made in developing MBGs with tailored architecture for desired applications other than bone tissue regeneration. Despite these advances in MBGs research, there remains scope for improving their bioactivity and controlled therapeutic delivery. Moreover, new research avenues are needed for viable vaccines, hormones, and therapeutic protein delivery in addition to the affirmation of growth factor and gene delivery. Also, in the future, MBGs should be examined in the form of a coating on the implants of other materials.
MBGs foams have shown the potential to be served as a scaffold material for bone tissue regeneration applications. These scaffolds should be degradable, bioactive and must possess compressive strength similar to porous bone. Also, they possess a hierarchical pore structure of interrelated macropores with intrinsic nanoporosity. However, fabricating bioactive glass foam scaffolds with the regulated structure for bone tissue engineering and drug delivery is still a challenge. Furthermore, the efficacious delivery of growth factors has always been a matter of concern. Therefore, polymer scaffolds were utilized for loading of growth factor with controlled release kinetics but they lack adequate osteoconductivity. Hence, polymer scaffolds are most commonly fabricated in the composites form using bioactive inorganic materials. Nevertheless, it is a challenge to match the degradation rates of the polymer matrix and the filler phase of the composite. The degradation rate of individual phases is different and does not match with the rate of new tissue formation which can result in the in vivo loss of mechanical properties. In an ideal case, both the phases ought to degrade at the rate which is suitable for the targeted application. The association of proficient drug delivery using MBGs scaffold is an enticing prospect for regenerative bone medicine.
Porous glass scaffolds show brittleness and exhibit inadequate fracture toughness, thereby restricting their utilization in load-carrying applications. To ameliorate the mechanical characteristics of scaffolds, numerous glass compositions which precipitate crystalline phases have been prepared and tested. The mechanical properties of bioactive glasses need to be explored extensively in vivo situations to strengthen their position as a promising biomaterial for bone tissue regeneration. Moreover, in future research on bioactive glasses, the degradation and bone ingrowth will play an important part as the mechanical properties of bioactive glasses can be significantly altered due to degradation with respect to time and with the bone-bonding, the behavior of the material can be modified drastically. Moreover, in the future, an attempt should be made in processing and designing innovative scaffolds with good mechanical properties especially for the load-bearing bones using bioactive glasses.
Conflicts of interest
The authors declare no conflict of interest.
Acknowledgements
This work has been supported by the Bavarian State Ministry of Economy and Media, Energy and Technology (Czech-Bavarian Cross-Border Cooperation Program, INTERREG V, EUS 2014–2020 Objective, MATEGRA-advanced porous biomaterials functionalized with stem cells for enhanced implant osseointegration, No. 201, co-funded by the ERDF and Ministry of Regional Development of the Czech Republic). Also, the authors would like to express their appreciation to the European Union for financial support provided through Mobility 3.0 project with Project No. CZ.02.2.69/0.0/0.0/16_027/0008370.
References
- G. J. Owens, R. K. Singh, F. Foroutan, M. Alqaysi, C. M. Han, C. Mahapatra and H. W. Kim, Sol–gel based materials for biomedical applications, Prog. Mater. Sci., 2016, 77, 1–79 CrossRef CAS
.
- S. N. Tan, W. Wang and L. Ge, 3.30 Biosensors based on sol–gel derived materials, Compr. Biomater. II, 2017, 3, 657–689 Search PubMed
.
- M. R. N. Monton, E. M. Forsberg and J. D. Brennan, Tailoring sol–gel derived silica materials for optical biosensing, Chem. Mater., 2012, 24, 796–811 CrossRef CAS
.
- U. G. Akpan and B. H. Hameed, The advancement in sol–gel method of doped TiO2 photocatalyst, Appl. Catal., A, 2010, 375, 1–11 CrossRef CAS
.
-
D. Levy and M. Zayat, The sol–gel handbook, ed. D. Levy and M. Zayat, vol. 1–3, Wiley VCH, USA, 2015, pp. 1–1508 Search PubMed
.
- J. M. Rosenholm, C. Sahlgren and M. Linden, Towards multifunctional, targeted drug delivery systems using mesoporous silica nanoparticles-opportunities & challenges, Nanoscale, 2010, 2, 1870–1883 RSC
.
- P. Yang, S. Gai and J. Lin, Functionalized mesoporous silica materials for controlled drug delivery, Chem. Soc. Rev., 2012, 41, 3679–3698 RSC
.
- K. Sumida, K. Liang, J. Reboul, I. A. Ibara, S. Furukawa and P. Falcaro, Sol–gel processing of metal organic frameworks, Chem. Mater., 2017, 29, 2626–2645 CrossRef CAS
.
-
L. M. Muresan, Corrosion protective coatings for Ti and Ti alloys used for biomedical implants, in Intelligent coatings for corrosion control, ed. A. Tiwari, J. Rawlins and L. H. Hihara, 2015, pp. 585–602 Search PubMed
.
-
G. Kickelbick, Introduction to sol–gel nanocomposites, in Sol–gel nanocomposites, Advances in sol–gel derived materials and technologies, ed. M. Guglielmi, G. Kickelbick and A. Martucci, Springer-Verlag, New York, 2014, pp. 1–19 Search PubMed
.
- D. Carta, D. M. Pickup, J. C. Knowles, M. E. Smith and R. J. Newport, Sol–gel synthesis of the P2O5–CaO–Na2O–SiO2 system as a novel bioresorbable glass, J. Mater. Chem., 2005, 15, 2134–2140 RSC
.
- S. Esposito, Traditional sol–gel chemistry as a powerful tool for the preparation of supported metal and metal oxide catalysts, Materials, 2019, 12, 668 CrossRef CAS PubMed
.
- T. Lopez, J. Manjarrez, D. Rembao, E. Vinogradova, A. Moreno and R. D. Gonzalez, An implantable sol–gel derived titania–silica carrier system for the controlled release of anticonvulsants, Mater. Lett., 2006, 60, 2903–2908 CrossRef CAS
.
- X. Chen, W. Zhang, Y. Lin, Y. Cai, M. Qiu and Y. Fan, Preparation of high-flux γ-alumina nanofiltration membranes by using a modified sol–gel method, Microporous Mesoporous Mater., 2015, 214, 195–203 CrossRef CAS
.
- S. Priya, J. R. Jones, V. Sophie, B. Robert, S. J. Victoria, L. L. Hench and P. M. Julia, Binary CaO–SiO2 gel-glasses for biomedical applications, Biomed. Mater. Eng., 2004, 14, 467–486 Search PubMed
.
- M. Abbasian, B. Massoumi, R. Mohammad-Rezaei, H. Samadian and M. Jaymand, Scaffolding polymeric biomaterials: are naturally occurring biological macromolecules more appropriate for tissue engineering, Int. J. Biol. Macromol., 2019, 134, 673–694 CrossRef CAS PubMed
.
- B. Massoumi, M. Hatamzadeh, N. Firouzi and M. Jaymand, Electrically conductive nanofibrous scaffold composed of poly(ethylene glycol)-modified polypyrrole and poly(ε-caprolactone) for tissue engineering applications, Mater. Sci. Eng., C, 2019, 98, 300–310 CrossRef CAS PubMed
.
- S. Vandhaanooni and M. Eskandani, Electrically conductive biomaterials based on natural polysaccharides: challenges and applications in tissue engineering, Int. J. Biol. Macromol., 2019, 141, 636–662 CrossRef
.
- H. Samadian, H. Maleki, Z. Allahyari and M. Jaymand, Natural polymers-based light-induced hydrogels: promising biomaterials for biomedical applications, Coord. Chem. Rev., 2020, 420, 213432 CrossRef CAS
.
- M. P. Lutolf and J. A. Hubbel, Synthetic biomaterials as instructive extracellular microenvironments for morphogenesis in tissue engineering, Nat. Biotechnol., 2005, 23, 47–55 CrossRef CAS PubMed
.
- L. S. Nair and C. T. Laurencin, Biodegradable polymers as biomaterials, Prog. Polym. Sci., 2007, 32, 762–798 CrossRef CAS
.
- B. Toirac, A. Garcia-Casas, S. C. Cifuentes, J. J. Aguilera-Correa, J. Esteban, A. Mediero and A. Jimmez-Morales, Electrochemical characterization of coatings for local prevention of Candida infections on titanium based biomaterials, Prog. Org. Coat., 2020, 146, 105681 CrossRef CAS
.
- J. J. Aguilera-Correa, A. Garcia-Casas, A. Mediero, D. Romera, F. Mulero, I. Cuevas-Lopez, A. Jimenez-Morales and J. Esteban, A new antibiotic loaded sol–gel can prevent bacterial prosthetic joint infections: from in vitro studies to an in vivo model, Front. Microbiol., 2020, 10, 2935 CrossRef
.
- L. Ming-Cheng, C. Chun-Cheng, W. I-Ting and D. Shinn-Jyh, Enhanced antibacterial activity of calcium silicate-based hybrid cements for bone repair, Mater. Sci. Eng., C, 2020, 110, 110727 CrossRef
.
- M. Roozbahani, M. Alehosseini, M. Kharaziha and R. Emadi, Nano-calcium phosphate bone cement based on Si-stabilized α-tricalcium phosphate with improved mechanical properties, Mater. Sci. Eng., C, 2017, 81, 532–541 CrossRef CAS
.
- H. Li, J. Li, J. Jiang, F. Lv, J. Chang, S. Chen and C. Wu, An osteogenesis/angiogenesis-stimulation artificial ligament for anterior cruciate ligament reconstruction, Acta Biomater., 2017, 54, 399–410 CrossRef CAS
.
- G. Wu, X. Deng, J. Song and F. Chen, Enhanced biological properties of biomimetic apatite fabricated polycaprolactone/chitosan nanofibrous bio-composite for tendon and ligament regeneration, J. Photochem. Photobiol., B, 2018, 178, 27–32 CrossRef CAS PubMed
.
- M. Catauro, F. bollino, F. Papale, C. Ferrara and P. Mustarelli, Silica–polyethylene glycol hybrids synthesized by sol–gel: biocompatibility improvement of titanium implants by coating, Mater. Sci. Eng., C, 2015, 55, 118–125 CrossRef CAS PubMed
.
- A. Cerqueira, F. Romero-Gavilan, N. Araujo-Gomes, I. Garcia-Arnez, C. Martinez-Ramos, S. Ozturan, M. Azkargota, F. Elortza, M. Gurruchanga, J. Suay and I. Goni, A possible use of melatonin in the dental field: protein adsorption and in vitro cell response on coated titanium, Mater. Sci. Eng., C, 2020, 116, 111262 CrossRef CAS PubMed
.
- W. D. Lee, R. Gawri, R. M. Pilliar, W. L. Stanford and R. A. Kandel, Sol–gel-derived hydroxyapatite films over porous calcium polyphosphate substrates for improved tissue engineering of osteochondral-like constructs, Acta Biomater., 2017, 62, 352–361 CrossRef CAS PubMed
.
- M. Hu, J. Fang, Y. Zhang, X. Wang, W. Zhong and Z. Zhou, Design and evaluation a kind of functional biomaterial for bone tissue engineering: selenium/mesoporous bioactive glass nanospheres, J. Colloid Interface Sci., 2020, 579, 654–666 CrossRef CAS
.
- S. Mondal, G. Hoang, P. Manivasagan, M. S. Moorthy, T. P. Nguyen, T. T. V. Phan, H. H. Kim, M. H. Kim, S. Y. Nam and J. Oh, Nano-hydroxyapatite bioactive glass composite scaffold with enhanced mechanical and biological performance for tissue engineering application, Ceram. Int., 2018, 44, 15735–15746 CrossRef CAS
.
- X. Wang, Y. Zhang, C. Lin and W. Zhong, Sol–gel derived terbium-containing mesoporous bioactive glasses nanospheres: in vitro hydroxyapatite formation and drug delivery, Colloids Surf., B, 2017, 160, 406–415 CrossRef CAS PubMed
.
- S. Sebastiammal, A. S. L. Fathima, S. Devanesan, M. S. AlSalhi, J. Henry, M. Govindarajan and B. Vaseeharan, Curcumin-encased hydroxyapatite nanoparticles as novel biomaterials for antimicrobial, antioxidant and anticancer applications: a perspective of nano-based drug delivery, J. Drug Delivery Sci. Technol., 2020, 57, 101752 CrossRef CAS
.
- C. Fa-Ming and L. Xiaohua, Advancing biomaterials of human origin for tissue engineering, Prog. Polym. Sci., 2016, 53, 86–168 CrossRef
.
- H. Yuan, J. D. De Bruijn, Y. Li, J. Feng, Z. Yang, K. De Groot and X. Zhang, Bone formation induced by calcium phosphate ceramics in soft tissue of dogs: a comparative study between porous α-TCP and β-TCP, J. Mater. Sci.: Mater. Med., 2001, 12, 7–13 CrossRef CAS PubMed
.
- S. Areva, H. Paldan, T. Peltola, T. Narhi, M. Jokinen and M. Linden, Use of sol–gel-derived titania coating for direct soft tissue attachment, J. Biomed. Mater. Res., Part A, 2004, 70, 169–178 CrossRef PubMed
.
- L. Meseguer-Olmo, M. J. Ros-Nicolas, V. Vincente-Ortega, M. Alcaraz-Banos, M. Clavel-Sainz, D. Arcos, C. V. Ragel, M. Vallet-Regi and C. I. Meseguer-Ortiz, A bioactive sol–gel glass implant for in vivo gentamicin release. Experimental model in rabbit, J. Orthop. Res., 2006, 24, 454–460 CrossRef CAS PubMed
.
- R. Gupta and N. K. Chaudhary, Entrapment of biomolecules in sol–gel matrix for applications in biosensors: problems and future prospects, Biosens. Bioelectron., 2007, 22, 2387–2399 CrossRef CAS
.
- M. S. Ahola, E. S. Sailynoja, M. H. Raitavno, M. H. Vaahtio, J. I. Salonen and A. U. O. Yli-Urpo, In vitro release of heparin from silica xerogels, Biomaterials, 2001, 22, 2163–2170 CrossRef CAS
.
- V. B. Kandimalla, V. S. Tripathi and H. Ju, Immobilization of biomolecules in sol–gels: biological and analytical applications, Crit. Rev. Anal. Chem., 2006, 36, 73–106 CrossRef CAS
.
- S. Kargozar, N. Lotfibakshaiesh, J. Ai, A. Samadikuchaksaraie, R. G. Hill, P. A. Shah, P. B. Milan, M. Mozafari, M. Fathi and M. T. Joghataei, Synthesis, physico-chemical and biological characterization of strontium and cobalt substituted bioactive glasses for bone tissue engineering, J. Non-Cryst. Solids, 2016, 449, 133–140 CrossRef CAS
.
- S. Kargozar, F. Baino, S. Hamzehlou, R. G. Hill and M. Mozafari, Bioactive glasses: sprouting angiogenesis in tissue engineering, Trends Biotechnol., 2018, 36, 430–444 CrossRef CAS
.
- S. Kargozar, M. Mozafari, S. J. Hashemian, P. B. Milan, S. Hamzehlou, M. Soleimani, M. T. Joghataei, M. Gholipourmalekabadi, A. Korourian, K. Mousavizadeh and A. M. Seifalian, Osteogenic potential of stem cells-seeded bioactive nanocomposite scaffolds: a comparative study between human mesenchymal stem cells derived from bone, umbilical cord Wharton's jelly, and adipose tissue, J. Biomed. Mater. Res., Part B, 2018, 106, 61–72 CrossRef CAS PubMed
.
- L. L. Hench, N. Roki and M. B. Fenn, Bioactive glass: importance of structure and properties in bone regeneration, J. Mol. Struct., 2014, 1073, 24–30 CrossRef CAS
.
- A. Hoppe, N. S. Guldal and A. R. Boccaccini, A review of the biological response to ionic dissolution products from bioactive glass and glass-ceramics, Biomaterials, 2011, 32, 2757–2774 CrossRef CAS
.
- M. Vallet-Regi, I. Izquierdo-Barba and M. Colilla, Structure and functionalization of mesoporous bioceramics for bone tissue regeneration and local drug delivery, Philos. Trans. R. Soc., A, 2012, 370, 1400–1421 CrossRef CAS
.
- X. Yan, C. Yu, X. Zhou, J. Tang and D. Zhao, Highly ordered mesoporous bioactive glasses with superior in vitro bone-forming bioactivities, Angew. Chem., Int. Ed., 2004, 43, 5980–5984 CrossRef CAS
.
- P. Chocolata, V. Kulda and V. Babuska, Fabrication of scaffolds for bone tissue regeneration, Materials, 2019, 12, 568 CrossRef PubMed
.
- S. Ni, J. Chang and L. Chou, A novel bioactive porous CaSiO3 scaffold for bone tissue engineering, J. Biomed. Mater. Res., Part A, 2006, 76, 196–205 CrossRef PubMed
.
- A. J. El Haj, M. A. T. Wood, P. Homas and Y. Yang, Controlling cell biomechanics in orthopaedic tissue engineering and repair, Pathol. Biol., 2005, 53, 581–589 CrossRef
.
- M. Vallet-Regi, L. Ruiz-Gonzalez, I. Izquierdo-Barba and J. M. Gonzalez-Calbet, Revisiting silica based ordered mesoporous materials medical applications, J. Mater. Chem., 2006, 16, 26–31 RSC
.
- A. Hertz, V. FitzGerald, E. Pignotti, J. C. Knowles, T. Sen and I. J. Bruce, Preparation and characterization of porous silica and silica/titania monoliths for potential use in bone replacement, Microporous Mesoporous Mater., 2012, 156, 51–61 CrossRef CAS
.
- I. Izquierdo-Barda, L. Ruiz-Gonzalez, J. M. Gonzalez-Calbet and M. Vallet-Regi, Tissue regeneration: a new property of mesoporous material, Solid State Sci., 2005, 7, 983–989 CrossRef
.
- T. Sen, G. J. T. Tiddy, J. L. Casci and M. W. Anderson, Meso-cellular silica foams, macro-cellular silica foams and mesoporous solids: a study of emulsion-mediated synthesis, Microporous Mesoporous Mater., 2005, 78, 255–263 CrossRef CAS
.
- H. Sun and H. L. Yang, Calcium phosphate scaffolds combined with bone morphogenetic proteins or mesenchymal stem cells in bone tissue engineering, Chin. Med. J., 2015, 128, 1121–1127 CrossRef CAS PubMed
.
- Q. Fu, E. Saiz, M. N. Rahaman and A. P. Tomsia, Bioactive glass scaffolds for bone tissue engineering: state of the art and future perspectives, Mater. Sci. Eng., C, 2011, 31, 1245–1256 CrossRef CAS
.
- H. M. Kim, Ceramic bioactivity and related biomimetic strategy, Curr. Opin. Solid State Mater. Sci., 2003, 7, 289–299 CrossRef CAS
.
- J. P. Zhong and D. C. Greenspan, Processing and properties of sol–gel bioactive glasses, J. Biomed. Mater. Res., 2000, 53, 694–701 CrossRef CAS
.
- J. Ma, C. Z. Chen, D. G. Wang, X. G. Meng and J. Z. Shi, In vitro degradability and bioactivity of mesoporous CaO–MgO–P2O5–SiO2 glasses synthesized by sol–gel method, J. Sol-Gel Sci. Technol., 2010, 54, 69–76 CrossRef CAS
.
- L. L. Hench, J. M. Polak, I. D. Xynos and L. D. K. Buttery, Bioactive materials to control cell cycle, Mater. Res. Innovations, 2000, 3, 313–323 CrossRef CAS
.
- K. Rezwan, Q. Z. Chen, J. J. Blaker and A. R. Boccaccini, Biodegradable and bioactive porous polymer/inorganic composite scaffolds for bone tissue engineering, Biomaterials, 2006, 27, 3413–3431 CrossRef CAS PubMed
.
- W. D. S. Roberto, M. M. Pereira and T. P. Ribiero de Campos, Analysis of bioactive glasses obtained by sol–gel processing for radioactive implants, Mater. Res., 2003, 6, 123–127 CrossRef CAS
.
-
A. H. Choi, R. C. Conway, S. Cazalbou and B. Ben-Nissan, Maxillofacial bioceramics in tissue engineering: production techniques, properties and applications, in Fundamental Biomaterials: Ceramics, S. Thomas, P. Balakrishnan and M. S. Sreekala, Woodhead Publishing, Elsevier, 2018, pp. 63–93 Search PubMed
.
- M. A. Malik, M. Y. Wani and M. A. Hashim, Microemulsion method: a novel route to synthesize organic and inorganic nanomaterials: 1st nano update, Arabian J. Chem., 2012, 5, 397–417 CrossRef CAS
.
- K. Zheng and A. R. Boccaccini, Sol–gel processing of bioactive glass nanoparticles: a review, Adv. Colloid Interface Sci., 2017, 249, 363–373 CrossRef CAS PubMed
.
- B. L. Cushing, V. L. Kolesnichenko and C. J. O'Connor, Recent advances in the liquid-phase syntheses of inorganic nanoparticles, Chem. Rev., 2004, 104, 3893–3946 CrossRef CAS PubMed
.
- J. Eastoe, M. J. Hollamby and L. Hudson, Recent advances in nanoparticle synthesis with reversed micelles, Adv. Colloid Interface Sci., 2006, 128–130, 5–15 CrossRef CAS PubMed
.
- C. Karagiozov and D. Momchilova, Synthesis of nanosized particles from metal carbonates by the method of reverse micelles, Chem. Eng. Process., 2005, 44, 115–119 CrossRef CAS
.
- Y. Sun, G. Guo, D. Tao and Z. H. Wang, Reverse microemulsion directed synthesis of hydroxyapatite nanoparticles under hydrothermal conditions, J. Phys. Chem. Solids, 2007, 68, 373–377 CrossRef CAS
.
- A. Lukowiak, J. Lao, J. Lacroix and J. M. Nedelec, Bioactive glass nanoparticles obtained through sol–gel chemistry, Chem. Commun., 2013, 49, 6620–6622 RSC
.
- X. Li, X. Chen, G. Miao, H. Liu, C. Mao, G. Yuan, Q. Liang, X. Shen, C. Ning and X. Fu, Synthesis of radial mesoporous bioactive glass particles to deliver osteoactivin gene, J. Mater. Chem. B, 2014, 2, 7045–7054 RSC
.
- Q. Liang, Q. Hu, G. Miao, B. Yuan and X. Chen, A facile synthesis of novel mesoporous bioactive glass nanoparticles with various morphologies and tunable mesostructure by sacrificial liquid template method, Mater. Lett., 2015, 148, 45–49 CrossRef CAS
.
- T. H. Kim, R. K. Singh, M. S. Kang, J. H. Kim and H. W. Kim, Gene delivery nanocarriers of bioactive glass with unique potential to load BMP2 plasmid DNA and to internalize into mesenchymal stem cells for osteogenesis and bone regeneration, Nanoscale, 2016, 8, 8300–8311 RSC
.
- T. H. Kim, R. K. Singh, M. S. Kang, J. H. Kim and H. W. Kim, Inhibition of osteoclstogenesis through siRNA delivery with tunable mesoporous bioactive nanocarriers, Acta Biomater., 2016, 29, 352–364 CrossRef CAS PubMed
.
- Y. Wang and X. Chen, Facile synthesis of hollow mesoporous bioactive glasses with tunable shell thickness and good monodispersity by micro-emulsion, Mater. Lett., 2017, 189, 325–328 CrossRef CAS
.
- K. Sinko, Influence of chemical conditions on the nanoporous structure of silicate aerogels, Materials, 2010, 3, 704–740 CrossRef CAS
.
- C. Vichery and J. M. Nedelc, Bioactive glass nanoparticles: from synthesis to material design for biomedical applications, Materials, 2016, 9, 288–295 CrossRef PubMed
.
- Y. Wang, H. Pan and X. Chen, The preparation of hollow mesoporous bioglass nanoparticles with excellent drug delivery capacity for bone tissue regeneration, Front. Chem., 2019, 7, 283 CrossRef CAS PubMed
.
- Q. Hu, Y. Li, N. Zhao, C. Ning and X. Chen, Facile synthesis of hollow mesoporous bioactive glass submicron spheres with a tunable cavity size, Mater. Lett., 2014, 134, 130–133 CrossRef CAS
.
- H. Duan, J. Diao, N. Zhao and Y. Ma, Synthesis of hollow mesoporous bioactive glass microspheres with tunable shell thickness by hydrothermal assisted self transformation method, Mater. Lett., 2016, 167, 201–204 CrossRef CAS
.
- J. Xiao, Y. Wan, F. Yao, Y. Huang, Y. Zhu, Z. Yang and H. Luo, Constructing 3D scaffold with 40 nm diameter hollow mesoporous bioactive glass nanofibres, Mater. Lett., 2019, 248, 201–203 CrossRef CAS
.
- M. A. Fardad, Catalysts and the structure of SiO2 sol–gel films, J. Mater. Sci., 2000, 35, 1835–1841 CrossRef CAS
.
- A. A. Issa and A. S. Luyt, Kinetics of alkoxysilanes and organoalkoxysilanes polymerization: a review, Polymers, 2019, 11, 537 CrossRef PubMed
.
- Q. Z. Chen, Y. Li, L. Y. Jin, J. M. W. Quinn and P. A. Komesaroff, A new sol–gel process for producing Na2O-containing bioactive glass ceramics, Acta Biomater., 2010, 6, 4143–4153 CrossRef CAS PubMed
.
- W. Xia and J. Chang, Preparation and characterization of nano-bioactive-glasses (NBG) by a quick alkali-mediated sol–gel method, Mater. Lett., 2007, 61, 3251–3253 CrossRef CAS
.
- X. Chen, C. GuO and N. Zhao, Preparation and characterization of the sol–gel nano-bioactive glasses modified by the coupling agent gamma-aminopropyltriethoxysilane, Appl. Surf. Sci., 2008, 255, 466–468 CrossRef CAS
.
- S. I. Roohani-Esfahani, S. Nouri-Khorasani, Z. F. F. Lu, R. C. Appleyard and H. Zreiqat, Effects of bioactive glass nanoparticles on the mechanical and biological behavior of composite coated scaffolds, Acta Biomater., 2011, 7, 1307–1318 CrossRef CAS PubMed
.
- Z. Hong, G. M. Luz, P. J. Hampel, M. Jin, A. Liu, X. Chen and J. F. Mano, Mono-dispersed bioactive glass nanospheres: preparation and effects on biomechanics of mammalian cells, J. Biomed. Mater. Res., Part A, 2010, 95, 747–754 CrossRef PubMed
.
- Z. Hong, R. L. Reis and J. F. Mano, Preparation and in vitro characterization of scaffolds of poly(L-lactic acid) containing bioactive glass ceramic nanoparticles, Acta Biomater., 2008, 4, 1297–1306 CrossRef CAS PubMed
.
- G. M. Luz and J. F. Mano, Nanoengineering of bioactive glasses: hollow and dense nanospheres, J. Nanopart. Res., 2013, 15, 1457–1468 CrossRef
.
- S. Nagayama, K. Kajihara and K. Kanamura, Synthesis of nanocrystalline LaF3 doped silica glasses by hydrofluoric acid catalyzed sol–gel process, Mater. Sci. Eng., B, 2012, 177, 510–514 CrossRef CAS
.
- R. Li, A. E. Clark and L. L. Hench, An investigation of bioactive glass powders by sol–gel processing, J. Appl. Biomater., 1991, 2, 231–239 CrossRef CAS PubMed
.
-
J. R. Jones, Bioactive glass, in Bioceramics and their clinical applications, ed. T. Kokubo, Woodhead Publishing Ltd., Cambridge UK, 2008, pp. 266–286 Search PubMed
.
- J. R. Jones, New trends in bioactive scaffolds: the importance of nanostructure, J. Eur. Ceram. Soc., 2009, 29, 1275–1281 CrossRef CAS
.
- J. R. Jones, Review of bioactive glass: from Hench to hybrids, Acta Biomater., 2013, 9, 4457–4486 CrossRef CAS PubMed
.
- G. Kaur, G. Pickrell, S. Sriranganathan, V. Kumar and D. Homa, Review and the state of the art: sol–gel and melt quenched bioactive glasses for tissue engineering, J. Biomed. Mater. Res., Part B, 2016, 104, 1248–1275 CrossRef CAS PubMed
.
- A. J. Salinas and M. Vallet-Regi, Bioactive ceramics: from bone grafts to tissue engineering, RSC Adv., 2013, 3, 11116–11131 RSC
.
- D. S. Brauer, Bioactive glasses – structure and properties, Angew. Chem., Int. Ed., 2015, 54, 4160–4181 CrossRef CAS PubMed
.
-
L. Hupa and K. H. Karlsson, Tailoring of bioactive glasses, in Bioactive glasses – fundamentals, technology and applications, ed. A. R. Boccaccinni, D. S. Brauer and L. Hupa, The Royal Society of Chemistry, 2017, pp. 136–160 Search PubMed
.
-
J. E. Shelby, Introduction to glass science and technology, The Royal Society of Chemistry, 2nd edn, 2005 Search PubMed
.
-
L. Hupa, Composition property relation of bioactive glasses; materials, properties and applications, ed. H. Ylanen, Woodhand Publishing, 2018 Search PubMed
.
- A. Pedone, G. Malavasi and M. C. Menziani, Computational insight into the effect of CaO/MgO substitution on the structural properties of phospho-silicate bioactive glasses, J. Phys. Chem. C, 2009, 113, 15723–15730 CrossRef CAS
.
- S. J. Watts, R. G. Hill, M. D. O'Donnell and R. V. Law, Influence of magnesia on the structure and properties of bioactive glasses, J. Non-Cryst. Solids, 2010, 356, 517–524 CrossRef CAS
.
- R. G. Hill and D. S. Brauer, Predicting the bioactivity of glasses using the network connectivity or split network models, J. Non-Cryst. Solids, 2011, 357, 3884–3887 CrossRef CAS
.
- A. Tilocca, Structural models of bioactive glasses from molecular dynamics simulations, Proc. R. Soc. A, 2009, 465, 1003–1027 CrossRef CAS
.
- M. W. G. Lockyer, D. Holland and R. Dupree, NMR investigation of the structure of some bioactive and related glasses, J. Non-Cryst. Solids, 1995, 188, 207–219 CrossRef CAS
.
- M. Eden, The split network analysis for exploring composition–structure correlations in multi-component glasses: I. Rationalizing bioactivity-composition trends of bioglasses, J. Non-Cryst. Solids, 2011, 357, 1595–1602 CrossRef CAS
.
- M. D. O'Donnell, Predicting bioactive glass properties from the molecular chemical composition: glass transition temperature, Acta Biomater., 2011, 7, 2264–2269 CrossRef PubMed
.
- M. Eden, NMR studies of oxide based glasses, Annu. Rep. Prog. Chem., Sect. C: Phys. Chem., 2012, 108, 177–221 RSC
.
- M. Vallet-Regi, Ceramics for medical applications, perspective article, J. Chem. Soc., Dalton Trans., 2001, 2, 97–108 RSC
.
- J. R. Jones, L. M. Ehrenfried, P. Sarapavan and L. L. Hench, Controlling ion release from bioactive glass foam scaffolds with antibacterial properties, J. Mater. Sci.: Mater. Med., 2006, 17, 989–996 CrossRef CAS PubMed
.
- Y. Zhao, C. Ning and J. Chang, Sol–gel synthesis of Na2CaSiO4 and its in vitro biological behaviors, J. Sol-Gel Sci. Technol., 2009, 52, 69–74 CrossRef CAS
.
- P. Saravapavan, J. R. Jones, R. S. Pryce and L. L. Hench, Bioactivity of gel–glass powders in the CaO–SiO2 system: a comparison with ternary (CaO–P2P5–SiO2) and quaternary glasses (SiO2–CaO–P2O5–Na2O), J. Biomed. Mater. Res., Part A, 2003, 66, 110–119 CrossRef PubMed
.
- A. Tilocca and A. N. Cormack, Structural effects of phosphorus inclusion in bioactive silicate glasses, J. Phys. Chem. B, 2007, 111, 14256–14264 CrossRef CAS PubMed
.
- D. Arcos, D. C. Greenspan and M. Vallet-Regi, Influence of the stabilization temperature on textural and structural features and ion release in SiO2–CaO–P2O5 sol–gel glasses, Chem. Mater., 2002, 14, 1515–1522 CrossRef CAS
.
- F. Balas, D. Arcos, J. Perez-Pariente and M. Vallet-Regi, Textural properties of SiO2–CaO–P2O5 glasses prepared by the sol–gel method, J. Mater. Res., 2001, 16, 1345–1348 CrossRef CAS
.
- D. Arcos and M. Vallet-Regi, Sol–gel silica-based biomaterials and bone tissue regeneration, Acta Biomater., 2010, 6, 2874–2888 CrossRef CAS PubMed
.
- M. N. Rahman, D. E. Day, D. S. Bal, Q. Fu, S. B. Jung, L. F. Bonewald and A. P. Tomsia, Bioactive glass in tissue engineering, Acta Biomater., 2011, 7, 2355–2373 CrossRef PubMed
.
- P. Kiran, V. Ramakrishna, M. Trebbin, N. K. Udayashankar and H. D. Shashikala, Effective role of CaO/P2O5 ratio on SiO2–CaO–P2O5 glass system, J. Adv. Res., 2017, 8, 279–288 CrossRef CAS PubMed
.
- P. Sepulveda, J. R. Jones and L. L. Hench, Characterization of melt-derived 45S5 and sol–gel-derived 58S bioactive glasses, J. Biomed. Mater. Res., 2001, 58, 734–740 CrossRef CAS PubMed
.
- A. Oki, B. Parveen, S. Hossain, S. Adeniji and H. Donahue, Preparation and in vitro bioactivity of zinc containing sol–gel-derived bioglass materials, J. Biomed. Mater. Res., Part A, 2004, 69, 216–221 CrossRef PubMed
.
- A. Moghanian, A. Sedghi, A. Ghorbanoghli and E. Salari, The effect of magnesium content on the in vitro bioactivity, biological behavior and antibacterial activity of sol–gel derived 58S bioactive glass, Ceram. Int., 2018, 44, 9422–9432 CrossRef CAS
.
- X. Liu, W. Hunag, H. Fu, A. Yao, D. Wang, H. Pan, W. W. Lu, X. Jiang and X. Zhang, Bioactive borosilicate glass scaffolds: in vitro degradation and bioactivity behaviors, J. Mater. Sci.: Mater. Med., 2009, 20, 1237–1243 CrossRef CAS PubMed
.
- J. Lao, J. M. Nedelec and E. Jallot, New strontium-based bioactive glasses: physicochemical reactivity and delivering capability of biologically active dissolution products, J. Mater. Chem., 2009, 19, 2940–2949 RSC
.
- V. G. Varansi, E. Siaz, P. M. Loomer, B. Ancheta, N. Uritani, A. P. Tomsia, S. J. Marshall and G. W. Marshall, Enhanced osteocalcin expression by osteoblast-like cells (MC3T3-E1) exposed to bioactive coating glass (SiO2–CaO–P2O5–MgO–K2O–Na2O system) ions, Acta Biomater., 2009, 5, 3536–3547 CrossRef PubMed
.
- L. L. Hench, Challenges for bioceramics in the 21st century, Am. Ceram. Soc. Bull., 2005, 84, 18–21 CAS
.
- H. Aguiar, J. Serra, P. Gonzalez and B. Leon, Influence of the stabilization temperature on the structure of bioactive sol–gel silicate glasses, J. Am. Chem. Soc., 2010, 93, 2286–2291 CAS
.
- G. Kaur, V. Kumar, F. Baino, J. C. Mauro, G. Pickrell, I. Evans and O. Breteano, Mechanical properties of bioactive glasses, ceramics and composites: state of the art review and future challenges, Mater. Sci. Eng., C, 2019, 104, 109895 CrossRef CAS PubMed
.
- R. F. Lenza, W. L. Vasconcelos, J. R. Jones and L. L. Hench, Surface-modified 3D scaffolds for tissue engineering, J. Mater. Sci.: Mater. Med., 2002, 13, 837–842 CrossRef CAS PubMed
.
- L. L. Hench and J. M. Polak, Third-generation biomedical materials, Science, 2002, 295, 1014–1017 CrossRef CAS PubMed
.
-
V. Kumar, G. Pickreu, S. G. Waldrop and N. Sriranganathan, Future perspectives of bioactive glasses for the clinical applications, in Bioactive glasses: potential biomaterials for future therapy, ed. G. Kaur, Springer Publication, 2017, p. 314 Search PubMed
.
- M. Catauro, F. Bollino, R. A. Renella and F. Papale, Sol–gel synthesis of SiO2–CaO–P2O5 glasses: influence of the heat treatment on their bioactivity and biocompatibility, Ceram. Int., 2015, 73, 12578–12588 CrossRef
.
- M. Dziadek, B. Zagrajczuk, E. Menassck, A. Wegrzynowicz, J. Pawlik and K. Cholewa-Kowalska, Gel derived SiO2–CaO–P2O5 bioactive glasses and glass ceramics modified by SrO addition, Ceram. Int., 2016, 42, 5842–5857 CrossRef CAS
.
- M. Vallet-Regi, D. Arcos and J. Perez-Pariente, Evolution of porosity during in vitro hydroxycarbonate apatite growth in sol–gel glasses, J. Biomed. Mater. Res., 2000, 51, 23–28 CrossRef CAS PubMed
.
- M. Vallet-Regi, C. V. Ragel and A. J. Salinas, Glasses with medical applications, Eur. J. Inorg. Chem., 2003, 1029–1042 CrossRef CAS
.
- M. Hamadouche, A. Meunier, D. C. Greenspan, C. Blanchat, J. P. Zhong, G. P. La Torre and L. Sedel, Long-term in vivo bioactivity and degradability of bulk sol–gel bioactive glasses, J. Biomed. Mater. Res., 2001, 54, 560–566 CrossRef CAS PubMed
.
- J. Gil-Albarova, R. Garrido-Lahiguera, A. J. Salinas, J. Roman, A. L. Bueno-Lozano, R. Gil-Albarova and M. Vallet-Regi, The in vivo performance of a sol–gel glass and glass ceramic in the treatment of limited bone defects, Biomaterials, 2004, 25, 4639–4645 CrossRef CAS PubMed
.
- I. A. Silver and M. Erecinska, Interactions of osteoblastic and other cells with bioactive glasses and silica in vitro and in vivo, Materialwiss. Werkstofftech., 2003, 34, 1069–1075 CrossRef CAS
.
- C. Lin, C. Mao, J. Zhang, Y. Li and X. Chen, Healing effect of bioactive glass ointment on full thickness skin wounds, Biomed. Mater., 2012, 7, 045017 CrossRef PubMed
.
- W. H. Xie, X. F. Chen, G. H. Miao, J. Y. Tang and X. L. Fu, Regulation of cellular behaviors of fibroblasts related to wound healing by sol–gel derived bioactive glass particles, J. Biomed. Mater. Res., Part A, 2016, 104, 2420–2429 CrossRef CAS PubMed
.
- A. J. Salinas, A. I. Martin and M. Vallet-Regi, Bioactivity of three CaO–P2O5–SiO2 sol–gel glasses, J. Biomed. Mater. Res., 2002, 61, 524–532 CrossRef CAS PubMed
.
- J. Ma, C. Z. Chen, D. G. Wang, Y. Jiao and J. Z. Shi, Effect of magnesia on the degradability and bioactivity of sol–gel derived SiO2–CaO–MgO–P2O5 system glasses, Colloids Surf., B, 2010, 81, 87–95 CrossRef CAS PubMed
.
- A. J. Salinas, J. Roman, M. Vallet-Regi, J. M. Oliveria, R. M. Correia and M. H. Fernandes, In vitro bioactivity of glass and glass-ceramics of the 3CaO·P2O5–CaO·SiO2–CaO·MgO·2SiO2 system, Biomaterials, 2000, 21, 251–257 CrossRef CAS PubMed
.
- J. Perez-Pariente, F. Balas and M. Vallet-Regi, Surface and chemical study of SiO2·P2O5·CaO·(MgO) bioactive glasses, Chem. Mater., 2000, 12, 750–755 CrossRef CAS
.
- A. Saboori, M. Rabiee, F. Mutarzadeh, M. Sheikhi, M. Tahiri and M. Karimi, Synthesis, characterizations and in vitro bioactivity of sol–gel derived SiO2–CaO–P2O5–MgO bioglasses, Mater. Sci. Eng., C, 2009, 29, 335–340 CrossRef CAS
.
- M. Bellantone, N. J. Coleman and L. L. Hench, Bacteriostatic action of a novel four-component bioactive glass, J. Biomed. Mater. Res., 2000, 51, 484–490 CrossRef CAS PubMed
.
- G. Hu, L. Xiao, P. Tong, D. Bi, H. Wang, H. Ma, G. Zhu and H. Liu, Antibacterial hemostatic dressings with nanoporous bioglass containing silver, Int. J. Nanomed., 2012, 7, 2613–2620 CrossRef CAS PubMed
.
- J. Pratten, S. N. Nazhat, J. J. Blaker and A. R. Boccaccini, In vitro attachment of staphylococcus epidermidis to surgical sutures with and without Ag-containing bioactive glass coating, J. Biomater. Appl., 2004, 19, 47–57 CrossRef CAS PubMed
.
- M. Catauro, M. G. Raucci, F. De Gaetano and A. Marotta, Antibacterial and bioactive silver-containing Na2OxCaOx2SiO2 glass prepared by sol–gel method, J. Mater. Sci.: Mater. Med., 2004, 15, 831–837 CrossRef CAS PubMed
.
- A. Balamurugan, G. Balossier, S. Kannan, J. Michel, A. H. S. Rebelo and J. M. F. Fereira, Development and in vitro characterization of sol–gel derived CaO–P2O5–SiO2–ZnO bioglass, Acta Biomater., 2007, 3, 255–262 CrossRef CAS PubMed
.
- L. Courtheoux, J. Lao, J. M. Nedelec and E. Jallot, Controlled bioactivity in zinc-doped sol–gel-derived binary bioactive glasses, J. Phys. Chem. C, 2008, 112, 13663–13667 CrossRef CAS
.
- V. Aina, F. Banino, C. Morterra, M. Miola, C. L. Bianchi, G. Malavasi, M. Marchetti and V. Bolis, Influence of the chemical composition on nature and activity of the surface layer of Zn-substituted sol–gel (bioactive) glasses, J. Phys. Chem. C, 2011, 115, 2196–2210 CrossRef CAS
.
- V. Aina, G. Malavasi, A. F. Pla, L. Munaron and C. Morterra, Zinc-containing bioactive glasses: surface reactivity and behaviour towards endothelial cells, Acta Biomater., 2009, 5, 1211–1222 CrossRef CAS PubMed
.
- J. Isaac, J. Nohra, J. Lao, E. Jallot, J. M. Nedelec, A. Berdal and J. M. Sautier, Effects of strontium doped bioactive glass on the differentiation of cultured osteogenic cells, Eur. Cells Mater., 2011, 21, 130–143 CrossRef CAS PubMed
.
- A. J. Leite, A. I. Gonclaves, M. T. Rodrigues, M. E. Gomes and J. F. Mano, Strontium-doped bioactive glass nanoparticles in osteogenic commitment, ACS Appl. Mater. Interfaces, 2018, 10, 23311–23320 CrossRef CAS PubMed
.
- P. Naruphontjirakul, A. E. Porter and J. R. Jones, In vitro osteogenesis by intracellular uptake of strontium containing bioactive glass nanoparticles, Acta Biomater., 2018, 66, 67–80 CrossRef CAS PubMed
.
- S. Herasaki, M. Alizadeh, H. Nazarian and D. Sharifi, Physico-chemical and in vitro biological evaluation of strontium/calcium silicophosphate glass, J. Mater. Sci.: Mater. Med., 2010, 21, 695–705 CrossRef
.
- K. L. Skelton, J. V. Glenn, S. A. Clarke, G. Georgiou, S. P. Valappil, J. C. Knowles, S. N. Nazhat and G. R. Jordan, Effect of ternary phosphate-based glass compositions on osteoblast and osteoblast-like proliferation, differentiation and death in vitro, Acta Biomater., 2007, 3, 563–572 CrossRef CAS PubMed
.
- R. K. Brow, Review: the structure of simple phosphate glasses, J. Non-Cryst. Solids, 2000, 263–264, 1–28 CrossRef
.
- V. Rajendran, A. V. G. Devi, M. Azooz and F. H. El-Batal, Physicochemical studies of phosphate based P2O5–Na2O–CaO–TiO2 glasses for biomedical applications, J. Non-Cryst. Solids, 2006, 353, 77–84 CrossRef
.
- E. A. Abou Neel, I. Ahmed, J. Pratten, S. N. Nazhat and J. C. Knowles, Characterisation of antibacterial copper releasing degradable phosphate glass fibres, Biomaterials, 2005, 26, 2247–2254 CrossRef CAS PubMed
.
- E. A. Abou Neel, L. A. O'Dell, M. E. Smith and J. C. Knowles, Processing, characterisation, and biocompatibility of zinc modified metaphosphate based glasses for biomedical applications, J. Mater. Sci.: Mater. Med., 2008, 19, 1669–1679 CrossRef CAS PubMed
.
- I. Ahmed, C. A. Collins, M. P. Lewis, I. Olsen and J. C. Knowles, Processing, characterisation and biocompatibility of iron-phosphate glass fibres for tissue engineering, Biomaterials, 2004, 25, 3223–3232 CrossRef CAS PubMed
.
- R. Shah, A. C. M. Sinanam, J. C. Knowles, N. P. Hunt and M. P. Lewis, Craniofacial muscle engineering using a 3-dimensional phosphate glass fibre construct, Biomaterials, 2005, 26, 1497–1505 CrossRef CAS PubMed
.
- J. C. Knowles, Phosphate based glasses for biomedical applications, J. Mater. Chem., 2003, 13, 2395–2401 RSC
.
- D. Carta, J. C. Knowles, M. E. Smith and R. J. Newport, Synthesis and structural characterization of P2O5–CaO–Na2O sol–gel materials, J. Non-Cryst. Solids, 2007, 353, 1141–1149 CrossRef CAS
.
- D. M. Pickup, P. Guerry, R. M. Moss, J. C. Knowles, M. E. Smith and R. J. Newport, New sol–gel synthesis of a (CaO)0.3(Na2O)0.2(P2O5)0.5 bioresorbable glass and its structural characterization, J. Mater. Chem., 2007, 17, 4777–4784 RSC
.
- D. M. Pickup, R. J. Speight, J. C. Knowles, M. E. Smith and R. J. Newport, Sol–gel synthesis and structural characterisation of binary TiO2–P2O5 glasses, Mater. Res. Bull., 2008, 43, 333–342 CrossRef CAS
.
- D. M. Pickup, R. J. Newport and J. C. Knowles, Sol–gel phosphate-based glass for drug delivery applications, J. Biomater. Appl., 2012, 26, 613–622 CrossRef CAS PubMed
.
- E. A. Abou Neel, W. Chrzanowski, S. P. Vlappil, L. A. O'Dell, D. M. Pickup, M. E. Smith, R. J. Newport and J. C. Knowles, Doping of a high calcium oxide metaphosphate glass with titanium dioxide, J. Non-Cryst. Solids, 2009, 355, 991–1000 CrossRef CAS
.
- E. A. Abou Neel and J. C. Knowles, Physical and biocompatibility studies of novel titanium dioxide doped phosphate-based glasses for bone tissue engineering applications, J. Mater. Sci.: Mater. Med., 2008, 19, 377–386 CrossRef CAS PubMed
.
- E. A. Abou Neel, T. Mizoguchi, M. Ito, M. Bitar, V. Salih and J. C. Knowles, In vitro bioactivity and gene expression by cells cultured on titanium dioxide doped phosphate-based glasses, Biomaterials, 2007, 28, 2967–2977 CrossRef CAS PubMed
.
- E. A. Abou Neel, A. M. Young, S. N. Nazhat and J. C. Knowles, A facile synthesis route to prepare microtubes from phosphate glass fibres, Adv. Mater., 2007, 19, 2856–2862 CrossRef
.
- J. C. Guedes, J. H. Park, N. J. Lakhkar, H. W. Kim, J. C. Knowles and I. B. Wall, TiO2-doped phosphate glass microcarriers: a stable bioactive substrate for expansion of adherent mammalian cells, J. Biomater. Appl., 2013, 28, 3–11 CrossRef CAS PubMed
.
- M. Bitar, V. Salih, J. C. Knowles and M. P. Lewis, Iron-phosphate glass fiber scaffolds for the hard–soft interface regeneration: the effect of fiber diameter and flow culture condition on cell survival and differentiation, J. Biomed. Mater. Res., Part A, 2008, 87, 1017–1026 CrossRef PubMed
.
- C. Vitale-Brovarone, G. Novajra, J. Lousteau, D. Milanese, S. Raimondo and M. Fornaro, Phosphate glass fibres and their role in neuronal polarization and axonal growth direction, Acta Biomater., 2012, 8, 1125–1136 CrossRef CAS PubMed
.
- E. A. Abou Neel, D. M. Pickup, S. P. Valappil, R. J. Newport and J. C. Knowles, Bioactive functional materials: a perspective on phosphate-based glasses, J. Mater. Chem. B, 2009, 19, 690–701 RSC
.
- F. F. Sene, J. R. Martinelli and E. Okumo, Synthesis and characterization of phosphate glass microspheres for radiotherapy applications, J. Non-Cryst. Solids, 2008, 354, 4887–4893 CrossRef CAS
.
- S. N. Nazhat, E. A. Abou Neel, A. Kidane, I. Ahmed, C. Hope, M. Kershaw, P. D. Lee, E. Stride, N. Saffari, J. C. Knowles and R. A. Brown, Controlled microchannelling in dense collagen scaffolds by soluble phosphate glass fibers, Biomacromol, 2006, 8, 543–551 CrossRef PubMed
.
- I. Ahmed, P. S. Cronin, E. A. Abou Neel, A. J. Parsons, J. C. Knowles and C. D. Rudd, Retention of mechanical properties and cytocompatibility of a phosphate-based glass fiber/polylactic acid composite, J. Biomed. Mater. Res., Part B, 2009, 89, 18–27 CrossRef CAS PubMed
.
- V. Salih, K. Franks, M. James, G. W. Hastings, J. C. Knowles and I. Olsen, Development of soluble glasses for biomedical use Part II: the biological response of human osteoblast cell lines to phosphate-based soluble glasses, J. Mater. Sci.: Mater. Med., 2000, 11, 615–620 CrossRef CAS PubMed
.
- M. Bitar, V. Salih, V. Mudera, J. C. Knowles and M. P. Lewis, Soluble phosphate glasses: in vitro studies using human cells of hard and soft tissue origin, Biomaterials, 2004, 25, 2283–2292 CrossRef CAS PubMed
.
- J. E. Gough, P. Christian, C. A. Scotchford, C. D. Rudd and I. A. Jones, Synthesis, degradation, and in vitro cell responses of sodium phosphate glasses for craniofacial bone repair, Biomed. Mater. Res., 2002, 59, 481–489 CrossRef CAS PubMed
.
- S. V. Dorozhkin and M. Epple, Biological and medical significance of calcium phosphates, Angew. Chem., 2002, 41, 3130–3146 CrossRef CAS
.
- J. M. Bouler, R. Z. LeGeros and G. Daculsi, Biphasic calcium phosphates: influence of three synthesis parameters on the HA/β-TCP ratio, J. Biomed. Mater. Res., 2000, 51, 680–684 CrossRef CAS PubMed
.
- M. Vallet-Regi and D. Arcos, Silicon substituted hydroxyapatites. A method to upgrade calcium phosphate based implants, J. Mater. Chem., 2005, 15, 1509–1516 RSC
.
- S. P. Valappil, D. M. Pickup, D. L. Carroll, C. K. Hope, J. Pratten, R. J. Newport, M. E. Smith, M. Wilson and J. C. Knowles, Effect of silver content on the structure and antibacterial activity of silver-doped phosphate-based glasses, Antimicrob. Agents Chemother., 2007, 51, 4453–4461 CrossRef CAS PubMed
.
- J. K. Christie, R. I. Ainsworth and N. H. de Leeuw, Ab initio molecular dynamics simulations of structural changes associated with the incorporation of fluorine in bioactive phosphate glasses, Biomaterials, 2014, 35, 6164–6171 CrossRef CAS PubMed
.
- N. J. Lahkar, E. A. Abou Neel, V. Salih and J. C. Knowles, Strontium oxide doped quaternary glasses: effect on structure, degradation and cytocompatibility, J. Mater. Sci.: Mater. Med., 2009, 20, 1339–1346 CrossRef PubMed
.
- K. M. Z. Hussain, U. Patel, A. R. Kennedy, M. P. Laura, V. Sottile, D. M. Grant, B. E. Scammell and I. Ahmed, Porous calcium phosphate glass microspheres for orthobiologic applications, Acta Biomater., 2018, 72, 396–406 CrossRef PubMed
.
- S. Maeno, Y. Niki, H. Matsumoto, H. Morioka, T. Yatabe, A. Funayama, Y. Toyama, T. Taguchi and J. Tanaka, The effect of calcium ion concentration on osteoblast viability, proliferation and differentiation in monolayer and 3D culture, Biomaterials, 2005, 26, 4847–4855 CrossRef CAS PubMed
.
- S. Yoshizawa, A. Brown, A. Bachowsky and C. Sfeir, Role of magnesium ions on osteogenic response in bone marrow stromal cells, Connect. Tissue Res., 2014, 55, 155–159 CrossRef CAS PubMed
.
- M. Julien, S. Khoshniat, A. Lacreusette, M. Gatius, A. Bozec, E. F. Wagner, Y. Wittrant, M. Masson, P. Weiss, L. Beck, D. Magne and J. Guicheux, Phosphate-dependent regulation of MGP in osteoblasts: role of ERK1/2 and Fra-1, J. Bone Miner. Res., 2009, 24, 1856–1868 CrossRef CAS PubMed
.
-
A. Michael-Titus, P. Revest and P. Shortland, Elements of cellular and molecular neurosciences, in The nervous system, 2nd Edition, 2010, pp. 31–46 Search PubMed
.
- U. Patel, R. M. Moss, K. M. Z. Hossain, A. R. Kennedy, E. R. Barney, I. Ahmed and A. C. Hannon, Structural and physico-chemical analysis of calcium/strontium substituted, near-invert phosphate based glasses for biomedical applications, Acta Biomater., 2017, 60, 109–127 CrossRef CAS PubMed
.
- I. Ahmed, E. A. Abou Neel, S. P. Valappil, S. N. Nazhat, D. M. Pickup, D. Carta, D. L. Carroll, R. J. Newport, M. E. Smith and J. C. Knowles, The structure and properties of silver-doped phosphate-based glasses, J. Mater. Sci., 2007, 42, 9827–9835 CrossRef CAS
.
- I. Ahmed, D. Reddy, M. Wilson and J. C. Knowles, Antimicrobial effect of silver-doped phosphate-based glasses, J. Biomed. Mater. Res., Part A, 2006, 79, 618–626 CrossRef CAS PubMed
.
- V. Mourino, J. P. Cattalini and A. R. Boccaccini, Metallic ions as therapeutic agents in tissue engineering scaffolds: an overview of their biological applications and strategies for new developments, J. R. Soc., Interface, 2012, 9, 401–419 CrossRef CAS PubMed
.
- L. Weng, S. K. Boda, M. J. Teusink, F. D. Shuler, X. Li and J. Xie, Binary doping of strontium and copper enhancing osteogenesis and angiogenesis of bioactive glass nanofibers while suppressing osteoclast activity, ACS Appl. Mater. Interfaces, 2017, 9, 24484–24496 CrossRef CAS PubMed
.
- H. Zhu, Z. Pan, B. Chen, B. Lee, S. M. Mahurin, S. H. Overbury and S. Dai, Synthesis of ordered mixed titania and silica mesostructured monoliths for gold catalysts, J. Phys. Chem. B, 2004, 108, 20038–20044 CrossRef CAS
.
- J. Tang, J. Liu, J. Yang, Z. Feng, F. Fan and Q. Yang, Mesoporous titanosilicates with high loading of titanium synthesized in mild acidic buffer solution, J. Colloid Interface Sci., 2009, 335, 203–209 CrossRef CAS PubMed
.
- W. I. Kim and I. K. J. Hong, Synthesis of monolithic titania–silica composite aerogels with supercritical drying process, J. Ind. Eng. Chem., 2003, 9, 728–734 CAS
.
- J. A. Melero, J. M. Arsuaga, P. de Frutos, J. Iglesias, J. Sainz and S. Blazquez, Direct synthesis of titanium-substituted mesostructured materials using non-ionic surfactants and titanocene dichloride, Microporous Mesoporous Mater., 2005, 86, 364–373 CrossRef CAS
.
- O. Ruzimuradov, S. Nurmanov, Y. Kodani, R. Takahashi and I. Yamada, Morphology and dispersion control of titania–silica monolith with macro–meso pore system, J. Sol-Gel Sci. Technol., 2012, 64, 684–693 CrossRef CAS
.
- W. H. Zhang, J. Lu, B. Han, M. Li, J. Xiu, P. Ying and C. Li, Direct synthesis and characterization of titanium-substituted mesoporous molecular sieve SBA-15, Chem. Mater., 2002, 14, 3413–3421 CrossRef CAS
.
- G. Liu, Y. Liu, G. Yang, S. Li, Y. Zu, W. Zhang and M. Jia, Preparation of titania–silica mixed oxides by a sol–gel route in the presence of citric acid, J. Phys. Chem. C, 2009, 113, 9345–9351 CrossRef CAS
.
- H. J. Chen, L. Wang and W. Y. Chiu, Chelation and solvent effect on the preparation of titania colloids, Mater. Chem. Phys., 2007, 101, 12–19 CrossRef CAS
.
- W. Rupp, N. Husing and U. Schubert, Preparation of silica–titania xerogels and aerogels by sol–gel processing of new single-source precursors, J. Mater. Chem., 2002, 12, 2594–2596 RSC
.
- R. F. Lenza and W. L. Vasconcelos, Synthesis of titania–silica materials by sol–gel, Mater. Res., 2002, 5, 497–502 CrossRef
.
- J. Konishi, K. Fujita, K. Nakanishi and K. Hirao, Monolithic TiO2 with controlled multiscale porosity via a template-free sol–gel process accompanied by phase separation, Chem. Mater., 2006, 18, 6069–6074 CrossRef CAS
.
- K. Nakanishi and N. Tanaka, Sol–gel with phase separation. Hierarchically porous materials optimized for high-performance liquid chromatography separations, Acc. Chem. Res., 2007, 40, 863–873 CrossRef CAS PubMed
.
- O. N. Ruzimuradov, Formation of bimodal porous silica–titania monoliths by sol–gel route, IOP Conf. Ser.: Mater. Sci. Eng., 2011, 18, 032004 Search PubMed
.
- K. Deshmukh, T. Kovarik, T. Krenek, T. Stitch and D. Docheva, Microstructural evaluation and thermal properties of sol–gel derived silica–titania based porous glasses, J. Phys.: Conf. Ser., 2020, 1527, 012031 CrossRef CAS
.
- K. Nakanishi, Preparation of SiO2–TiO2 gels with controlled pore structure via sol–gel route, Bull. Inst. Chem. Res., Kyoto Univ., 1992, 70, 144–151 CAS
.
- S. Flaig, J. Akbarzadeh, P. Dolcet, S. Gross, H. Peterlik and N. Husing, Hierarchically organized silica–titania monoliths prepared under purely aqueous conditions, Chem.–Eur. J., 2014, 20, 17409–17419 CrossRef CAS PubMed
.
- P. R. Aravind, P. Shjesh, P. Mukundan and K. G. K. Warrier, Silica–titania aerogel monoliths with large pore volume and surface area by ambient pressure drying, J. Sol-Gel Sci. Technol., 2009, 52, 328–334 CrossRef CAS
.
- J. F. Destino, N. A. Dudukovic, M. A. Johnson, D. T. Nguyen, T. D. Yee, G. C. Egan, A. M. Sawvel, W. A. Steele, T. F. Baumann, E. B. Duoss, T. Suratwala and R. Dylla-Spears, 3D printed optical quality silica and silica–titania glasses from sol–gel feedstocks, Adv. Mater. Technol., 2018, 3, 1700323 CrossRef
.
- G. Scannell, A. Koike and L. Hunag, Structure and thermomechanical response of TiO2–SiO2 glasses to temperature, J. Non-Cryst. Solids, 2016, 447, 238–247 CrossRef CAS
.
- G. Brusatin, M. Guglielmi, P. Innocenzi, A. Martucci, G. Battaglin, S. Pelli and G. Righini, Microstructural and optical properties of sol–gel silica–titania waveguides, J. Non-Cryst. Solids, 1997, 220, 202–209 CrossRef CAS
.
- K. Shingyouchi and S. Konishi, Gradient-index doped silica rod lenses produced by a sol gel method, Appl. Opt., 1990, 29, 4061–4063 CrossRef CAS PubMed
.
- Z. Deng, J. Wang, Y. Zhang, Z. Weng, Z. Zhang, B. Zhou, J. Shen and L. Chen, Preparation and photocatalytic activity of TiO2–SiO2 binary aerogels, Nanostruct. Mater., 1999, 11, 1313–1318 CrossRef CAS
.
- D. C. M. Dutoit, M. Schneider and A. Baiker, Titania-silica mixed oxides: I. Influence of sol–gel and drying conditions on structural properties, J. Catal., 1995, 153, 165–176 CrossRef CAS
.
- Z. Xu, Y. Jia, Z. Hao, M. Liu and L. Chen, Preparation and characterization of silica–titania aerogel-like balls by ambient pressure drying, J. Sol-Gel Sci. Technol., 2007, 41, 203–207 CrossRef CAS
.
- B. Malinowska, J. Walendziewski, D. Robert, J. V. Weber and M. Stolarski, The study of photocatalytic activities of titania and titania–silica aerogels, Appl. Catal., B, 2003, 46, 441–451 CrossRef CAS
.
- Z. Deng, E. Breval and C. G. Pantano, Colloidal sol/gel processing of ultra-low expansion TiO2/SiO2 glasses, J. Non-Cryst. Solids, 1988, 100, 364–370 CrossRef CAS
.
- S. Satoh, K. Susa and I. Matsuyama, Sol–gel derived binary silica glasses with high refractive index, J. Non-Cryst. Solids, 1992, 146, 121–128 CrossRef CAS
.
- S. M. El-Bashir, Physical properties Nd3+ doped (SiO2–TiO2) monolithic glass for photoresistor applications, Mater. Res. Express, 2017, 4, 115203 CrossRef
.
-
L. Guangwu and L. Yangang, Preparation and characterization of silica–titania aerogel monoliths by sol gel method, IEEE 16th International Conference on Nanotechnology (IEEE-NANO), Sendai, Japan, August 22–25, 2016, pp. 85–88 Search PubMed
.
- S. Pandey and S. B. Mishra, Sol–gel derived organic–inorganic hybrid materials: synthesis, characterizations and applications, J. Sol-Gel Sci. Technol., 2011, 59, 73–94 CrossRef CAS
.
- S. H. Mir, L. A. Nagahara, T. Thundat, P. Mokarian-Tabari, H. Furukawa and A. Khosla, Review – organic–inorganic hybrid functional materials: an integrated platform for applied technologies, J. Electrochem. Soc., 2018, 165, B3137–B3156 CrossRef CAS
.
-
G. Kickelbick, Introduction to hybrid materials, in Hybrid materials: synthesis, characterization and applications, ed. G. Kickelbick, Wiley-VCH Verlag, GmbH & Co KGaA, Weinheim, 2007, pp. 1–48 Search PubMed
.
- F. Baino, E. Fiume, M. Miola and E. Verne, Bioactive sol–gel glasses: processing, properties, and applications, Int. J. Appl. Ceram. Technol., 2018, 15, 841–860 CrossRef CAS
.
- I. Gill, Bio-doped nanocomposite polymers: sol–gel bioencapsulates, Chem. Mater., 2001, 13, 3404–3421 CrossRef CAS
.
- E. M. Vallient and J. R. Jones, Softening bioactive glass for bone regeneration: sol–gel hybrid materials, Soft Matter, 2011, 7, 5083–5095 RSC
.
- I. Crouzet and D. Leclercq, Polyphosphazene–metal oxide hybrids by nonhydrolytic sol–gel processes, J. Mater. Chem., 2000, 10, 1195–1201 RSC
.
- R. Tamaki, K. Naka and Y. Chujo, Synthesis of poly(N,N-dimethylacrylamide)/silica gel polymer hybrids by in situ polymerization method, Polym. J., 1998, 30, 60–65 CrossRef CAS
.
- M. Toki, T. Y. Chow, T. Ohnaka, H. Samura and T. Saegusa, Structure of poly(vinylpyrrolidone)–silica hybrid, Polym. Bull., 1992, 29, 653–660 CrossRef
.
- K. Landfester, Miniemulsion polymerization and the structure of polymer and hybrid nanoparticles, Angew. Chem., 2009, 48, 4488–4507 CrossRef CAS PubMed
.
- F. Yang and G. L. Nelson, PMMA/silica nanocomposite studies: synthesis and properties, J. Appl. Polym. Sci., 2004, 91, 3844–3850 CrossRef CAS
.
- K. A. Mauritz and C. K. Jones, Novel poly (n-butyl methacrylate)/titanium oxide alloys produced by sol–gel process for titanium alkoxides, J. Appl. Polym. Sci., 1990, 40, 1401–1420 CrossRef CAS
.
- X. Zhao, Y. Wu, Y. Du, X. Chen, B. Lei, Y. Xue and P. X. Ma, A highly bioactive and biodegradable poly(glycerolsebacate)–silica glass hybrid elastomer with tailored mechanical properties for bone tissue regeneration, J. Mater. Chem. B, 2015, 3, 3222–3233 RSC
.
- M. Kamitakahara, M. Kawashita, N. Miyata, T. Kokubo and T. Nakamura, Bioactivity and mechanical properties of polydimethylsiloxane (PDMS)–CaO–SiO2 hybrids with different calcium contents, J. Mater. Sci.: Mater. Med., 2002, 13, 1015–1020 CrossRef CAS PubMed
.
- D. Sanchez-Tellez, L. Tellez-Jurado and L. M. Rodriguez-Lorenzo, Optimization of the CaO and P2O5 contents on PDMS–SiO2–CaO–P2O5 hybrids intended for bone regeneration, J. Mater. Sci., 2015, 50, 5993–6006 CrossRef CAS
.
- A. P. V. Pereira, W. L. Vasconcelos and R. L. Orefice, Novel multicomponent silicate–poly(vinyl alcohol) hybrids with controlled reactivity, J. Non-Cryst. Solids, 2000, 273, 180–185 CrossRef CAS
.
- A. I. Martin, A. J. Salinas and M. Vallet-Regi, Bioactive and degradable organic–inorganic hybrids, J. Eur. Ceram. Soc., 2005, 25, 3533–3538 CrossRef CAS
.
- M. Catauro, F. Bollino, F. Papale, M. Gallicchio and S. Pacifico, Influence of the polymer amount on bioactivity and biocompatibility of SiO2/PEG hybrid materials synthesized by sol–gel technique, Mater. Sci. Eng., C, 2015, 48, 548–555 CrossRef CAS PubMed
.
- J. H. Song, B. H. Yoon, H. E. Kim and H. W. Kim, Bioactive and degradable hybridized nanofibers of gelatin–siloxane for bone regeneration, J. Biomed. Mater. Res., Part A, 2008, 84, 875–884 CrossRef PubMed
.
- E. J. Lee, S. H. Jun, H. E. Kim, H. W. Kim, Y. H. Koh and J. H. Jang, Silica xerogel–chitosan nano-hybrids for use as drug eluting bone replacement, J. Mater. Sci.: Mater. Med., 2010, 21, 207–214 CrossRef CAS PubMed
.
- E. J. Lee, D. S. Shin, H. E. Kim, H. W. Kim, Y. H. Koh and J. H. Jang, Membrane of hybrid chitosan–silica xerogel for guided bone regeneration, Biomaterials, 2009, 30, 743–750 CrossRef CAS PubMed
.
- E. J. Lee, S. H. Teng, T. S. Jang, P. Wang, S. W. Yook, H. E. Kim and Y. H. Koh, Nanostructured poly(ε-caprolactone)–silica xerogel fibrous membrane for guided bone regeneration, Acta Biomater., 2010, 6, 3557–3565 CrossRef CAS PubMed
.
- M. Catauro, F. Bollino, F. Papale, S. Marciano and S. Pacifico, TiO2/PCL hybrid materials synthesized via sol–gel technique for biomedical applications, Mater. Sci. Eng., C, 2015, 47, 135–141 CrossRef CAS PubMed
.
- M. Catauro, F. Bollino, F. Papale, P. Mozetic, A. Rainer and M. Trombetta, Biological response of human mesenchymal stromal cells to titanium grade 4 implants coated with PCL/ZrO2 hybrid materials synthesized by sol–gel route: in vitro evaluation, Mater. Sci. Eng., C, 2014, 45, 395–401 CrossRef CAS PubMed
.
- L. Ren, K. Tsuru, S. Hayakawa and A. Osaka, Sol–gel preparation and in vitro deposition of apatite on porous gelatin–siloxane hybrids, J. Non-Cryst. Solids, 2001, 285, 116–122 CrossRef CAS
.
- L. Ren, K. Tsuru, S. Hayakawa and A. Osaka, Novel approach to fabricate porous gelatin–siloxane hybrids for bone tissue engineering, Biomaterials, 2002, 23, 4765–4773 CrossRef CAS PubMed
.
- S. S. Silva, R. A. S. Ferreira, L. Fu, L. D. Carlos, J. F. Mano, R. L. Reis and J. Rocha, Functional nanostructured chitosan–siloxane hybrids, J. Mater. Chem., 2005, 15, 3952–3961 RSC
.
- C. Ohtsuki, T. Miyazaki and M. Tanihara, Development of bioactive organic–inorganic hybrid for bone substitutes, Mater. Sci. Eng., C, 2002, 22, 27–34 CrossRef
.
- R. Gupta and A. Kumar, Bioactive materials for biomedical applications using sol–gel technology, Biomed. Mater., 2008, 3, 034005 CrossRef PubMed
.
- B. Szczesniak, J. Choma and M. Joroniec, Major advances in the development of ordered mesoporous materials, Chem. Commun., 2020, 56, 7836–7848 RSC
.
- M. Thommes, K. Kaneko, A. V. Neimark, J. P. Olivier, F. Rodriguez-Reinoso, J. Rouquerol and K. S. W. Sing, Physisorption of gases, with special reference to the evaluation of surface area and pore size distribution (IUPAC Technical Report), Pure Appl. Chem., 2015, 87, 1051–1069 CrossRef CAS
.
- J. R. Jones, P. D. Lee and L. L. Hench, Hierarchical porous materials for tissue engineering, Philos. Trans. R. Soc., A, 2006, 364, 263–281 CrossRef CAS PubMed
.
- N. Pal and A. Bhaumik, Soft templating strategies for the synthesis of mesoporous materials: inorganic, organic–inorganic hybrids and purely organic solids, Adv. Colloid Interface Sci., 2013, 189–190, 21–41 CrossRef CAS PubMed
.
- D. Arcos and M. Vallet-Regi, Bioceramics for drug delivery, Acta Mater., 2013, 61, 890–911 CrossRef CAS
.
- C. Wu and J. Chang, Mesoporous bioactive glasses: structure characteristics, drug/growth factor delivery and bone regeneration application, Interface Focus, 2012, 2, 292–306 CrossRef PubMed
.
- P. Horcajada, A. Ramila, J. Perez-Pariente and M. Vallet-Regi, Influence of pore size of MCM-41 matrices on drug delivery rate, Microporous Mesoporous Mater., 2004, 68, 105–109 CrossRef CAS
.
- Y. Li, B. P. Bastakoti and Y. Yamauchi, Smart soft-templating synthesis of hollow mesoporous bioactive glass spheres, Chem.–Eur. J., 2015, 21, 8038–8042 CrossRef CAS PubMed
.
- G. S. Pappas, P. Liatsi, I. A. Kartsonakis, I. Danilidis and G. Kordas, Synthesis and characterization of new SiO2–CaO hollow nanospheres by sol–gel method: bioactivity of the new system, J. Non-Cryst. Solids, 2008, 354, 755–760 CrossRef CAS
.
- K. Zheng, J. A. Bortuzzo, Y. Liu, W. Li, M. Pischetsrieder, J. Roether, M. Lu and A. R. Boccaccini, Bio-templated bioactive glass particles with hierarchical macro–nano porous structure and drug delivery capability, Colloids Surf., B, 2015, 135, 825–832 CrossRef CAS PubMed
.
- X. Li, M. Guohou, C. Xiaofeng, Y. Guang, L. Hui, M. Cong, S. Xiongjun and N. Chengyun, Investigation of radial mesoporous bioactive glass particles as drug carriers for inhibition of tumor cells, Sci. Adv. Mater., 2017, 9, 562–570 CrossRef
.
- D. Arcos, A. Lopez-Noriega, E. Ruiz-Hernandez, O. Terasaki and M. Vallet-Regi, Ordered mesoporous microspheres for bone grafting and drug delivery, Chem. Mater., 2009, 21, 1000–1009 CrossRef CAS
.
- K. Zheng, M. Lu, B. Rutkowski, X. Dai, Y. Yang, N. Taccardi, U. Stachewicz, A. Czyrska-Filemonowicz, N. Huser and A. R. Boccaccini, ZnO quantum dots modified bioactive glass nanoparticles with pH-sensitive release of Zn ions, fluorescence, antibacterial and osteogenic properties, J. Mater. Chem. B, 2016, 4, 7936–7949 RSC
.
- O. Tsigkou, S. Labbaf, M. M. Stevens, A. E. Porter and J. R. Jones, Monodispersed bioactive glass submicron particles and their effect on bone marrow and adipose tissue-derived stem cells, Adv. Healthcare Mater., 2014, 3, 115–125 CrossRef CAS PubMed
.
- Q. Hu, Y. Li, G. Miao, N. Zhao and X. Chen, Size control and biological properties of monodispersed mesoporous bioactive glass sub-micron spheres, RSC Adv., 2014, 4, 22678–22687 RSC
.
- P. Kolhar, A. C. Anselmo, V. Gupta, K. Pant, B. Prabhakarpandian, E. Ruoslahti and S. Mitragotri, Using shape effects to target antibody-coated nanoparticles to lung and brain endothelium, Proc. Natl. Acad. Sci. U. S. A., 2013, 110, 10753–10758 CrossRef CAS PubMed
.
- R. Colquhoun and K. E. Tanner, Mechanical behaviour of degradable phosphate glass fibres and composites: a review, Biomed. Mater., 2015, 11, 14105 CrossRef CAS PubMed
.
- Y. Li, X. Chen, C. Ning, B. Yuan and Q. Hu, Facile synthesis of mesoporous bioactive glasses with controlled shapes, Mater. Lett., 2015, 161, 605–608 CrossRef CAS
.
- E. El-Meliegy, M. Mabrouk, S. A. M. El-Sayed, B. M. Abd El-Hady, M. R. Shehata and W. M. Hosny, Novel Fe2O3-doped glass/chitosan scaffolds for bone tissue replacement, Ceram. Int., 2018, 44, 9140–9151 CrossRef CAS
.
- S. A. M. El-Sayed, M. Mabrouk, M. E. Khallaf, B. M. Abd El-Hady, E. El-Meliegy and M. R. Shehata, Antibacterial, drug delivery, and osteoinduction abilities of bioglass/chitosan scaffolds for dental applications, J. Drug Delivery Sci. Technol., 2020, 57, 101757 CrossRef CAS
.
- F. Baino, E. Fiume, M. Miola, F. Leone, B. Onida and E. Verne, Fe-doped bioactive glass-derived scaffolds produced by sol–gel foaming, Mater. Lett., 2019, 235, 207–211 CrossRef CAS
.
- T. Charoensuk, C. Sirisathitkul, U. Boonyang, I. J. Macha, J. Santos, D. Grossin and B. Ben-Nissan, In vitro bioactivity and stem cells attachment of three-dimensionally ordered macroporous bioactive glass incorporating iron oxides, J. Non-Cryst. Solids, 2016, 452, 62–73 CrossRef CAS
.
- J. Mesquita-Guimarães, L. Ramos, R. Detsch, B. Henriques, M. C. Fredel, F. S. Silva and A. R. Boccaccini, Evaluation of in vitro properties of 3D micro–macro porous zirconia scaffolds coated with 58S bioactive glass using MG-63 osteoblast-like cells, J. Eur. Ceram. Soc., 2019, 39, 2545–2558 CrossRef
.
- S. Ni, X. Li, P. Yang, S. Ni, F. Hong and T. J. Webster, Enhanced apatite-forming ability and antibacterial activity of porous anodic alumina embedded with CaO–SiO2–Ag2O bioactive materials, Mater. Sci. Eng., C, 2016, 58, 700–708 CrossRef CAS PubMed
.
- M. Yazdimamaghani, D. Vashae, S. Assefa, K. J. Walker, S. V. Madihally, G. A. Kohler and L. Tayebi, Hybrid macroporous gelatin/bioactive-glass/nanosilver scaffolds with controlled degradation behavior and antimicrobial activity for bone tissue engineering, J. Biomed. Nanotechnol., 2014, 10(6), 911–931 CrossRef CAS PubMed
.
- F. Sharifianjazi, N. Parvin and M. Tahriri, Synthesis and characteristics of sol–gel bioactive SiO2–P2O5–CaO–Ag2O glasses, J. Non-Cryst. Solids, 2017, 476, 108–113 CrossRef CAS
.
- S. Z. Jalise, N. Baheiraei and F. Bagheri, The effects of strontium incorporation on a novel gelatin/bioactive glass bone graft: in vitro and in vivo characterization, Ceram. Int., 2018, 44, 14217–14227 CrossRef
.
- M. Shaltooki, G. Dini and M. Mehdikhani, Fabrication of chitosan-coated porous polycaprolactone/strontium-substituted bioactive glass nanocomposite scaffold for bone tissue engineering, Mater. Sci. Eng., C, 2019, 105, 110138 CrossRef CAS PubMed
.
- S. Amudha, J. R. Ramya, K. T. Arul, A. Deepika, P. Sathiamurthi, B. Mohana, K. Asokan, C. L. Dong and S. N. Kalkura, Enhanced mechanical and biocompatible properties of strontium ions doped mesoporous bioactive glass, Composites, Part B, 2020, 196, 108099 CrossRef CAS
.
- D. Zamani, F. Moztarzadeh and D. Bizari, Alginate-bioactive glass containing Zn and Mg composite scaffolds for bone tissue engineering, Int. J. Biol. Macromol., 2019, 137, 1256–1267 CrossRef CAS PubMed
.
- Y. Hong, X. Chen, X. Jing, H. Fan, Z. Gu and X. Zhang, Fabrication and drug delivery of ultrathin mesoporous bioactive glass hollow fibers, Adv. Funct. Mater., 2010, 20, 1503–1510 CrossRef CAS
.
- G. Poologasundarampillai, D. Wang, S. Li, J. Nakamura, R. Bradley, P. D. Lee, M. M. Stevens, D. S. McPhail, T. Kasuga and J. R. Jones, Cotton–wool-like bioactive glasses for bone regeneration, Acta Biomater., 2014, 10, 3733–3746 CrossRef CAS PubMed
.
- F. Baino, G. Novajra, V. Miguez-Pacheco, A. R. Boccaccini and C. Vitale-Bovarone, Bioactive glasses: special applications outside the skeletal system, J. Non-Cryst. Solids, 2016, 432, 15–30 CrossRef CAS
.
- S. M. Rabie, N. Nazparvar, M. Azizian, D. Vashaee and L. Tayebi, Effect of ion substitution on properties of bioactive glasses: a review, Ceram. Int., 2015, 41, 7241–7251 CrossRef
.
- J. Faure, R. Drevet, A. Lemelle, N. B. Jaber, A. Tara, H. El Btaouri and H. Benhayoune, A new sol–gel synthesis of 45S5 bioactive glass
using an organic acid as catalyst, Mater. Sci. Eng., C, 2015, 47, 407–412 CrossRef CAS PubMed
.
- J. H. Lopes, O. M. V. M. Bueno, I. O. Mazali and C. A. Bertran, Investigation of citric acid-assisted sol–gel synthesis coupled to the self-propagating combustion method for preparing bioactive glass with high structural homogeneity, Mater. Sci. Eng., C, 2019, 97, 669–678 CrossRef CAS PubMed
.
- M. H. Thibault, C. Comeau, G. Vienneau, J. Robichaud, D. Brown, R. Bruening, L. J. Martin and Y. Djaoued, Assessing the potential of boronic acid/chitosan/bioglass composite, Mater. Sci. Eng., C, 2020, 110, 110674 CrossRef CAS PubMed
.
- L. L. Hench, The story of bioglass, J. Mater. Sci.: Mater. Med., 2006, 17, 967–978 CrossRef CAS PubMed
.
- F. Baino, Bioactive glass – when glass science and technology meet regenerative medicine, Ceram. Int., 2018, 44, 14953–14966 CrossRef CAS
.
-
L. Hupa, Composition–property relations of bioactive silicate glasses, in Bioactive glasses: materials properties and applications, ed. H. Ylanen, Woodhead Publishing, 2nd edn, 2018, pp. 1–36 Search PubMed
.
- A. M. Deliormanli, X. Liu and M. N. Rahaman, Evaluation of borate bioactive glass scaffolds with different pore sizes in a rat subcutaneous implantation model, J. Biomater. Appl., 2014, 28, 643–653 CrossRef PubMed
.
- F. A. Shah, D. S. Brauer, N. Desai, R. G. Hill and K. A. Hing, Fluoride-containing bioactive glasses and Bioglass® 45S5 form apatite in low pH cell culture medium, Mater. Lett., 2014, 119, 96–99 CrossRef CAS
.
- O. H. Andersson, G. Liu, K. H. Karlsson, L. Niemi, J. Miettinen and J. Juhanoja, In vivo behaviour of glasses in the SiO2–Na2O–CaO–P2O5–Al2O3–B2O3 system, J. Mater. Sci.: Mater. Med., 1990, 1, 219–227 CrossRef CAS
.
- M. Brink, T. Turunen, R. P. Happonen and A. Yli-Urpo, Compositional dependence of bioactivity of glasses in the system Na2O–K2O–MgO–CaO–B2O3–P2O5–SiO2, J. Biomed. Mater. Res., 1997, 37, 114–121 CrossRef CAS
.
- G. Lusvardi, G. Malavasi, L. Menabue and M. C. Menziani, Synthesis, characterization, and molecular dynamics simulation of Na2O–CaO–SiO2–ZnO glasses, J. Phys. Chem. B, 2002, 106, 9753–9760 CrossRef CAS
.
- A. W. Wren, A. Coughlan, C. M. Smith, S. P. Hudson, F. R. Laffir and M. R. Towler, Investigating the solubility and cytocompatibility of CaO–Na2O–SiO2/TiO2 bioactive glasses, J. Biomed. Mater. Res., Part A, 2015, 103, 709–720 CrossRef PubMed
.
- S. Pourshahrestani, E. Zeimaran, N. A. Kadri, N. Gargiulo, S. Samuel, S. V. Naveen, T. Kamarul and M. R. Towler, Gallium-containing mesoporous bioactive glass with potent hemostatic activity and antibacterial efficacy, J. Mater. Chem. B, 2016, 4, 71–86 RSC
.
- G. Kaur, O. P. Pandey, K. Singh, D. Homa, B. Scott and G. Pickrell, A review of bioactive glasses: their structure, properties, fabrication and apatite formation, J. Biomed. Mater. Res., Part A, 2014, 102, 254–274 CrossRef PubMed
.
- O. M. Goudouri, E. Kontonasaki, U. Lohbauer and A. R. Boccaccini, Antibacterial properties of metal and metalloid ions in chronic periodontitis and peri-implantitis therapy, Acta Biomater., 2014, 10, 3795–3810 CrossRef CAS PubMed
.
- X. Li, X. Wang, D. He and J. Shi, Synthesis and characterization of mesoporous CaO–MO–SiO2–P2O5 (M = Mg, Zn, Cu) bioactive glasses/composites, J. Mater. Chem., 2008, 18, 4103–4109 RSC
.
- E. Verne, S. D. Nunzio, M. Bosetti, P. Appendino, C. V. Brovarone, G. Maina and M. Cannas, Surface characterization of silver-doped bioactive glass, Biomaterials, 2005, 26, 5111–5119 CrossRef CAS PubMed
.
- M. Yamaguchi, Role of zinc in bone metabolism and preventive effect on bone disorder, Biomed. Res. Trace Elem., 2007, 18, 346–366 CAS
.
- D. Boyd, O. M. Clarkin, A. W. Wren and M. R. Towler, Zinc-based glass polyalkenoate cements with improved setting times and mechanical properties, Acta Biomater., 2008, 4, 425–431 CrossRef CAS PubMed
.
- H. Zreqat, S. M. Valenzuela, B. B. Nissan, R. Roest, C. Knabe, R. J. Radlanski, H. Renz and P. J. Evans, The effect of surface chemistry modification of titanium alloy on signalling pathways in human osteoblasts, J. Biomed. Mater. Res., 2002, 62, 175–184 CrossRef PubMed
.
- Y. Arima and H. Iwata, Effect of wettability and surface functional groups on protein adsorption and cell adhesion using well-defined mixed self-assembled monolayers, Biomaterials, 2007, 28, 4079–4087 CrossRef PubMed
.
- A. Hasan, S. K. Pattanayek and L. M. Pandey, Effect of functional groups of self assembled monolayers on protein adsorption and initial cell adhesion, ACS Biomater. Sci. Eng., 2018, 4, 3224–3233 CrossRef CAS PubMed
.
- K. Duan and R. Z. Wang, Surface modifications of bone implants through wet chemistry, J. Mater. Chem., 2006, 16, 2309–2321 RSC
.
-
J. Chang and Y. L. Zhou, Surface modification of bioactive glasses, in Bioactive glasses: materials properties and applications, ed. H. Ylanen, Woodhead Publishing, 2017, pp. 119–143 Search PubMed
.
- S. Kargozar, F. Kermani, S. M. Beidokhti, S. Hamzehlou, E. Verne, S. Ferraris and F. Baino, Functionalization and surface modification of bioactive glasses (BGs): tailoring the biological response working on the outermost surface layer, Materials, 2019, 12, 3696 CrossRef CAS PubMed
.
-
S. Ferraris and E. Verne, Surface functionalization of bioactive glasses: reactive groups, biomolecules, and drugs on bioactive surfaces for smart and functional biomaterials, in Bioactive glasses: fundamentals, technology and applications, ed. A. R. Boccaccini, D. S. Brauer and L. Hupa, The Royal Society of Chemistry, 2018, pp. 221–235 Search PubMed
.
- Z. Y. Qiu, C. Chen, X. M. Wang and I. S. Lee, Advances in the surface modification techniques of bone-related implants for last 10 years, Regener. Biomater., 2014, 1, 67–79 CrossRef PubMed
.
-
V. Stanic, Variation in properties of bioactive glasses after surface modification, in Clinical applications of biomaterials, ed. G. Kaur, Springer International Publishing AG, 2017, pp. 35–63 Search PubMed
.
- G. K. Towarfe, R. J. Composto and I. M. Shapiro, Nucleation and growth of calcium phosphate on amine-, carboxyl- and hydroxyl-silane self-assembled monolayers, Biomaterials, 2006, 27, 631–642 CrossRef PubMed
.
- P. Zucca and E. Sanjust, Inorganic materials as supports for covalent enzyme immobilization: methods and mechanisms, Molecules, 2014, 19, 14139–14194 CrossRef PubMed
.
- V. Aina, C. Magistris, G. Cerrato, G. Martra, G. Viscardi, G. Lusvardi, G. Malavasi and L. Menabue, New formulation of functionalized bioactive glasses to be used as carriers for the development of pH-stimuli responsive biomaterials for bone diseases, Langmuir, 2014, 30, 4703–4715 CrossRef CAS PubMed
.
- E. Verne, C. Vitale-Brovarone, E. Bui, C. L. Bianchi and A. R. Boccaccini, Surface functionalization of bioactive glasses, J. Biomed. Mater. Res., Part A, 2009, 90(4), 981–992 CrossRef CAS PubMed
.
- S. Ferraris, C. Vitale-Brovarone, O. Bretcanu, C. Cassinelli and E. Verne, Surface functionalization of 3D glass-ceramic porous scaffolds for enhanced mineralization in vitro, Appl. Surf. Sci., 2013, 271, 412–420 CrossRef CAS
.
- Q. Z. Chen, K. Rezwan, V. Francon, D. Armitage, S. N. Nazhat, F. H. Jones and A. R. Boccaccini, Surface functionalization of Bioglass®-derived porous scaffolds, Acta Biomater., 2007, 3, 551–562 CrossRef CAS PubMed
.
- A. Lopez-Noriega, D. Arcos and M. Vallet-Regi, Functionalizing mesoporous bioglasses for long-term anti-osteoporotic drug delivery, Chem.–Eur. J., 2010, 16, 10879–10886 CrossRef CAS PubMed
.
- A. El-Fiqi, J. H. Lee, E. J. Lee and H. W. Kim, Collagen hydrogels incorporated with surface-aminated mesoporous nanobioactive glass: improvement of physicochemical stability and mechanical properties is effective for hard tissue engineering, Acta Biomater., 2013, 9, 9503–9521 CrossRef PubMed
.
- E. Verne, S. Ferraris, C. Vitale-Brovarone, S. Spriano, C. L. Bianchi, A. Naldoni, M. Morra and C. Cassinelli, Alkaline phosphatase grafting on bioactive glasses and glass ceramics, Acta Biomater., 2010, 6, 229–240 CrossRef CAS PubMed
.
- G. Lusvardi, G. Malavasi, L. Menabue and S. Shruti, Gallium-containing phosphosilicate glasses: functionalization and in vitro bioactivity, Mater. Sci. Eng., C, 2013, 33, 3190–3196 CrossRef CAS PubMed
.
- Q. Z. Chen, K. Rezwan, D. Armitage, S. N. Nazhat and A. R. Boccaccini, The surface functionalization of 45S5 Bioglass®-based glass–ceramic scaffolds and its impact on bioactivity, J. Mater. Sci.: Mater. Med., 2006, 17, 979–987 CrossRef CAS PubMed
.
- Q. Z. Chen, I. Ahmed, J. C. Knowles, S. N. Nazhat, A. R. Boccaccini and K. Rezwan, Collagen release kinetics of surface functionalized 45S5 Bioglass®-based porous scaffolds, J. Biomed. Mater. Res., Part A, 2008, 86, 987–995 CrossRef CAS PubMed
.
- C. Gruian, E. Vanea, S. Simon and V. Simon, FTIR and XPS studies of protein adsorption onto functionalized bioactive glass, Biochim. Biophys. Acta, 2012, 1824, 873–881 CrossRef CAS PubMed
.
- V. Ainar, G. Malavasi, C. Magistris, G. Cerrato, G. Martra, G. Viscardi, L. Menabue and G. Lusvardi, Conjugation of amino-bioactive glasses with 5-aminofluorescein as probe molecule for the development of pH sensitive stimuli-responsive biomaterials, J. Mater. Sci.: Mater. Med., 2014, 25, 2243–2253 CrossRef PubMed
.
- J. Sun, Y. Li, L. Li, W. Zhao, L. Li, J. Gao, M. Ruan and J. Shi, Functionalization and bioactivity in vitro of mesoporous bioactive glasses, J. Non-Cryst. Solids, 2008, 354, 3799–3805 CrossRef CAS
.
- X. Zhang, D. Zeng, N. Li, J. Wen, X. Jiang, C. Liu and Y. Li, Functionalized mesoporous bioactive glass scaffolds for enhanced bone tissue regeneration, Sci. Rep., 2016, 6, 19361 CrossRef CAS PubMed
.
- S. K. Misra, D. Mohn, T. J. Brunner, W. J. Stark, S. E. Philip, I. Roy, V. Salih, J. C. Knowles and A. R. Boccaccini, Comparison of nanoscale and microscale bioactive glass on the properties of P(3HB)/Bioglass® composites, Biomaterials, 2008, 25, 1750–1761 CrossRef PubMed
.
- V. Maquet, A. R. Boccaccini, L. Pravata, I. Notingher and R. Jerome, Porous poly(α-hydroxyacid)/Bioglass® composite scaffolds for bone tissue engineering. I: preparation and in vitro characterization, Biomaterials, 2004, 25, 4185–4194 CrossRef CAS PubMed
.
- G. Jiang, M. E. Evans, I. A. Jones, C. D. Rudd, C. A. Scotchford and G. S. Walker, Preparation of poly(ε-caprolactone)/continuous bioglass fibre composite using monomer transfer moulding for bone implant, Biomaterials, 2005, 26, 2281–2288 CrossRef CAS PubMed
.
- G. A. Silva, F. J. Costa, O. P. Coutinho, S. Radin, P. Ducheyne and R. L. Reis, Synthesis and evaluation of novel bioactive composite starch/bioactive glass microparticles, J. Biomed. Mater. Res., Part A, 2004, 70, 442–449 CrossRef CAS PubMed
.
- J. A. Roether, A. R. Boccaccini, L. L. Hench, V. Maquet, S. Gautier and R. Jerome, Development and in vitro characterisation of novel bioresorbable and bioactive composite materials based on polylactide foams and Bioglass® for tissue engineering applications, Biomaterials, 2002, 23, 3871–3878 CrossRef CAS PubMed
.
- Z. Zhou, L. Liu, Q. Liu, Q. Yi, W. Zeng and Y. Zhao, Effect of surface modification of bioactive glass on properties of poly-L-lactide composite materials, J. Macromol. Sci., Part B: Phys., 2012, 51, 1637–1646 CrossRef CAS
.
- R. E. Ducker, M. T. Montague and G. J. Leggett, A comparative investigation of methods for protein immobilization on self-assembled monolayers using glutaraldehyde, carbodiimide, and anhydride reagents, Biointerphases, 2008, 3, 59–65 CrossRef CAS PubMed
.
- C. Gruian, A. Vulpoi, H. J. Steinhoff and S. Simon, Structural changes of methemoglobin after adsorption on bioactive glass, as a function of surface functionalization and salt concentration, J. Mol. Struct., 2012, 1015, 20–26 CrossRef CAS
.
- C. Gruian, A. Vulpoi, E. Vanea, B. Oprea, H. J. Steinhoff and S. Simon, The attachment affinity of hemoglobin toward silver-containing bioactive glass functionalized with glutaraldehyde, J. Phys. Chem. B, 2013, 117, 16558–16564 CrossRef CAS PubMed
.
- K. Wang, C. Zhou, Y. Hong and X. Zhang, A review of protein adsorption on bioceramics, Interface Focus, 2012, 2, 259–277 CrossRef PubMed
.
- Q. Long, Z. Da-Li, X. Zhang and Z. Jia-Bei, Surface modification of apatite–wollastonite glass ceramic by synthetic coupling agent, Front. Mater. Sci., 2014, 8, 157–164 CrossRef
.
- V. Aina, T. Marchis, E. Laurenti, E. Diana, G. Lusvardi, G. Malavasi, L. Menabue, G. Cerrato and C. Morterra, Functionalization of sol gel bioactive glasses carrying Au nanoparticles: selective Au affinity for amino and thiol ligand groups, Langmuir, 2010, 26, 18600–18605 CrossRef CAS PubMed
.
- I. B. Leonor, C. M. Alves, H. S. Azevedo and R. L. Reis, Effects of protein incorporation on calcium phosphate coating, Mater. Sci. Eng., C, 2009, 29, 913–918 CrossRef CAS
.
- M. Zhu, J. Zhang, C. Tao, X. He and Y. Zhu, Design of mesoporous bioactive glass/hydroxyapatite composites for controllable co-delivery of chemotherapeutic drugs and proteins, Mater. Lett., 2014, 115, 194–197 CrossRef CAS
.
- K. Schickle, K. Zurlinden, C. Bergmann, M. Lindner, A. Kirsten, M. Laub, R. Telle, H. Jennissen and H. Fischer, Synthesis of novel tricalcium phosphate–bioactive glass composite and functionalization with rhBMP-2, J. Mater. Sci.: Mater. Med., 2011, 22, 763 CrossRef CAS PubMed
.
- S. Hattar, A. Asselin, D. Greenspan, M. Oboeuf, A. Berdal and J. M. Sautier, Potential of biomimetic surfaces to promote in vitro osteoblast-like cell differentiation, Biomaterials, 2005, 26, 839–848 CrossRef CAS PubMed
.
- R. F. S. Lenza, J. R. Jones, W. L. Vasconcelos and L. L. Hench,
In vitro release kinetics of proteins from bioactive foams, J. Biomed. Mater. Res., Part A, 2003, 67, 121–129 CrossRef CAS PubMed
.
- W. Xia and J. Chang, Preparation, in vitro bioactivity and drug release property of well-ordered mesoporous 58S bioactive glass, J. Non-Cryst. Solids, 2008, 354, 1338–1341 CrossRef CAS
.
- W. Xia and J. Chang, Well-ordered mesoporous bioactive glasses (MBG): a promising bioactive drug delivery system, J. Controlled Release, 2006, 110, 522–530 CrossRef CAS PubMed
.
- L. Zhao, X. Yan, L. Zhou, H. Wang, J. Tang and C. Yu, Mesoporous bioactive glasses for controlled drug release, Microporous Mesoporous Mater., 2008, 109, 210–215 CrossRef CAS
.
- F. Baino, S. Fiorilli, R. Mortera, B. Onida, E. Saino, L. Visai, E. Verne and C. Vitale-Brovarone, Mesoporous bioactive glass as a multifunctional system for bone regeneration and controlled drug release, J. Appl. Biomater. Funct. Mater., 2012, 10, 12–21 Search PubMed
.
- E. Verne, M. Miola, S. Ferraris, C. L. Bianchi, A. Naldoni, G. Maina and O. Bretcanu, Surface activation of a ferrimagnetic glass-ceramic for antineoplastic drugs grafting, Adv. Biomater., 2010, 12, B309–B319 Search PubMed
.
- E. Boanini, S. Panseri, F. Arroyo, M. Montesi, K. Rubini, A. Tampieri, C. Covarrubias and A. Bigi, Alendronate functionalized mesoporous bioactive glass nanospheres, Materials, 2016, 9, 135 CrossRef PubMed
.
- G. Malavasi, E. Ferrari, L. Gigliola, A. Valentina, F. Francesca, M. Claudio, P. Francesca, S. Monica and M. Ledi, The role of coordination chemistry in the development of innovative gallium-based bioceramics: the case of curcumin, J. Mater. Chem., 2011, 21, 5027–5037 RSC
.
- X. Zhang, S. Ferraris, E. Prenesti and E. Verne, Surface functionalization of bioactive glasses with natural molecules of biological significance, Part I: gallic acid as model molecule, Appl. Surf. Sci., 2013, 287, 329–340 CrossRef CAS
.
- S. Ferraris, X. Zhang, E. Prenesti, I. Corazzari, F. Turci, M. Tomatis and E. Verne, Gallic acid grafting to a ferrimagnetic bioactive glass-ceramic, J. Non-Cryst. Solids, 2016, 432, 167–175 CrossRef CAS
.
- S. Zhao, J. Zhang, M. Zhu, Y. Zhang, Z. Liu, Y. Ma, Y. Zhu and C. Zhang, Effects of functional groups on the structure, physicochemical and biological properties of mesoporous bioactive glass scaffolds, J. Mater. Chem. B, 2015, 3, 1612–1623 RSC
.
- N. Olmo, A. I. Marti, A. J. Salinas, J. Turnay, M. Vallet-Regi and M. A. Lizarbe, Bioactive sol–gel glasses with and without a hydroxycarbonate apatite layer as substrates for osteoblast cell adhesion and proliferation, Biomaterials, 2003, 24, 3383–3393 CrossRef CAS PubMed
.
- J. A. Sanz-Herrera and A. R. Boccaccini, Modelling bioactivity and degradation of bioactive glass based tissue engineering scaffolds, Int. J. Solids Struct., 2011, 48, 257–268 CrossRef CAS
.
- C. Wu, Y. Zhang, Y. Zhu, T. Friis and Y. Xiao, Structure–property relationships of silk-modified mesoporous bioglass scaffolds, Biomaterials, 2010, 31, 3429–3438 CrossRef CAS PubMed
.
- A. R. Amini, J. S. Wallace and S. P. Nukavarapu, Short-term and long-term effects of orthopedic biodegradable implants, J. Long-Term Eff. Med. Implants, 2011, 21, 93–122 CrossRef CAS PubMed
.
- S. Solgi, M. Khakbiz, M. Shahrezaee, A. Zamanian, M. Tahriri, S. Keshtkari, M. Raz, K. Khoshroo, S. Moghadas and A. Rajabnejad, Synthesis, characterization and in vitro biological evaluation of sol–gel derived Sr-containing nano bioactive glass, Silicon, 2017, 9, 535–542 CrossRef CAS
.
- E. M. Valliant, F. Romer, D. Wang, D. S. McPhail, M. E. Smith, J. V. Hanna and J. R. Jones, Bioactivity in silica/poly(γ-glutamic acid) sol–gel hybrids through calcium chelation, Acta Biomater., 2013, 9, 7662–7671 CrossRef CAS PubMed
.
- P. Sepulveda, J. R. Jones and L. L. Hench,
In vitro dissolution of melt-derived 45S5 and sol–gel derived 58S bioactive glasses, J. Biomed. Mater. Res., 2002, 61, 301–311 CrossRef CAS PubMed
.
- L. Varila, S. Fagerlund, T. Lehtonen, J. Tuominen and L. Hupa, Surface reactions of bioactive glasses in buffered solutions, J. Eur. Ceram. Soc., 2012, 32, 2757–2763 CrossRef CAS
.
- H. R. Fernandes, A. Gaddam, A. Rebelo, D. Brazete, G. E. Stan and J. M. F. Ferreira, Bioactive glasses and glass ceramics for healthcare applications in bone regeneration and tissue engineering, Materials, 2018, 11, 2530 CrossRef CAS PubMed
.
- T. Kokubo and H. Takadama, How useful is SBF in predicting in vivo bone bioactivity?, Biomaterials, 2006, 27, 2907–2915 CrossRef CAS PubMed
.
- G. M. Luz and J. F. Mano, Preparation and characterization of bioactive glass nanoparticles prepared by sol–gel for biomedical applications, Nanotechnology, 2011, 22, 494014–494025 CrossRef PubMed
.
- C. Turdean-Ionescu, B. Stevensson, I. Izquierdo-Barba, A. Garcia, D. Arcos, M. Vallet-Regi and M. Eden, Surface reactions of mesoporous bioactive glasses monitored by solid state NMR: concentration effects in simulated body fluid, J. Phys. Chem. C, 2016, 120, 4961–4974 CrossRef CAS
.
- M. Vallet-Regi, A. J. Salinas, A. Martinez, I. Izquierdo-Barba and J. Perez-Pariente, Textural properties of CaO–SiO2 glasses for use in implants, Solid State Ionics, 2004, 172, 441–444 CrossRef CAS
.
- A. A. R. De Oliveira, A. A. De Souza, L. L. S. Dias, S. M. De Carvalho, H. S. Mansur and M. D. M. Pereira, Synthesis, characterization and cytocompatibility of spherical bioactive glass nanoparticles for potential hard tissue engineering applications, Biomed. Mater., 2011, 8, 025011 CrossRef PubMed
.
- T. Kokubo, Design of bioactive bone substitutes based on biomineralization process, Mater. Sci. Eng., C, 2005, 25, 97–104 CrossRef
.
- C. Vitale-Brovarone, F. Baino, F. Tallia, C. Gervasio and E. Verne, Bioactive glass-derived trabecular coating: a smart solution for enhancing osteointegration of prosthetic elements, J. Mater. Sci.: Mater. Med., 2012, 23, 2369–2380 CrossRef CAS PubMed
.
- A. D. Lopez-Noriego, D. Arcos, I. Izquierdo-Barba, Y. Sakamoto, O. Terasaki and M. Vallet-Regi, Ordered mesoporous bioactive glasses for bone tissue regeneration, Chem. Mater., 2006, 18, 3137–3144 CrossRef
.
-
M. Vallet-Regi, M. M. Garcia and M. Colilla, Biocompatible and bioactive mesoporous ceramics, in Biomedical applications of mesoporous ceramics: drug delivery, smart materials and bone tissue engineering, CRC Press, Boca Raton, FL, USA, 2012, p. 40 Search PubMed
.
-
A. J. Salinas, M. Vallet-Regi and J. Heikkila, Use of bioactive glasses as bone substitutes in orthopedics and traumatology, in Bioactive glasses: materials, properties and applications, ed. H. Ylanen, 2018, pp. 337–364 Search PubMed
.
- M. M. Pereira and L. L. Hench, Mechanisms of hydroxyapatite formation on porous gel-silica substrates, J. Sol-Gel Sci. Technol., 1996, 7, 59–68 CrossRef CAS
.
- I. Cacciotti, M. Lombardi, A. Bianco, A. Ravaglioli and L. Montanaro, Sol–gel derived 45S5 bioglass: synthesis, microstructural evolution and thermal behaviour, J. Mater. Sci.: Mater. Med., 2012, 23, 1849–1866 CrossRef CAS PubMed
.
- B. C. Bunker, Molecular mechanisms for corrosion of silica and silicate glasses, J. Non-Cryst. Solids, 1994, 179, 300–308 CrossRef CAS
.
- B. C. Bunker, G. W. Arnold and J. A. Wilder, Phosphate glass dissolution in aqueous solutions, J. Non-Cryst. Solids, 1984, 64, 291–316 CrossRef CAS
.
- J. L. Paris, M. Colilla, I. Izquierdo-Barba, M. Manzano and M. Vallet-Regi, Tuning mesoporous silica dissolution in physiological environment: a review, J. Mater. Sci., 2017, 52, 8761–8771 CrossRef CAS
.
- M. Etou, Y. Tsuji, K. Somiya, Y. Okaue and T. Yokoyama, The dissolution of amorphous silica in the presence of tropolone under acidic conditions, Clays Clay Miner., 2014, 62, 235–242 CrossRef CAS
.
- M. Uo, M. Mizuno, Y. Kuboki, A. Makishima and F. Watari, Properties and cytotoxicity of water soluble Na2O–CaO–P2O5 glass, Biomaterials, 1998, 19, 2277–2284 CrossRef CAS PubMed
.
- T. Woignier and J. Phalippou, Mechanical strength of silica aerogels, J. Non-Cryst. Solids, 1988, 100, 404–408 CrossRef CAS
.
- S. Calas, F. Despetis, T. Woignier and J. Phalippou, Mechanical strength evaluation from aerogel to silica glass, J. Porous Mater., 1997, 4, 211–217 CrossRef CAS
.
- F. Baino and C. Vitale-Brovarone, Three-dimensional glass-derived scaffolds for bone tissue engineering: current trends and forecasts for the future, J. Biomed. Mater. Res., Part A, 2011, 97, 514–535 CrossRef PubMed
.
-
L. C. Klein, Sol–gel optics: processing and applications, Springer Science and Business Media, New York, 1994 Search PubMed
.
- H. C. Li, D. G. Wang, J. H. Hu and C. Z. Chen, Effect of various additives on microstructure, mechanical properties and invitro bioactivity of sodium oxide–calcium oxide–silica–phosphorous pentoxide glass ceramics, J. Colloid Interface Sci., 2013, 405, 296–304 CrossRef CAS PubMed
.
- R. A. Martin, R. M. Moss, N. J. Lakar, J. C. Knowles, G. J. Cuello, M. E. Smith, J. V. Hanna and R. J. Newport, Structural characterization of titanium doped bioglass using isotopic substitution neutron diffraction, Phys. Chem. Chem. Phys., 2012, 14, 15807–15815 RSC
.
- S. M. Rabie, R. Ravarian, M. Mehmanchi, P. Khoshakhlagh and M. Azizian, Effect of alumina on microstructure and compressive strength of a porous silicate hydroxyapatite, J. Appl. Biomater. Funct. Mater., 2012, 12, 102–106 Search PubMed
.
- S. K. Arepalli, H. Tripathi, V. K. Vyas, S. Jain, S. K. Suman, R. Pyare and S. P. Singh, Influence of barium substitution on bioactivity, thermal and physio-mechanical properties of bioactive glass, Mater. Sci. Eng., C, 2015, 49, 549–559 CrossRef CAS PubMed
.
- O. Castano, N. Sachot, E. Xuriguera, E. Engel, J. A. Planell, J. H. Park, G. Z. Jin, T. H. Kim, J. H. Kim and H. W. Kim, Angiogenesis in bone regeneration: tailored calcium release in hybrid fibrous scaffolds, ACS Appl. Mater. Interfaces, 2014, 6, 7512–7522 CrossRef CAS PubMed
.
- X. Yang, L. Zhang, X. Chen, X. Sun, G. Yang, X. GuO, H. Yang, C. Gao and Z. GuO, Incorporation of B2O3 in CaO–SiO2–P2O5 bioactive glass system for improving strength of low temperature co-fired porous glass ceramics, J. Non-Cryst. Solids, 2012, 358, 1171–1179 CrossRef CAS
.
- B. A. E. Ben Arfa, S. Neto, I. M. M. Salvado, R. C. Pullar and J. M. F. Ferreira, Robocasting of Cu2+ & La3+ doped sol–gel glass scaffolds with greatly enhanced mechanical properties: compressive strength up to 14 MPa, Acta Biomater., 2019, 87, 265–272 CrossRef CAS PubMed
.
- D. R. Carter, G. H. Schwab and D. M. Spengler, Tensile fracture of cancellous bone, Acta Orthop. Scand., 1980, 51, 733–741 CrossRef CAS PubMed
.
- C. C. Lin, S. F. Chen, K. S. Leung and P. Shen, Effects of CaO/P2O5 ratio on the structure and elastic properties of SiO2–CaO–Na2O–P2O5 bioglass, J. Mater. Sci.: Mater. Med., 2012, 23, 245–258 CrossRef CAS PubMed
.
- G. Poologasundarampillai, P. D. Lee, C. Lam, A. M. Kourkouta and J. R. Jones, Compressive strength of bioactive sol–gel glass foam scaffolds, Int. J. Appl. Glass Sci., 2016, 7, 229–237 CrossRef CAS
.
- Q. Chen and G. A. Thouas, Fabrication and characterization of sol–gel derived 45S5 bioglass ceramic scaffolds, Acta Biomater., 2011, 7, 3616–3626 CrossRef CAS PubMed
.
- J. R. Jones, L. M. Ehrenfried and L. L. Hench, Optimising bioactive glass scaffolds for bone tissue engineering, Biomaterials, 2006, 27, 964–973 CrossRef CAS PubMed
.
- M. Vallet-Regi, J. Roman, S. Padilla, J. C. Doadrio and F. J. Gil, Bioactivity and mechanical properties of SiO2–CaO–P2O5 glass ceramics, J. Mater. Chem., 2005, 15, 1353–1359 RSC
.
- N. Li, C. Wang, S. Zhu, Q. Li and R. Wang, Preparation and evaluation of macroporous sol–gel bioglass with high mechanical strength, Key Eng. Mater., 2005, 280–283, 1585–1588 CAS
.
- J. M. Karp, P. D. Dalton and M. S. Shoichet, Scaffolds for tissue engineering, MRS Bull., 2003, 28, 301–306 CrossRef CAS
.
- A. R. Boccaccini, J. J. Blaker, V. Maquet, R. M. Day and R. Jerome, Preparation and characterisation of poly(lactide-co-glycolide) (PLGA) and PLGA/Bioglass® composite tubular foam scaffolds for tissue engineering applications, Mater. Sci. Eng., C, 2005, 25, 23–31 CrossRef
.
- K. Zhang, Y. Wang, M. A. Hillmyer and L. F. Francis, Processing and properties of porous poly(L-lactide)/bioactive glass composites, Biomaterials, 2004, 25, 2489–2500 CrossRef CAS PubMed
.
- G. Poologasundarampillai, B. Yu, O. Tsigkou, E. Valliant, S. Yue, P. D. Lee, R. W. Hamilton, M. M. Stevens, T. Kasuga and J. R. Jones, Bioactive silica–poly(γ-glutamic acid) hybrids for bone regeneration: effect of covalent coupling on dissolution and mechanical properties and fabrication of porous scaffolds, Soft Matter, 2012, 8, 4822–4832 RSC
.
- L. L. Hench and J. K. West, Biological applications of bioactive glasses, Life Chem. Rep., 1996, 13, 187–241 CAS
.
- A. Li, H. Shen, H. Ren, D. Wu, R. A. Martin and D. Qiu, Bioactive organic/inorganic hybrids with improved mechanical performance, J. Mater. Chem. B, 2015, 3, 1379–1390 RSC
.
- C. Bossard, H. Granel, Y. Wittrant, E. Jallot, J. Lao, C. Vial and H. Tiainen, Polycaprolactone/bioactive glass hybrid scaffolds for bone regeneration, Biomed. Glas., 2018, 4(1), 108–122 Search PubMed
.
- L. J. Gibson, Biomechanics of cellular solids, J. Biomech., 2005, 38, 377–399 CrossRef PubMed
.
- D. L. Kopperdahl and T. M. Keaveny, Yield strain behavior of trabecular bone, J. Biomech., 1998, 31, 601–608 CrossRef CAS PubMed
.
- I. Engelberg and J. Kohn, Physico-mechanical properties of degradable polymers used in medical applications: a comparative study, Biomaterials, 1991, 12, 292–304 CrossRef CAS PubMed
.
- K. Vandel de Velde and P. Kiekens, Biopolymers: overview of several properties and consequences on their applications, Polym. Test., 2002, 21, 433–442 CrossRef
.
-
J. D. Currey, Ontogenetic changes in compact bone material properties, in Bone mechanics handbook, ed. S. C. Cowin, Boca Raton, FL, Informa Healthcare, CRC Press, 2001, ch. 19, pp. 5–9 Search PubMed
.
-
X. E. Guo, Mechanical properties of cortical bone and cancellous bone tissue, in Bone mechanics handbook, ed. S. C. Cowin, Informa Healthcare, CRC Press, Boca Raton, FL, 2001, ch. 10, pp. 4–11 Search PubMed
.
- J. J. Chung, S. Li, M. M. Stevens, T. K. Georgiou and J. R. Jones, Tailoring mechanical properties of sol–gel hybrids for bone regeneration through polymer structure, Chem. Mater., 2016, 28, 6127–6135 CrossRef CAS
.
- J. E. Babensee, L. V. McIntire and A. G. Mikos, Growth factor delivery for tissue engineering, Pharm. Res., 2000, 17, 497–504 CrossRef CAS PubMed
.
- M. J. Mahoney and W. M. Saltzman, Transplantation of brain cells assembled around a programmable synthetic microenvironment, Nat. Biotechnol., 2001, 19, 934–939 CrossRef CAS PubMed
.
- S. Pina, V. P. Ribeiro, C. F. Marques, F. R. Maia, T. H. Silva, R. L. Reis and J. M. Oliveira, Scaffolding strategies for tissue engineering and regenerative medicine applications, Materials, 2019, 12, 1824 CrossRef CAS PubMed
.
- S. Yang, K. F. Leong, Z. Du and C. K. Chua, The design of scaffolds for use in tissue engineering. Part I. Traditional factors, Tissue Eng., 2001, 7, 679–689 CrossRef CAS PubMed
.
- D. W. Hutmacher, Scaffold design and fabrication technologies for engineering tissues – state of the art and future perspectives, J. Biomater. Sci., Polym. Ed., 2001, 12, 107–124 CrossRef CAS PubMed
.
- V. Miguez-Pacheco, L. L. Hench and A. R. Boccaccini, Bioactive glass beyond bone and teeth: emerging applications in contact
with soft tissues, Acta Biomater., 2015, 13, 1–15 CrossRef CAS PubMed
.
-
Handbook of bioactive ceramics, ed. T. Yamamuro, L. L. Hench and J. Wilson, CRC Press, Boca Raton, FL, 1990, vol. 1 and 2 Search PubMed
.
- M. N. Rahaman, R. F. Brown, B. S. Bal and D. E. Day, Bioactive glasses for nonbearing applications in total joint replacement, Semin. Arthroplasty, 2007, 17, 102–112 CrossRef
.
- S. Kargozar, S. Hamsehlou and F. Baino, Can bioactive glasses be useful to accelerate the healing of epithelial tissues?, Mater. Sci. Eng., C, 2019, 97, 1009–1020 CrossRef CAS PubMed
.
- T. Albrektsson and C. Johansson, Osteoinduction, osteoconduction and osteointegration, Eur. Spine J., 2001, 10, S96–S101 CrossRef PubMed
.
- V. Karageorgiou and D. Kaplan, Porosity of 3D biomaterial scaffolds and osteogenesis, Biomaterials, 2005, 26, 5474–5491 CrossRef CAS PubMed
.
- K. Anselme, P. Davidson, A. M. Popa, M. Giazzon, M. Liley and L. Ploux, The interaction of cells and bacteria with surfaces structured at the nanometre scale, Acta Biomater., 2010, 6, 3824–3846 CrossRef CAS PubMed
.
- S. Wang, T. J. Kowal, M. K. Marei, M. M. Falk and H. Jain, Nanoporosity significantly enhances the biological performance of engineered glass tissue scaffolds, Tissue Eng., Part A, 2013, 9, 1632–1640 CrossRef PubMed
.
- D. W. Hutmacher, Scaffolds in tissue engineering bone and cartilage, Biomaterials, 2000, 21, 2529–2543 CrossRef CAS PubMed
.
-
J. R. Jones and A. R. Boccaccini, Cellular ceramics in biomedical applications: tissue engineering, in Cellular ceramics: structure, manufacturing, processing and applications, ed. M. Scheffler and P. Colombo, Wiley-VCH Verlag GmbH & Co., Wenheim, 2005, pp. 550–573 Search PubMed
.
- R. G. Ribbas, V. M. Schatkoksi, T. L. D. A. Montanheiro, B. R. C. de Menezes, C. Stegemann, D. M. G. Leite and G. P. Thim, Current advances in bone tissue engineering concerning ceramic and bioglass scaffolds: a review, Ceram. Int., 2019, 45, 21051–21061 CrossRef
.
- L. G. Griffith, Polymeric biomaterials, Acta Mater., 2000, 48, 263–277 CrossRef CAS
.
- K. Y. Lee and D. J. Mooney, Hydrogels for tissue engineering, Chem. Rev., 2001, 101, 1869–1880 CrossRef CAS PubMed
.
- B. D. Porter, J. B. Oldham, S. L. He, M. E. Zobitz, R. G. Payne, K. N. An, B. L. Currier, A. G. Mikos and M. J. Yaszemski, Mechanical properties of a biodegradable bone regeneration scaffold, J. Biomech. Eng., 2000, 122, 286–288 CrossRef CAS PubMed
.
- H. Yu, H. W. Mathew, P. H. Wooley and S. Y. Yang, Effect of porosity and pore size on microstructures and mechanical properties of poly-ε-caprolactone–hydroxyapatite composites, J. Biomed. Mater. Res., Part B, 2008, 86, 541–547 CrossRef PubMed
.
- R. C. Thomson, M. J. Yaszemski, J. M. Powers and A. G. Mikos, Hydroxyapatite fiber reinforced poly(α-hydroxy ester) foams for bone regeneration, Biomaterials, 1998, 19, 1935–1943 CrossRef CAS PubMed
.
- S. S. Kim, K. M. Ahn, M. S. Park, J. H. Lee, C. Y. Choi and B. S. Kim, A poly(lactide-co-glycolide)/hydroxyapatite composite scaffold with enhanced osteoconductivity, J. Biomed. Mater. Res., Part A, 2007, 80, 206–215 CrossRef PubMed
.
- Q. Chen, N. Miyata, T. Kokubo and T. Nakamura, Bioactivity and mechanical properties of PDMS-modified CaO–SiO2–TiO2 hybrids prepared by sol–gel process, J. Biomed. Mater. Res., 2000, 51, 605–611 CrossRef CAS PubMed
.
- H. H. Lu, S. F. El-Amin, K. D. Scott and C. T. Laurencrin, Three-dimensional, bioactive, biodegradable, polymer–bioactive glass composite scaffolds with improved mechanical properties support collagen synthesis and mineralization of human osteoblast-like cells in vitro, J. Biomed. Mater. Res., Part A, 2003, 64, 465–474 CrossRef PubMed
.
- F. Baino, S. Fiorilli and C. Vitale-Brovone, Bioactive glass based materials with hierarchical porosity for medical applications: review of recent advances, Acta Biomater., 2016, 42, 18–32 CrossRef CAS PubMed
.
- O. M. Goudourni, C. Vogel, A. Grunewald, R. Detsch, E. Kontonasaki and A. R. Boccaccini, Sol–gel processing of novel bioactive Mg-containing silicate scaffolds for alveolar bone regeneration, J. Biomater. Appl., 2016, 30, 740–749 CrossRef PubMed
.
- C. M. Murphy and F. J. Obrien, Understanding the effect of mean pore size on cell activity in collagen–glycosaminoglycan scaffolds, Cell Adhes. Migr., 2010, 4, 377–381 CrossRef PubMed
.
- F. J. Obrien, B. A. Harley, M. A. Waller, I. V. Yannas, L. J. Gibson and P. J. Prendergast, The effect of pore size on permeability and cell attachment in collagen scaffolds for tissue engineering, Technol. Health Care, 2007, 15, 3–17 Search PubMed
.
- I. V. Yannas, Tissue regeneration by use of collagen–glycosaminoglycan copolymers, Clin. Mater., 1992, 9, 179–187 CrossRef CAS PubMed
.
- I. Izquierdo-Barba, D. Arcos, Y. Sakamoto, O. Terasaki, A. Lopez-Noriega and M. Vallet-Regi, High-performance mesoporous bioceramics mimicking bone mineralization, Chem. Mater., 2008, 20, 3191–3198 CrossRef CAS
.
- A. J. Salgado, O. P. Coutinho and R. L. Reis, Bone tissue engineering: state of the art and future trends, Macromol. Biosci., 2004, 4, 743–765 CrossRef CAS PubMed
.
- A. J. Salinas and M. Vallet-Regi, Glasses in bone regeneration: a multiscale issue, J. Non-Cryst. Solids, 2016, 432, 9–14 CrossRef CAS
.
- C. Wu, Y. Zhou, J. Chang and Y. Xiao, Delivery of dimethyloxallyl glycine in mesoporous bioactive glass scaffolds to improve angiogenesis and osteogenesis of human bone marrow stromal cells, Acta Biomater., 2013, 9, 9159–9168 CrossRef CAS PubMed
.
- H. Yun, S. E. Kim, Y. T. Hyun, S. J. Heo and J. W. Shin, Hierarchically mesoporous–macroporous bioactive glasses scaffolds for bone tissue regeneration, J. Biomed. Mater. Res., Part B, 2008, 87, 374–380 CrossRef PubMed
.
- Y. Zhu, C. Wu, Y. Ramaswamy, E. Kockrick, P. Simon, S. Kaskel and H. Zreiqat, Preparation, characterization and in vitro bioactivity of mesoporous bioactive glasses (MBGs) scaffolds for bone tissue engineering, Microporous Mesoporous Mater., 2008, 112, 494–503 CrossRef CAS
.
- X. Li, J. Shi, X. Dong, L. Zhang and H. Zeng, A mesoporous bioactive glass/polycaprolactone composite scaffold and its bioactivity behavior, J. Biomed. Mater. Res., Part A, 2008, 84, 84–91 Search PubMed
.
- C. Wu, Y. Ramaswamy, Y. Zhu, R. Zheng, R. Appleyard, A. Howard and H. Zreiqat, The effect of mesoporous bioactive glass on the physiochemical, biological and drug-release properties of poly(DL-lactide-co-glycolide) films, Biomaterials, 2009, 30, 2199–2208 CrossRef CAS PubMed
.
- Y. Zhu, Y. Zhang, C. Wu, Y. Fang, J. Yang and S. Wang, The effect of zirconium incorporation on the physiochemical and biological properties of mesoporous bioactive glasses scaffolds, Microporous Mesoporous Mater., 2011, 143, 311–319 CrossRef CAS
.
- C. Wu, R. Miron, A. Sculean, S. Kaskel, T. Doert, R. Schulze and Y. Zhang, Proliferation, differentiation and gene expression of osteoblasts in boron-containing associated with dexamethasone deliver from mesoporous bioactive glass scaffolds, Biomaterials, 2011, 32, 7068–7078 CrossRef CAS PubMed
.
- C. Wu, W. Fan, M. Gelinsky, Y. Xiao, P. Simon, R. Schulze, T. Doert, Y. Luo and G. Cuniberti, Bioactive SrO–SiO2 glass with well-ordered mesopores: characterization, physiochemistry and biological properties, Acta Biomater., 2011, 7, 1797–1806 CrossRef CAS PubMed
.
- C. Wu, W. Fan, Y. Zhu, M. Gelinsky, J. Chang, G. Cuniberti, V. Albrecht, T. Friis and Y. Xiao, Multifunctional magnetic mesoporous bioactive glass scaffolds with a hierarchical pore structure, Acta Biomater., 2011, 7, 3563–3572 CrossRef CAS PubMed
.
- C. Wu, J. Chang and Y. Xiao, Mesoporous bioactive glasses as drug delivery and bone tissue engineering platforms, Ther. Delivery, 2011, 2, 1189–1198 CrossRef CAS PubMed
.
- J. R. Jones, G. Poologasundarampillai, R. C. Atwood, D. Bernard and P. D. Lee, Non-destructive quantitative 3D analysis for the optimisation of tissue scaffolds, Biomaterials, 2007, 28, 1404–1413 CrossRef CAS PubMed
.
- R. A. Martin, S. Yue, J. V. Hanna, P. D. Lee, R. J. Newport, M. E. Smith and J. R. Jones, Characterizing the hierarchical structures of bioactive sol–gel silicate glass and hybrid scaffolds for bone regeneration, Philos. Trans. R. Soc., A, 2012, 370, 1422–1443 CrossRef CAS PubMed
.
- J. R. Jones, S. Lin and S. Yue, Bioactive glass scaffolds for bone regeneration and their hierarchical characterization, Proc. Inst. Mech. Eng., Part H, 2010, 224, 1373–1387 CrossRef CAS PubMed
.
-
J. R. Jones, Sol–gel materials for biomedical applications, in The sol–gel handbook: synthesis, characterization and application, ed. L. David and Z. Marcos., Wiley-VCH Verlag GmbH, 1st edn, 2015, pp. 1345–1369 Search PubMed
.
- J. R. Jones and L. L. Hench, Effect of surfactant concentration and composition on the structure and properties of sol–gel-derived bioactive glass foam scaffolds for tissue engineering, J. Mater. Sci., 2003, 38, 3783–3790 CrossRef CAS
.
- J. R. Jones and L. L. Hench, Factors affecting the structure and properties of bioactive foam scaffolds for tissue engineering, J. Biomed. Mater. Res., Part B, 2004, 68, 36–44 CrossRef PubMed
.
- P. Sepulveda, J. R. Jones and L. L. Hench, Bioactive sol–gel foams for tissue repair, J. Biomed. Mater. Res., 2002, 59, 340–348 CrossRef CAS PubMed
.
- J. E. Gough, J. R. Jones and L. L. Hench, Nodule formation and mineralization of human primary osteoblasts cultured on a porous bioactive glass scaffold, Biomaterials, 2004, 25, 2039–2046 CrossRef CAS PubMed
.
-
R. J. Crook, 58S sol–gel bioglass: a study of osteoproductive interfacial and handling properties using new microscopic techniques, PhD thesis, University of London, 2013
.
- P. Valerio, M. H. R. Guimaraes, M. M. Pereira, M. F. Leite and A. M. Goes, Primary osteoblast cell response to sol–gel derived bioactive glass foams, J. Mater. Sci.: Mater. Med., 2005, 16, 851–856 CrossRef CAS PubMed
.
- J. R. Jones, O. Tsigkou, E. E. Coates, M. M. Steven, J. M. Polak and L. L. Hench, Extracellular matrix formation and mineralization on a phosphate free porous bioactive glass scaffold using primary human osteoblasts (HOB) cells, Biomaterials, 2007, 28, 1653–1663 CrossRef CAS PubMed
.
|
This journal is © The Royal Society of Chemistry 2020 |