DOI:
10.1039/D0RA03786A
(Paper)
RSC Adv., 2020,
10, 23403-23409
A quick selection of natural deep eutectic solvents for the extraction of chlorogenic acid from herba artemisiae scopariae
Received
27th April 2020
, Accepted 1st June 2020
First published on 19th June 2020
Abstract
Natural deep eutectic solvents (NADES) were successfully employed as green alternatives to the traditional ones for the extraction of chlorogenic acid from herba artemisiae scopariae. Significantly, the method of solvent effect theory chemical calculation assistance to guide the NADES selection for the extraction was proposed. Proline–malic acid was successfully screened as the suitable solvent using the calculation results and it gave the best chlorogenic acid yield of 3.77 mg g−1 among the NADES tested with a solvation free energy of −5.86 × 106 kJ mol−1 from the calculation. The calculation-assisted method saves costs and material resources for the applications of the green alternative NADES and provides a research route that can be used for the extraction of target active molecules in the traditional Chinese medicine and food industry.
1 Introduction
Plants, rich in reserves, are the most important potential sources of natural bioactive compounds such as primary and secondary metabolites. Chlorogenic acid, as a vital antioxidant1 of the secondary metabolites, is widely found in artemisia compositae, eucommia and caprifoliaceae. Because of the biological activity of scavenging free radicals, antibacterial and anti-inflammatory activities, inhibiting tumors and protection from sun, it is significantly more attractive in commercial applications, particularly in cosmetics, healthcare, pharmaceutics, and food processing fields. Herba artemisiae scopariae (HAS), the dried sprout of Artemisia argyi, is one of the primary sources of chlorogenic acid and widely distributed in China as one of the well-known traditional herbs. In addition, numerous clinical applications were discovered with comprehensive pharmacological effects.
In general, organic solvents are applied in the extraction and separation of natural products in quantities, which bring countless environmental problems. To alleviate the issue, numerous related studies have been launched to discover renewable green solvents. The second generation of eco-friendly solvent-ionic liquids2,3 have attracted considerable attention owing to their special physical and chemical properties.4,5 Although ionic liquids can overcome numerous shortcomings of the conventional solvents and have shown certain advantages6–8 in some fields, such as low cytotoxicity for separating bioactive compounds,9,10 their limitations in the food and medicine industry are not negligible. Most ionic liquids have problems of difficulty in purification, high price and toxicity.11 For example, the important components of ionic liquids are not only the tetraalkylammonium, [CnPY]+, [CnMIM]+ cations, but also a large number of anionic substances, such as PF6−, BF4− and CF3SO3−, which are expensive and have relatively high toxicity and irritation.12
Therefore, deep eutectic solvents (DESs) have emerged and expanded observably as a promising substitution to the traditional organic solvents. DESs, consist of a hydrogen bond acceptor and hydrogen bond donor, a eutectic mixture that has the characteristics of simple preparation, strong dissolving ability,13 low volatilization at high temperature,14 selectivity,15 adjustable polarity. In particular, the natural deep eutectic solvents (NADESs), composed of neutral, acidic or basic compounds that are obtained from nature, have been tried to replace organic solvents in the fields of extraction and separation,16,17 electrochemistry,18 biomedical science19,20 with excellent biodegradability.21 Dai22 et al. conducted an in-depth study of NADES and pointed out its application potential as an promising green medium in the extraction and separation of targets compounds from mixtures. The ternary eutectic solvent applied in the Diels–Alder reaction made the system react faster and produce higher yields.23 Phenolic compounds were extracted from safflower by NADES green media with a higher extraction rate; also, it was discovered that the stability of phenolic compounds can be enhanced under NADES.24 Coincidentally, Karina25 proved the wonderful extraction potential of NADES in metabolites. The application of NADES for the extraction of chlorogenic acid active substances is also worth exploring.
Despite the potential, accurately selecting appropriate NADES for the project is still an unresolved issue. Wei26 experiment compared the effects of twelve different NADES in extracting phenolics from Cajanus cajan leaves. Zhuang27 picked three varieties of fixed base NADES and investigated the best option for the platycladi cacumen extraction. The extraction of rutin with NADES was carried out using thirteen common solvents.28 Density, stability and pH were considered as the basis for NADES screening to reduce the amount of experimentation.29 However, NADES were plentiful in quantity. To obtain the most suitable solvent is a huge project. The process is time-consuming and labor-intensive as well as a waste of resources. Searching for a rapid and economical selection path is critical for the extraction applications of NADES.
With the development of quantum chemistry, numerous scholars30,31 have explored the solvent effect of the solvents surrounding the solutes. The introduction of quantum chemistry provides a more economical route for solvent screening and applications. In this study, for the extraction of the active material chlorogenic acid from the herba artemisiae scopariae, computationally assisted solvent screening of NADES was considered, and not only experimentation was simplified and resources were saved, but it also greatly improved the efficiency of NADES for industrial applications.
2 Experimental
2.1. Materials
Malic acid (≥99% mass fraction purity) and L(−) proline (≥99% mass fraction purity) were purchased from MYM Biological Technology Co., Ltd (Beijing China). Choline chloride (≥98% mass fraction purity) was obtained from Sinopharm Chemical Reagent Co., Ltd. (Shanghai China). D(−) Fructose (≥99% mass fraction purity) was purchased from Shanghai Boao Biotechnology Co., Ltd. D(−) Glucose (≥99% mass fraction purity) was gained from Alfa Aesar (China) Chemicals Co., Ltd. Sucrose was purchased from Guangdong Guanghua Sci-Tech Co., Ltd. Lactic acid (≥85% mass fraction purity) and D(−) sorbitol (≥98% mass fraction purity) were obtained from Tianjin Komiou Chemical Reagent Co., Ltd. Such reagents were prepared to produce NADES as the extraction solvent. Chromatographically pure acetonitrile and phosphate acid purchased from Yonghua Chemical Technology (Jiangsu) Co., Ltd. were prepared for the mobile phase solution. The chlorogenic acid standard (≥98% mass fraction purity) was purchased from Beijing Bailingwei Technology Co., Ltd.
2.2. Preparation of raw materials
Herba artemisiae scopariae traditional Chinese herb materials were obtained from the Xinjiang province. The preliminary dusting of the raw material was performed by sorting and pulverizing on a micro-plant mill (Tianjin Taisite Instrument Co., Ltd.). The particles with a size of about 2–4 mm were gained. The treated materials were dried for 2 h in the electric blast oven (Shanghai Yiheng Scientific Instrument Co., Ltd.). All dried herb samples were stored in a seal desiccator for further use.
2.3. Preparation of NADES
Six representative types of NADES were selected from the numerous species and prepared as solvents according to a certain mole ratio:32 malic acid–choline chloride (1
:
1, MCH), fructose–glucose–sucrose (1
:
1
:
1, FGSH), lactic acid–glucose (5
:
1, LGH), glucose–choline chloride (1
:
1, GCH), proline–malic acid (1
:
1, PMH), sorbitol–choline chloride (1
:
3, SoCH). The mixtures were obtained by stirring and heating in an oil bath at 90 °C until a homogeneous transparent liquid was formed. Samples with different water contents (25%, 40%, 55%, 70%, and 85%) were prepared for extracting first and the 55% group showed a significantly better extraction effect than others. In order to reduce the viscosity for proving utilization, the NADES used in this study were prepared with a fifty-five percent water content. They were placed in the desiccator until completely cooled down.
2.4. Extraction of chlorogenic acid from herba artemisiae scopariae
The as-prepared herba artemisiae scopariae (HAS) material was extracted with NADES using a water bath. In order to prevent splashing, the dried materials were infiltrated for 5 min by adding 5 ml of water until the HAS were completely moist. 2 g of dried HAS particles were mixed with 10 mL of the as-prepared NADESs and conventional solvents (water and ethanol, respectively) in flasks, and the samples were pre-treated via a process of ultrasonic cell smashing under 300 W for 30 min. The active substances were released adequately. Then, the flasks were placed in water baths at 323 K with continuous stirring for 2 h, and the crude extract was obtained through a filter to remove the dregs. Next, the turbid extracts were centrifuged at 1400 rpm for 20 min to remove the finer impurities. The supernatant was further applied to complete the determination of the chlorogenic acid content using an Agilent 1260 high-performance liquid chromatography instrument. The parallel test repeated three times for average. The extraction rate was calculated using the formula: |
The extraction rate (mg g−1) = nCV/m
| (1) |
where C is the concentration of chlorogenic acid measured under HPLC conditions, V is the sample volume, n is the dilution factor, m is the weight of HAS.
2.5. High-performance liquid chromatography analysis
2.5.1 Preparation of standard curve. A series of concentration gradient solutions of the chlorogenic acid standard were prepared accurately. 8 mg chlorogenic acid standard was weighed accurately, and the original standard solution was obtained by adding the deionized water into the flask to dissolve the sample. 0.1 ml, 1 ml, 3 ml, 5 ml, and 7 ml of the original standard solution were pipetted into the numbered 10 ml brown volumetric flasks and then water was added to acquire the standard samples with different concentrations. The samples were stored in vials and placed in the desiccator for high-performance liquid chromatography (HPLC) analysis.
2.5.2 The condition of HPLC. The chlorogenic acid content was measured by HPLC. The samples were analyzed via HPLC-VWD on a 4.6 mm × 250 mm (5 μm, made in the USA) Agilent Eclipse plus C18 column (315 K) using a UV detector at 327 nm. The mobile phase solution was composed of acetonitrile (0.4%) and phosphoric acid solution (1
:
9, 99.6%). The system was eluted at a flow rate of 1 ml min−1 for 2 h. Under these chromatographic conditions, the chlorogenic acid retention time is around 20 min, and it was well separated from other chromatographic peaks with a resolution greater than 1.5. The number of theoretical plates is not less than 3000 based on the peak of chlorogenic acid.
2.6. Separation and purification of chlorogenic acid
The separation and purification of the chlorogenic acid crude extract were realized via the macroporous resin technology. A macroporous resin is generally applied in adsorbing hydrophobic organic substances from aqueous solutions. It has a special porous structure; a polymer specialized in separation and purification with both adsorption and screening properties. The advantages of good selectivity, high mechanical strength and recyclability have promoted macroporous resins as a popular technology for separation and purification in recent years.
NKA-9 was selected as the adsorption resin for the purification of chlorogenic acid. The resin was soaked in absolute ethanol for 48 h to ensure that it was fully swollen. Deionized water was added to rinse the resin, which was then placed in the chromatography column to recover the ethanol until there was no alcohol smell. Then, NKA-9 was soaked in a 1% wt sodium hydroxide solution for 4 h, deionized water was added until the resin was neutral, and the same process was executed in an 1 wt% dilute hydrochloric acid solution for 4 h. The resin regained neutrality with the use of deionized water.
Pre-treated resin (80 g) and chlorogenic acid crude extracts (500 ml) were mixed in a flask. The mixture was agitated at room temperature for 24 h until an adsorption equilibrium state was reached. Then, it was washed with deionized water to remove the NADES around the resin, this step was repeated three times. 1000 ml 40% ethanol solution (pH = 4) was prepared for desorbing chlorogenic acid from NKA-9. The NKA-9 adsorbed chlorogenic acid was desorbed with 300 ml of ethanol solution for three times, agitating at room temperature for 2 h, and the desorption solutions were collected. The desorption behavior occurred on the oscillator. Vacuum spinning removes most of the solution and then freezing dry 12 h to acquire the purified chlorogenic acid.
2.7. Calculation method
The development of quantum mechanics promoted scientists to use quantum mechanics methods to solve chemical problems. Most chemical processes occur in a solvent environment. Solute–solvent interactions can have a significant impact on the molecular energy, structure and properties. This effect can be effectively calculated within the framework of solvation models in many cases.33–36 Among these models, there are discontinuous models and continuous models. The electron polarization effect of molecules was not taken into consideration in the discontinuous models. The electrostatic interactions between the solutes and the solvents depend on the charge parameters of the selected force field, which has certain requirements for obtaining the physical parameters of the solvents. Since its proposal in 1981,37 the polarizable continuum model (PCM) has been constantly updated and extended and is one of the most reliable continuum solvation procedures.38 In this article, we discussed the conductor-like polarizable continuum model (CPCM). The theoretical basis of CPCM is characterizing the interaction between molecules by calculating the surface shielding charge density, setting the dielectric constant of the continuous medium (solvent) to infinity, that assuming an ideal conductor. The shielding charge was confined to the interface, making no electric field between solute and solvent. This ideal conductor hypothesis makes the CPCM model very suitable for the calculation of unconventional solvents. At present, the molecular energy and electronic structures of the solutes that dissolved in the solvents with uneven39 and anisotropy characters40 can be calculated. The optimization of the solute geometric structure can also be analyzed.41 Calculating the static42 and frequency-dependent polarizability43 and hyperpolarizability of the solution can be achieved. Yan et al. at Zhejiang University44 applied the conductor-like polarizable continuum model to predict the solubility of flavonoids in organic solvents for guiding the separation and purification of drugs, the team has made outstanding progress. The reliability of this research method has been affirmed continually.
During the extraction process of chlorogenic acid biomolecules, an interaction exists between the chlorogenic acid molecule and the extraction solvent molecule. Hydrogen bonds were formed among chlorogenic acid and NADES molecules, which made the molecules regularly arranged, forming a network system. The strong interaction makes the extraction environment at lower stable energy. The solvent effect was the result of the mutual attraction of the solute and solvent molecules, that is, the process by which solvent molecules accumulate around the solute through their interaction with the solute.
A key parameter describing the effect of the solvent is ΔGsol, the solvation free energy, which is a change in the free energy when the solute molecules were moved from the vacuum to the solvent.
|
ΔGsol = ΔGele + ΔGdisp + ΔGrep + ΔGcav
| (2) |
Δ
Gele, the electrostatic field component of solvent-free energy, Δ
Gdisp, Δ
Grep, van der Waals interaction between molecules, Δ
Gcav, the hole energy. The different items in
eqn (2) affect the solvation free energy with varying degrees due to the different combinations of solution systems and the special binding modes between solute molecules and solvent molecules in different solution systems.
45
The solvation free energy electrostatic field component is expressed in the CPCM model as:
|
 | (3) |
ρnucl is the charge density of the nucleus,
Vσ is the electrostatic potential generated by the surface charge,
Ĥ and
Ψ are the Hamiltonian and wave functions of the solute in solution, respectively,
Ĥo and
Ψo are the Hamiltonian and wave function of the solute in the gas phase, respectively.
The cavitation energy is the free energy required of the solute molecule to form a hole in a solvent molecule:46
|
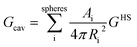 | (4) |
Ri is the radius of the cavitation,
GHS is the free energy of forming cavitation of radius
Ri in solution, and
Ai is the surface area of the cavitation in contact with the solvent molecules.
van der Waals interactions can be calculated under the classical approximations proposed by Floris and Tomasi,47 and the potential parameters of the atoms reported by Caillet and Claverie.48
Many scholars49,50 have done numerous research on the prediction of solubility, but most of them are currently stuck in simple theoretical calculations and do not combine it with the practical applications. The work guided the selection of natural deep eutectic solvents for extracting chlorogenic acid from herba artemisiae scopariae. An extraction method of a new bio-friendly solvent for active biomolecule extraction and a design and screening method for a new solvent was proposed, which has better application significance.
Gaussian 09 program software and CPCM solvation theory model were used to achieve the theoretical calculation data. All the molecules selected in the system were structurally optimized and calculated via density functional theory (DFT), B3LYP method, and 6-31++g (d, p) basis set. The optimization and frequency calculations of the structures of chlorogenic acid and different NADES solvent model molecules were accomplished with a minimum energy optimization structure that no virtual frequency appeared. For the high viscosity limit of the NADES, water was always added for reducing the viscosity when utilized. Therefore, the solvation calculation was implemented under the condition of water surrounded accompanied by the optimization of NADES composed of mixtures with different molar ratio models. Water was added as a keyword to keep water molecules evenly dispersed around NADES molecules.
The difference of the extraction ability of chlorogenic acid was exhibited when choosing various NADES in the experiment by referring to the solvation free energy ΔGsol that is considered the effects of solvent effects.
3 Results and discussion
3.1. Solvation energy calculation of the CPCM model
Conductor polarization continuous medium model (CPCM),51 a method to obtain the structure and surface charge density distribution of molecules by the theory of quantum mechanics, and then directly predicting macroscopic thermodynamic properties using the statistical mechanics method. The structures of chlorogenic acid and natural deep eutectic solvent molecules were first optimized from calculations in this study. The optimized structure diagram and charge density distribution map of chlorogenic acid are shown in Fig. 1. The charge near the hydroxyl group on the chlorogenic acid molecule showed a significant difference. The hydrogen atoms (green) have enough ability to absorb electrons to promote hydrogen bond formation, and the oxygen atoms have a strong ability to provide electrons at the same time. The frequency calculation structure has no virtual frequency, and the energy value is −3.41 × 106 kJ mol−1. The optimized molecular structure of chlorogenic acid can be used as the computational structure.
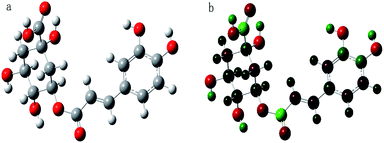 |
| Fig. 1 Structure optimization (a) and charge density distribution (b) of chlorogenic acid. | |
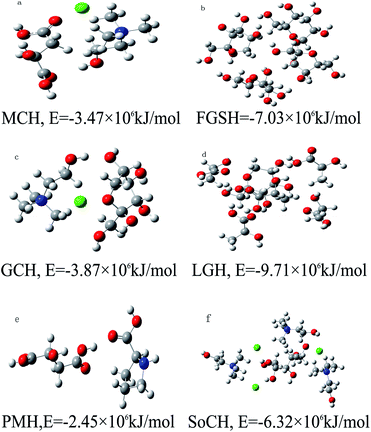 |
| Fig. 2 Structural optimization diagram of six NADES molecular models. | |
Structural optimization and frequency calculation of MCH, FGSH, LGH, GCH, PMH and SoCH models were carried out. All the structures prepared were at their lowest energy states (Fig. 2) with no virtual frequency.
The molecular structures used in the solvent effect calculation are all based on the optimized structures. Geometry optimizations used tight convergence criteria (Gaussian keywords “SCF = Tight OPT = Tight”). Frequency calculations confirmed that all optimized geometries are local minima on the potential energy surface. The solvent effects of chlorogenic acid bioactive molecules in six different types of NADES were calculated to predict the extraction ability of chlorogenic acid in different types of NADES.
The solvation free energy calculations of chlorogenic acid in six natural deep eutectic solvents were considered. The calculation results showed that chlorogenic acid has a maximum solvation free energy of −5.86 × 106 kJ mol−1 in the PMH solvent environment, which means that chlorogenic acid was easier to ionize in the PMH solvent environment. Among the several compared natural deep eutectic solvents, PMH showed the best extraction capacity. The extraction ability order of chlorogenic acid in several natural deep eutectic solvents is PMH > LGH > MCH > GCH > FGSH > SoCH. The extraction effect of these natural deep eutectic solvents for chlorogenic acid was greatly consistent with the experimental data in Section 2.
3.2. Experimental extraction of chlorogenic acid from herba artemisiae scopariae
3.2.1 The comparison of the extraction capacity of NADES and traditional solvent. The chlorogenic acid of herba artemisiae scopariae was extracted using water or ethanol solvents via the traditional methods. In order to investigate the feasibility of the deep eutectic solvent as the new type extraction solvent of chlorogenic acid, the extraction effects of six different NADES, water and ethanol were compared under the same experimental conditions, as mentioned above. The HPLC results are shown in the histogram (Fig. 3) below.
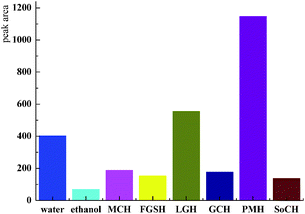 |
| Fig. 3 The comparison of the extraction capacity of NADES and traditional solvent. | |
PMH has the obvious advantages as solvents for extracting chlorogenic acid from herba artemisiae scopariae. The data showed that not all the types of NADES were effective in the extraction of chlorogenic acid. It was very critical and useful for selecting the right NADES for the extraction activity. Simultaneously, water makes a certain contribution as an extraction solvent. In the extraction experiment of NADES later, we added water to reduce the viscosity of NADES to improve its usability. The addition of moisture greatly increased the hydrogen bonding of the system while reducing the viscosity of the solvents as speculation. The formation of strong interactions increased the dynamic difference of the extraction process and enhanced the extraction effect.
3.2.2 The comparison of the extraction capacity of different NADES. The pre-treated herba artemisiae scopariae materials were extracted using MCH, FGSH, LGH, GCH, PMH, SoCH, water and ethanol. The chlorogenic acid was extracted from the pre-treated herba artemisiae scopariae materials.The chlorogenic acid standard was formulated into five different concentrations of samples and the standard curve was drawn depending on the HPLC test. Taking the concentration (μg ml−1) as the abscissa and obtained the regression equation y = 1.4445 + 0.0143x, R2 = 0.9988 (x is the peak area, and y is the concentration of chlorogenic acid). The results show that the chlorogenic acid concentration has a good linear relationship with the concentration in the range of 1.62–113.40 μg ml−1.
Under the HPLC measurement condition described above, the high-performance liquid chromatograms of the extracts achieved good separation efficiency for different components, the chlorogenic acid target was effectively acquired (Fig. 4B). The extract was prepared for high performance liquid chromatography analysis and the chromatographic peak area data is shown in Table 1. It is obvious that the PMH solvent showed the best extraction capacity for chlorogenic acid in the experimental results. The extraction concentration of chlorogenic acid was the highest when the PMH solvent was the extraction agent, reaching 16.75 μg ml−1, and the yield of 3.77 mg g−1 (1.32 mg g−1 of water, 0.11 mg g−1 of ethanol), while SoCH exhibited the least suitable extraction capacity of chlorogenic acid among these six NADES (Fig. 5). The calculated results are basically consistent with the experimental results.
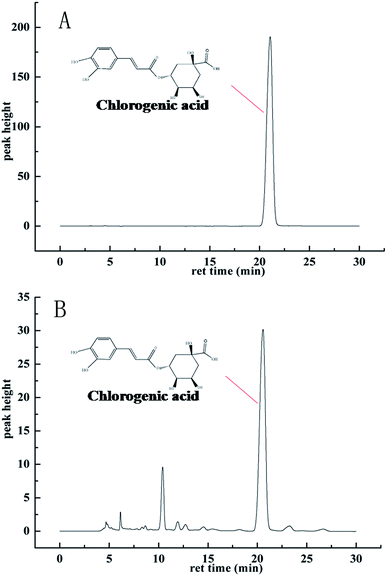 |
| Fig. 4 High-performance liquid chromatogram ((A) standard, (B) sample). | |
Table 1 Calculation and experimental data of the dissolution capacity of chlorogenic acid in six NADES
Solvents |
Optimal structural energy/kJ mol−1 × 106 |
Solvation free energy/kJ mol−1 × 106 |
Peak area |
Chlorogenic acid concentration/μg ml−1 |
Relative std deviation/% |
MCH |
−3.47 |
−6.86 |
188.79 |
2.76 |
1.89 |
FGSH |
−7.01 |
−10.42 |
153.53 |
2.25 |
4.54 |
GCH |
−3.87 |
−6.11 |
554.69 |
8.10 |
2.44 |
LGH |
−9.72 |
−9.71 |
177.44 |
2.59 |
2.63 |
PMH |
−2.45 |
−5.86 |
1146.92 |
16.75 |
0.82 |
SoCH |
−6.31 |
−11.42 |
137.25 |
2.00 |
1.58 |
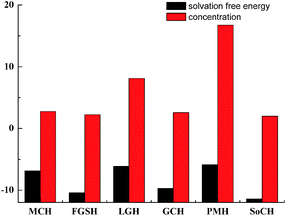 |
| Fig. 5 A comparison of the solvation free energy and extraction capacity of different NADES. | |
At present, there is a large amount of research on NADES as extraction solvents. In general, NADES was confirmed by dividing one component into choline chloride, changing the other component,52,53 or fixing one component into two or three substances, changing the other component with four to five chemicals.54 However, there are hundreds of NADES and almost more than 30 types of NADES are fixed with choline chloride. The experimental selection of the NADES solvent will be a costly project. The introduction of computational chemistry with the perspective of the solvent effect will save a huge cost for the selection of NADES. Tang researched the solvent effect method on predicting the selection of the solvent for flavonoids.44 The histogram shows more clearly the potential of PMH as a solvent for chlorogenic acid extracted from herba artemisiae scopariae with the higher solvation free energy.
4 Conclusions
Six NADES were considered by the solvent effect for the extraction of chlorogenic acid from herba artemisiae scopariae as models. PMH with the high solvation free energy has the best extraction effect. The simulation results were consistent with the extraction capacity and guided the selection of NADES for the experiment of extracting chlorogenic acid from herba artemisiae scopariae. The combination of simulation calculation and experiments was used for quickly selecting the green novel NADES for extract use. The method provided a reliable solvent selection method for the extraction and storage of biologically active substances.
Conflicts of interest
There are no conflicts to declare.
Acknowledgements
We are grateful for the financial support from the National Key Research and Development Program of China (2018YFB1501404), the Science and Technology Planning Project of Guangdong Province, China (2017A010104005).
Notes and references
- J. Zhao, Z. Zhang, J. Dai, L. Wang, C. Zhang, Y. Ye and L. Li, RSC Adv., 2014, 4, 43057–43063 RSC.
- S. Zhang, N. Sun, X. He, X. Lu and X. Zhang, J. Phys. Chem. Ref. Data, 2006, 35, 1475–1517 CrossRef CAS.
- K. R. Seddon, J. Chem. Technol. Biotechnol., 1997, 68, 351–356 CrossRef CAS.
- A. P. Abbott, G. Capper, D. L. Davies, R. K. Rasheed and V. Tambyrajah, Chem. Commun., 2003, 70–71 RSC.
- W. Tang and K. Ho Row, Bioresour. Technol., 2020, 296, 122309 CrossRef CAS PubMed.
- Y. Sun, J. Xu, F. Xu and R. Sun, Ind. Crops Prod., 2013, 47, 277–285 CrossRef CAS.
- Y. Sun, J. Xu, F. Xu and R. Sun, Process Biochem., 2013, 48, 844–852 CrossRef CAS.
- W. Tang and K. H. Row, J. Sep. Sci., 2018, 41, 3372–3381 CrossRef CAS PubMed.
- W. Jin, Q. Yang, B. Huang, Z. Bao, B. Su, Q. Ren, Y. Yang and H. Xing, Green Chem., 2016, 18, 3549 RSC.
- X. Liu, C. Ji, Q. Yang, Z. Bao, X. Fan, Y. Yang and H. Xing, ACS Sustainable Chem. Eng., 2017, 5, 1974 CrossRef CAS.
- N. V. Plechkova and K. R. Seddon, Chem. Soc. Rev., 2008, 37, 123–150 RSC.
- N. Tang, J. Zhong and W. Yan, J. Chem. Eng. Data, 2016, 61, 4203–4208 CrossRef CAS.
- H. G. Morrison, C. C. Sun and S. Neervannan, Int. J. Pharm., 2009, 378, 136–139 CrossRef CAS PubMed.
- B. Y. Zhao, P. Xu, F. Yang, H. Wu, M. Zong and W. Lou, ACS Sustainable Chem. Eng., 2015, 3, 2746–2755 CrossRef CAS.
- Y. Dai, G. J. Witkamp, R. Verpoorte and Y. H. Choi, Anal. Chem., 2013, 85, 6272–6278 CrossRef CAS PubMed.
- Y. Xie, H. Liu, L. Lin, M. Zhao, L. Zhang, Y. Zhang and Y. Wu, RSC Adv., 2019, 9, 22677–22684 RSC.
- Y. C. Wu, P. Wu, Y. B. Li, T. C. Liu, L. Zhang and Y. H. Zhou, RSC Adv., 2018, 8, 15069–15077 RSC.
- C. Mukesh, R. Gupta, D. N. Srivastava, S. K. Nataraj and K. Prasad, RSC Adv., 2016, 6, 28586–28592 RSC.
- A. Rajawat, S. Khandelwal and M. Kumar, RSC Adv., 2014, 4, 5105 RSC.
- W. Tang, Y. Dai and K. H. Row, Anal. Bioanal. Chem., 2018, 410, 7325–7336 CrossRef CAS PubMed.
- A. Paiva, R. Craveiro, I. Aroso, M. Martins and R. L. Reis, ACS Sustainable Chem. Eng., 2014, 2, 1063–1071 CrossRef CAS.
- Y. Dai, E. Rozema, R. Verpoorte and Y. H. Choi, J. Chromatogr. A, 2016, 1434, 50–56 CrossRef CAS PubMed.
- G. Imperato, E. Eibler, J. Niedermaier and B. Konig, Chem. Commun., 2005, 1170–1172 RSC.
- Y. Dai, R. Verpoorte and Y. H. Choi, Food Chem., 2014, 159, 116–121 CrossRef CAS PubMed.
- K. Fraige, R. D. Arrua, A. T. Sutton, C. S. Funari, A. J. Cavalheiro, E. F. Hilder and V. S. Bolzani, J. Sep. Sci., 2019, 42, 591–597 CrossRef CAS PubMed.
- Z. Wei, X. Qi, T. Li, M. Luo, W. Wang, Y. Zu and Y. Fu, Sep. Purif. Technol., 2015, 149, 237–244 CrossRef CAS.
- B. Zhuang, L. Dou, P. Li and E. Liu, J. Pharmaceut. Biomed., 2017, 134, 214–219 CrossRef CAS PubMed.
- Y. Huang, F. Feng, J. Jiang, Y. Qiao, T. Wu, J. Voglmeir and Z. Chen, Food Chem., 2017, 221, 1400–1405 CrossRef CAS PubMed.
- M. L. A. Fernandez, M. Espino, F. J. V. Gomez and M. F. Silva, Food Chem., 2018, 239, 671–678 CrossRef CAS PubMed.
- D. J. Tannor, B. Marten, R. Murphy, R. A. Friesner, D. Sitkoff, A. Nicholls, M. Ringnalda, W. A. Goddard and B. Honig, J. Am. Chem. Soc., 1994, 116, 11875–11882 CrossRef CAS.
- J. Tomasi and M. Persico, Chem. Rev., 1994, 94, 2027–2094 CrossRef CAS.
- Y. Dai, J. van Spronsen, G. J. Witkamp, R. Verpoorte and Y. H. Choi, Anal. Chim. Acta, 2013, 766, 61–68 CrossRef CAS PubMed.
- M. Ruiz-López, F. Bohr, M. Martins Costa and D. Rinaldi, Chem. Phys. Lett., 1994, 221, 109–116 CrossRef.
- J. Jeon and H. J. Kim, J. Solution Chem., 2001, 30, 849–860 CrossRef CAS.
- T. N. Truong and E. V. Stefanovich, Chem. Phys. Lett., 1995, 240, 253–260 CrossRef CAS.
- F. D. Angelis, A. Sgamellotti, M. Cossi, N. Rega and V. Barone, Chem. Phys. Lett., 2000, 328, 302–309 CrossRef.
- S. Miertuš, E. Scrocco and J. Tomasi, Chem. Phys., 1981, 55, 117–129 CrossRef.
- M. Cossi, N. Rega, G. Scalmani and V. Barone, J. Comput. Chem., 2003, 24, 669–681 CrossRef CAS PubMed.
- M. Cossi, B. Mennucci and J. Tomasi, Chem. Phys. Lett., 1994, 228, 165–170 CrossRef CAS.
- B. Mennucci, M. Cossi and J. Tomasi, J. Chem. Phys., 1995, 102, 6837–6845 CrossRef CAS.
- R. Cammi and J. Tomasi, J. Chem. Phys., 1994, 101, 3888–3897 CrossRef CAS.
- R. Cammi, M. Cossi, B. Mennucci and J. Tomasi, J. Mol. Struct., 1997, 436, 567–575 CrossRef.
- R. Cammi, L. Frediani, B. Mennucci and J. Tomasi, J. Mol. Struct., 2003, 633, 209–216 CrossRef CAS.
- N. Tang and W. Yan, J. Zhejiang Univ., 2017, 62, 115–128 Search PubMed.
- M. Orozco and F. J. Luque, Chem. Rev., 2000, 100, 4187–4226 CrossRef CAS PubMed.
- R. A. Pierotti, Chem. Rev., 1976, 76, 717–726 CrossRef CAS.
- F. Floris, J. Tomasi and J. P. Ahuir, J. Comput. Chem., 1991, 12, 784–791 CrossRef CAS.
- J. Caillet, P. Claverie and B. Pullman, Acta Crystallogr. B, 1978, 34, 3266–3272 CrossRef.
- A. Niederquell and M. Kuentz, J. Pharm. Sci., 2018, 107, 503–506 CrossRef CAS PubMed.
- C. M. Gray, K. Saravanan, G. Wang and J. A. Keith, Mol. Simulat., 2017, 43, 420–427 CrossRef CAS.
- M. Cossi, V. Barone, R. Cammi and J. Tomasi, Chem. Phys. Lett., 1996, 255, 327–335 CrossRef CAS.
- X. Peng, M. Duan, X. Yao, Y. Zhang, C. Zhao, Y. Zu and Y. Fu, Sep. Purif. Technol., 2016, 157, 249–257 CrossRef CAS.
- M. C. Bubalo, N. Ćurko, M. Tomašević, K. K. Ganić and I. R. Redovniković, Food Chem., 2016, 200, 159–166 CrossRef PubMed.
- T. Bosiljkov, F. Dujmić, M. C. Bubalo, J. Hribar, R. Vidrih, M. Brnčić, E. Zlatic, I. R. Redovniković and S. Jokić, Food Bioprod. Process., 2017, 102, 195–203 CrossRef CAS.
|
This journal is © The Royal Society of Chemistry 2020 |
Click here to see how this site uses Cookies. View our privacy policy here.