DOI:
10.1039/D0RA03736B
(Paper)
RSC Adv., 2020,
10, 24642-24652
CO2 and water vapor adsorption properties of framework hybrid W-ZSM-5/silicalite-1 prepared from RHA
Received
26th April 2020
, Accepted 3rd June 2020
First published on 29th June 2020
Abstract
Framework hybrid W-ZSM-5 and W-silicalite-1 zeolites were synthesized by hydrothermal methods using rice husk ash (RHA) as a silicon raw material. RHA is a low-cost precursor material, and its use can also alleviate the environmental and human health related problems that may occur when it is stacked in open fields. A series of comparative samples were characterized by XRD, FTIR, ICP-OES, SEM, N2 adsorption–desorption and pore size analysis in order to examine their crystal structure, hybrid state, morphology and textural properties. The maximum CO2 adsorption capacities of W-ZSM-5 and W-silicalite-1 are 81.69 and 69.96 cm3 g−1, respectively, measured at 15 bar. The isotherms of CO2, N2 and O2 are perfectly fitted by the Toth model, and it is noted that the presence of Al atoms increases the heterogeneity. It can be seen that the greater the heterogeneity of the adsorbent, the larger the CO2 adsorption capacity achieved. The incorporation of tungsten into the framework does not affect the crystallization of the zeolite, but it prevents the formation of silanol and O–H groups at the adsorption sites. Therefore, the CO2/H2O selectivity of W-ZSM-5 is slightly higher than that of ZSM-5, and that of W-silicalite-1 is three times that of silicalite-1. W-ZSM-5/silicalite-1 are promising adsorbents for separating CO2 under humid industrial conditions.
1. Introduction
As the main greenhouse gas, carbon dioxide (CO2) from factories is emitted into the atmosphere, contributing to the greenhouse effect and a series of problems for ecosystems, biodiversity and human economic activity.1,2 In the short term, if there is no revolutionary development of new energy and energy storage technologies, fossil fuel combustion will still be needed to meet the growing demand for energy consumption.3 A promising medium-term solution is carbon capture and utilization (CCU), which continuously captures CO2 from industrial processes and converts it into value-added chemicals.4 This technology is not enough to replace large-scale storage facilities for CO2, but other economic chemical products can compensate for the cost of carbon emission reduction. The crucial technology captures CO2 gas from power plants, industry and transportation.5 In addition, CO2 capture and separation technology is also used for air prepurification, natural gas purification and carbon removal from flue gas.6–9 Aqueous amine absorption, solid adsorbent adsorption, membrane separation and cryogenic separation methods for CO2 capture have received wide attention due to their respective advantages.10–12 Instead of the aqueous alkanolamine absorbents, the use of solid adsorbents can avoid large amounts of renewable energy and equipment corrosion. They can also be used in pressure swing adsorption and temperature swing adsorption processes to separate CO2 efficiently.13 Hence, it is necessary to explore solid adsorbents that are superior in performance, economical to produce, and suitable for practical industrial conditions.
Zeolites are pore-rich solid adsorbents with regions of electric field due to their inherently negatively charged frameworks and cations. And thus they have unique physical adsorption properties. Yan et al. prepared amidoxime modified zeolite X to simulate its sensitivity change as a cerium ion adsorbent for industrial wastewater treatment.14 Wanigarathna et al. optimized the pore size of zeolite 5A by chemical modification with tetraethyl orthosilicate to obtain pure R134a from a refrigerant mixture.15 Zukal et al. prepared isoreticular siliceous zeolites for the separation of CO2 and investigated the effect of their dimensions on the heat of adsorption.16 Industrial waste, agricultural waste and natural minerals can be used as alternative synthetic raw materials for silicon and aluminum to improve the economics of preparation and facilitate large-scale application. The agricultural waste rice husk ash (RHA) contains more than 90% amorphous silica, which is suitable for preparing silica-based adsorbents including zeolites. Setthaya et al. synthesized a TiO2–zeolite photocatalyst by a hydrothermal method with metakaolin and rice husk ash, which showed excellent performance in the adsorption and degradation of methylene blue.17 Khoshbin et al. synthesized a series of ZSM-5 zeolites using ultrasonic energy and studied their activity in the catalytic cracking of light naphtha.18 Jesudoss et al. successfully synthesized ZSM-5 by a hydrothermal method using waste such as rice hull ash, and studied the potential cytotoxic effects of different concentrations of graded ZSM-5 zeolite on human lung epithelial carcinoma A549 cells.19 RHA is a potential source of low-cost precursors, and its use can also alleviate the environmental and human health related issues that may occur when it is stacked in open spaces.20 Further, the RHA concentrated in biomass power plants has excellent sustainability performance in environmental, economic and social assessments.21
The synthesis of ZSM-5 zeolite needs a template as a structure-directing agent, so it is easier to prepare it with inexpensive alternative raw materials.22 In general, ZSM-5 zeolite with a low silicon/aluminum ratio has an excellent performance in pressure swing adsorption (PSA) applications, because the counter ions outside the framework are the main active adsorption sites for CO2 adsorption.23 Also, highly crystalline ZSM-5 with high surface area and adjustable three-dimensional pore structure is expected to become a promising CO2 adsorbent.24 Although ZSM-5 zeolite has been widely used and intensively explored in various fields, it also needs to be modified to improve the practicability for CO2 adsorption; for example, hybrid zeolites doped with other substances have been prepared. Kalantarifard et al. synthesized ZSM-5 with large pore surface structures and modified it with ethylenediamine to achieve high CO2 adsorption capacity from the gas stream.25 Lin et al. used an amine bifunctional compounding strategy for the manufacture of a graft impregnated ZSM-5/KIT-6 composite, which exhibited excellent CO2 adsorption performance and prospects for capturing CO2 from actual flue gas.26 It is well known that the CO2 adsorption performance of adsorbents is largely influenced by water vapor in various industrial applications. Datta et al. prepared a microporous coppersilicate in which copper atoms were introduced into the framework of a titanosilicate in place of titanium atoms and achieved excellent CO2 capture performance in humid flue gases and humid atmosphere.27 Ke et al. used a bulky alkali metal–crown ether complex as a template to incorporate boron and copper heteroatoms into the framework of a high silicon RHO zeolite, which was used to remove traces of carbon dioxide from a CO2/CH4/N2 mixture under humid conditions.28 Ding et al. studied a method for liquid-phase oxidation of ethylamine with hydrogen peroxide on a tungsten-doped MOR zeolite, and the catalytic reaction activity was increased due to the change in the coordination state of the W species.29 However, research on the incorporation of other atoms into the ZSM-5 framework has rarely been reported. Moreover, it is valuable to explore the effect of mixed atoms in the ZSM-5 framework on the adsorption of CO2 and water vapor.
The aim of this work is to synthesize W-ZSM-5 and W-silicalite-1, in which tungsten is incorporated within the framework, using RHA as an alternative silicon raw material to reduce the cost of preparation. The physicochemical properties of the materials were investigated by means of characterization, and a series of CO2, N2, O2 and water vapor adsorption measurements were performed. By comparing the experimental results with those for ZSM-5 and silicalite-1, the tungsten and aluminum content in the framework and its effect on gas adsorption were explored. Through calculation of the equilibrium adsorption amount, selectivity and water vapor adsorption properties, the materials were evaluated under actual humid multi-component industrial conditions.
2. Experimental
2.1. Materials
Fresh rice husk was obtained from local farms. The silica was then extracted from the RHA using alkali dissolution and acid leaching processes according to our previous work.30 Hydrochloric acid (HCl), sodium aluminate (NaAlO2), sodium tungstate dihydrate (Na2WO4·2H2O), sodium hydroxide (NaOH) and tetrapropylammonium hydroxide (TPAOH, 25 wt% in H2O) were obtained from Sinopharm Chemical Reagent Co., Ltd. The helium, nitrogen, carbon dioxide and oxygen gas (all 99.999%) used in this work were supplied by Shenyang Shuntai Special Gas.
2.2. Preparation of W-ZSM-5/silicalite-1
W-ZSM-5 zeolite was synthesized by using a hydrothermal template method with an initial gel composition of 1.24Na2O
:
100SiO2
:
Al2O3
:
2WO3
:
32TPAOH
:
2000H2O. In a typical synthesis process, 0.032 g of NaOH and 0.27 g of NaAlO2 were dissolved in 27 mL of deionized water, followed by the addition of 1.1 g of NaWO4·2H2O with continued stirring. After the stirred solution became clear, 43.4 g TPAOH and 10 g RHA silica were slowly added. Then, the solution was aged for 4 h at room temperature. The resulting mixed gel was transferred to a 200 mL Teflon-lined stainless-steel autoclave and sealed. The crystallization process was carried out in an oven at 165 °C for 48 h. The product was filtered, washed and completely dried, and finally calcined at 500 °C for 6 h in N2 flow to remove the organic template. The Al-free zeolite W-silicalite-1 was synthesized similarly using the above chemical ratio and process, but without the addition of an Al source. As comparative samples, non-framework hybrid zeolites without tungsten were also synthesized, and named ZSM-5 and silicalite-1.
2.3. Characterization methods
The powder X-ray diffraction (XRD) data was collected on an XRD-7000S powder diffractometer (Shimadzu, Japan) with Cu Kα radiation (λ = 0.154 nm) operated at 40 kV and 40 mA. The samples were placed in a glass sample holder and scanned from 2θ = 5 to 40° with step size of 0.02° and scanning rate of 2° min−1. The Fourier transform infrared (FTIR) spectra of the samples were recorded using a Cary 660 Spectrum One FTIR spectrometer (Agilent Technologies, Australia) within the wavenumber range of 3600–400 cm−1. Before this, the samples were dried under vacuum for 2 h at 100 °C to avoid signal interference from adsorbed water. The inductively coupled plasma optical emission spectrometry (ICP-OES) analysis was performed on an Optima 8300DV spectrometer (PerkinElmer, America) to determinate the element contents. Scanning electron microscopy (SEM) images were obtained on an ULTRA PLUS microscope (ZEISS, Germany) with a working distance of 8.7 mm at an accelerating voltage of 15 kV. The N2 adsorption–desorption isotherm at −196 °C was measured with an ASAP 2020 adsorption instrument (Micromeritics, USA). Prior to the test, the samples were degassed at 350 °C for 5 h. The specific surface area, micropore volume, micropore distribution and mesoporous distribution were calculated by the Brunauer–Emmett–Teller (BET) method, t-plot method, Horvath–Kawazoe (HK) method and Barrett–Joyner–Halenda (BJH) method, respectively. The total pore volume was calculated at a relative pressure of P/P0 = 0.995.
2.4. Adsorption measurements
CO2, N2 and O2 gas adsorption capacity measurements were carried out on a 3H-2000PH adsorption instrument (BeiShiDe, China) with isotherms at −15, 0, 25 and 50 °C. The temperature was controlled by heating and cooling of the circulating glycol thermal fluid. The sample was pretreated at 350 °C for 5 h under a vacuum of 10−5 bar, and adsorption was carried out in a pressure range from approximately 0.01 to 15 bar. Water vapor adsorption–desorption isotherms were measured using a 3H-2000 PW gravimetric vapor adsorption instrument (BeiShiDe, China). During the test, the relative pressure was controlled at the target pressure through the volatilization of pure steam into the vacuum test chamber, and the temperature was constant at 25 °C. Before the sample was assembled, it was heated under the same conditions as above in order to remove moisture and impurities.
3. Results and discussion
3.1. Characterization
Fig. 1 shows the XRD patterns of the synthesized zeolites W-ZSM-5, W-silicalite-1, ZSM-5 and silicalite-1. The MFI-type structure diffraction peaks at 7.8°, 8.9°, 23.5°, 23.7° and 24.1° are observed for all samples, and correspond to the (101), (200), (051), (033) and (133) crystal planes respectively.31 This indicates that the incorporation of tungsten does not affect the zeolite framework type, and there are no diffraction peaks for Al2O3 or WO3 crystals. For W-ZSM-5 and ZSM-5, the intensity of the peaks at 2θ = 7–9° is relatively low due to the Si/Al content ratio.32 The unit cell parameters of the samples are listed in Table 1. The differences in the values of the unit cell volume and the interplanar spacing indicate that the zeolite framework contains a mixture of tungsten and aluminum atoms. Fig. 2 shows the FTIR spectra of the samples in the wavenumber ranges of 3600–3300 and 1800–400 cm−1. The strong, broad bands at 1234 and 1072 cm−1 are due to the T–O stretching vibration and the asymmetric stretching vibration of the TO4 tetrahedron, respectively (T = Si, Al, W). The bands at 800, 547, and 451 cm−1 are associated with the symmetric stretching vibrations of the tetrahedrons, typical vibrations connected to the outside of the tetrahedrons of the MFI framework structure, and Si–O bending vibrations, respectively. The bands at 3450 and 1630 cm−1 are ascribed to the OH stretching vibration of the silanol groups and the HOH bending vibration resulting from extended hydrogen bonding.33–35 It can be clearly seen that the doping of tungsten atoms in the zeolite framework prevents the formation of silanol and O–H groups. The quantitative elemental analysis is summarized in Table 2. W atoms are present in the hybrid frameworks of W-ZSM-5 and W-silicalite-1, which is consistent with the XRD and FTIR findings. Fig. 3 shows SEM images of the microscopic appearance of the morphological features. The ZSM-5 type zeolite consists of agglomerated small particle crystals, and silicalite-1 consists of single angular crystals. The incorporation of tungsten has no effect on the appearance of the morphology, and only affects the internal framework. The crystal size of W-silicalite-1 is approximately 100 nm, compared with 200 nm for silicalite-1. Fig. 4 shows the N2 adsorption–desorption isotherms at liquid nitrogen temperature, and the corresponding pore size distributions calculated by the HK and BJH models are shown in Fig. 5. The main difference in the isotherms is between the ZSM-5 and silicalite-1 types, in which a hysteresis loop is present for silicalite-1 due to the larger size of the mesopores. This may be due to reorientation of the nitrogen molecules, which increases the packing density and creates a more favorable quadrupole–quadrupole interaction. The step in the adsorption branch for W-silicalite-1 was located at higher P/P0 compared to silicalite-1. The reason for the shift in this step is that W-silicalite-1 has 10% more mesopores than silicalite-1. The pore size properties of ZSM-5 are minimally affected by tungsten, but its influence on the BET surface area and mesopore ratio of silicalite-1 is not negligible. The detailed textural properties including surface area, pore volume and pore size are summarized in Table 3. It is seen that the presence or absence of Al atoms in the zeolite framework structure has a greater impact on the properties of the potential adsorption pores and channels by comparison.
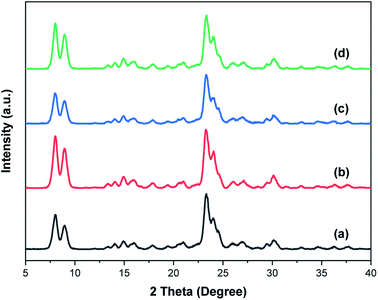 |
| Fig. 1 XRD patterns of (a) W-ZSM-5, (b) W-silicalite-1, (c) ZSM-5 and (d) silicalite-1. | |
Table 1 Unit cell parameters calculated from powder diffraction of the samples
Sample |
Unit cell (Å) |
Unit cell volume (Å3) |
Interplanar spacing (051) |
a |
b |
c |
W-ZSM-5 |
19.9895 |
19.8621 |
13.3258 |
5290.78 |
3.8069 |
W-silicalite-1 |
19.9264 |
19.8092 |
13.2528 |
5231.21 |
3.7959 |
ZSM-5 |
19.9927 |
19.8649 |
13.3040 |
5283.70 |
3.8068 |
Silicalite-1 |
19.8786 |
20.3437 |
13.2802 |
5370.56 |
3.8903 |
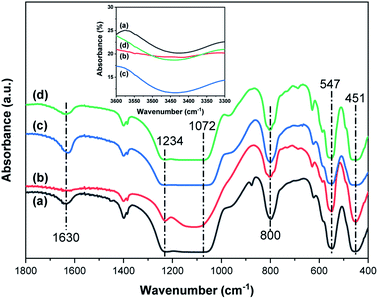 |
| Fig. 2 FTIR spectra of (a) W-ZSM-5, (b) W-silicalite-1, (c) ZSM-5 and (d) silicalite-1. | |
Table 2 Elemental analysis from ICP-OES of the samples (at%)
Sample |
Na |
Si |
Al |
O |
W |
W-ZSM-5 |
0.21 |
42.76 |
0.72 |
55.62 |
0.68 |
W-silicalite-1 |
0.25 |
44.46 |
0.00 |
54.55 |
0.74 |
ZSM-5 |
0.18 |
41.73 |
0.92 |
57.16 |
0.00 |
Silicalite-1 |
0.05 |
42.63 |
0.00 |
57.32 |
0.00 |
 |
| Fig. 3 SEM images of (a) W-ZSM-5, (b) W-silicalite-1, (c) ZSM-5 and (d) silicalite-1. | |
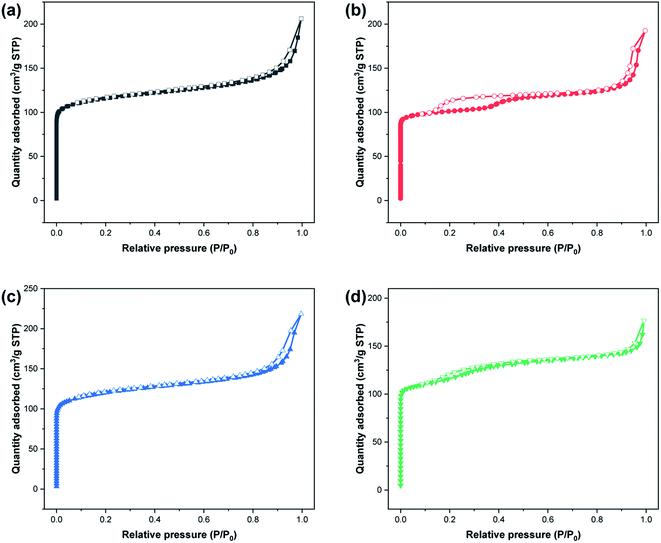 |
| Fig. 4 N2 adsorption–desorption isotherms of (a) W-ZSM-5, (b) W-silicalite-1, (c) ZSM-5 and (d) silicalite-1. Filled symbols: adsorption; empty symbols: desorption. | |
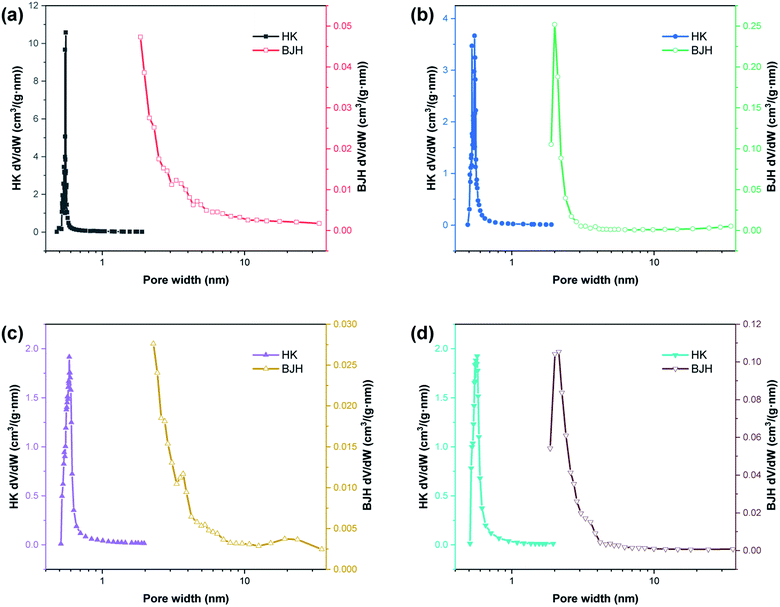 |
| Fig. 5 HK and BJH pore size distributions of (a) W-ZSM-5, (b) W-silicalite-1, (c) ZSM-5 and (d) silicalite-1. | |
Table 3 Textural properties of the prepared samples
Sample |
SBET (m2 g−1) |
Vtotal (cm3 g−1) |
Vmicro (cm3 g−1) |
Mesopore ratio (%) |
DHK (nm) |
DBJH (nm) |
W-ZSM-5 |
400 |
0.319 |
0.163 |
48.90 |
0.55 |
1.85 |
W-silicalite-1 |
351 |
0.298 |
0.176 |
40.94 |
0.55 |
2 |
ZSM-5 |
416 |
0.338 |
0.172 |
49.11 |
0.59 |
1.85 |
Silicalite-1 |
404 |
0.273 |
0.191 |
30.04 |
0.57 |
2.14 |
3.2. CO2 adsorption
Fig. 6 shows the adsorption isotherms of CO2 on W-ZSM-5 and W-silicalite-1 at −15, 0, 25 and 50 °C at pressures up to 15 bar. The adsorption equilibrium curve is well consistent with the typical Langmuir isotherm, which is type I according to the IUPAC classification.36 It should be pointed out that CO2 enters the zeolite sample as a single layer in a reversible adsorption process, and this is a result of volume filling due to most of the space being microporous. The steric effect between the adsorbate and the pore structure of the adsorbent is negligible due to the sufficient pore volume. As the pressure reaches 15 bar, the isotherm shape becomes flat, indicating that the material is close to adsorption saturation. The measured maximum adsorption capacities of W-ZSM-5 and W-silicalite-1 are 81.69 and 69.96 cm3 g−1, respectively. For single layer adsorption, the difference in adsorption amount between the two samples is caused by the specific surface area and pore volume. Furthermore, although the CO2 molecule is non-polar, it has a very large quadrupole and can be attracted by the electrostatic field in the zeolite. Therefore, the difference in the atoms causing the electronegativity in the zeolite framework also affects the number of adsorption sites. Adsorption decreases with increasing temperature due to the increase in the gas kinetic energy, which is determined by the physical adsorption characteristics. Since it is an exothermic process, heat rapidly accumulates as the adsorption process progresses, and thus high temperatures are unfavorable for the reaction.
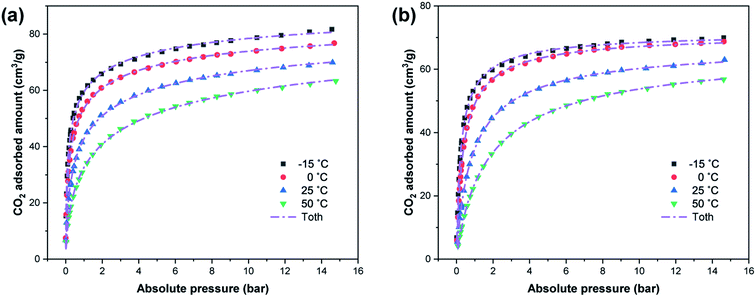 |
| Fig. 6 Adsorption isotherms of CO2 on (a) W-ZSM-5 and (b) W-silicalite-1 at different temperatures. | |
In Fig. 6 and 7, the dashed lines represent the CO2 adsorption data as fitted by the Toth model. In the process of designing an adsorption device, it is necessary to rely on an adsorption model to evaluate the adsorption properties of the adsorbent. The Toth model is actually a three-parameter improved Langmuir model, which has the advantage of accurately describing adsorption at high pressure, and is suitable for predicting the saturated adsorption capacity of the adsorbent. It assumes that the adsorption sites have a quasi-Gaussian distribution and that most of their adsorption energies are less than the peak adsorption energy. It is given by the following equation:37–39
|
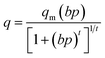 | (1) |
|
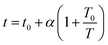 | (2) |
where
q is the equilibrium adsorbed amount at adsorbate gas pressure
p,
qm is the maximum adsorbed amount,
b is the Toth model adsorption constant,
t is a constant representing the heterogeneity of the adsorbent,
t0 is the heterogeneity constant at the reference temperature
T0,
T is the adsorption temperature and
α describes the temperature dependence.
The CO2 adsorption isotherm fitting parameters obtained from the Toth model are listed in Table 4, and the correlation coefficient R2 is in a relatively high range of 0.9983–0.9998. With the increase in temperature, the saturated adsorption amount on all adsorption sites on the adsorbent surface covered by adsorbate, represented by qm, decreases, which is because the process is a physical adsorption process. b represents the activity coefficient of the competitive process between the solvent and the adsorbate that occupies the binding site on the solid surface. It can be seen from its value that the adsorbate has a higher thermodynamic activity coefficient in its bound state and free state at lower temperature. By calculation, the CO2 adsorption amounts of the samples at 1 bar are up to 68–72% of those at 15 bar at 0 °C. However, this value becomes lower as the temperature rises, and it drops to 50% at 50 °C. Therefore, there is a larger amount of adsorption when the pressure exceeds atmospheric pressure, especially at high temperatures. t is further away from unity for W-ZSM-5 and ZSM-5 than W-silicalite-1 and silicalite-1, indicating that the adsorption systems of W-ZSM-5 and ZSM-5 are more heterogeneous.40 The difference in the values of t can be explained by the fact that Al atoms have a greater effect on heterogeneity. The greater the heterogeneity of the adsorbent, the larger the CO2 adsorption capacity achieved.
Table 4 Toth isotherm parameters for CO2 adsorption at different temperatures
Sample |
Temperature (°C) |
qm (cm3 g−1) |
b |
t |
R2 |
q15 bar (cm3 g−1) |
W-ZSM-5 |
−15 |
96.524 |
53.172 |
0.393 |
0.9984 |
80.79 |
0 |
91.914 |
24.654 |
0.424 |
0.9983 |
76.39 |
25 |
91.239 |
8.885 |
0.433 |
0.9984 |
70.22 |
50 |
97.669 |
4.239 |
0.404 |
0.9988 |
63.89 |
W-silicalite-1 |
−15 |
72.038 |
5.679 |
0.787 |
0.9993 |
69.36 |
0 |
71.670 |
3.370 |
0.824 |
0.9995 |
68.38 |
25 |
68.618 |
1.451 |
0.817 |
0.9998 |
62.40 |
50 |
68.216 |
0.717 |
0.795 |
0.9998 |
57.15 |
ZSM-5 |
0 |
89.481 |
11.322 |
0.482 |
0.9991 |
75.71 |
Silicalite-1 |
0 |
73.824 |
2.953 |
0.781 |
0.9994 |
69.21 |
Fig. 7 shows a comparison of the CO2, N2, and O2 adsorption equilibrium data for the four samples, and the Toth model fitting curves. The Toth isotherm fitting parameters for N2 and O2 are listed in Table 5, and R2 is close to 1. Although CO2, N2 and O2 are all non-polar molecules, the quadrupole moments of N2 (1.2 × 10−26 esu cm2) and O2 (0.4 × 10−26 esu cm2) are lower compared to that of CO2 (4.3 × 10−26 esu cm2).41,42 The value of t for CO2 is less than for N2 and O2, and that for O2 is the largest. Thus, the adsorption amount of CO2 is higher than that of N2 and O2, and this results in a selective adsorption performance.
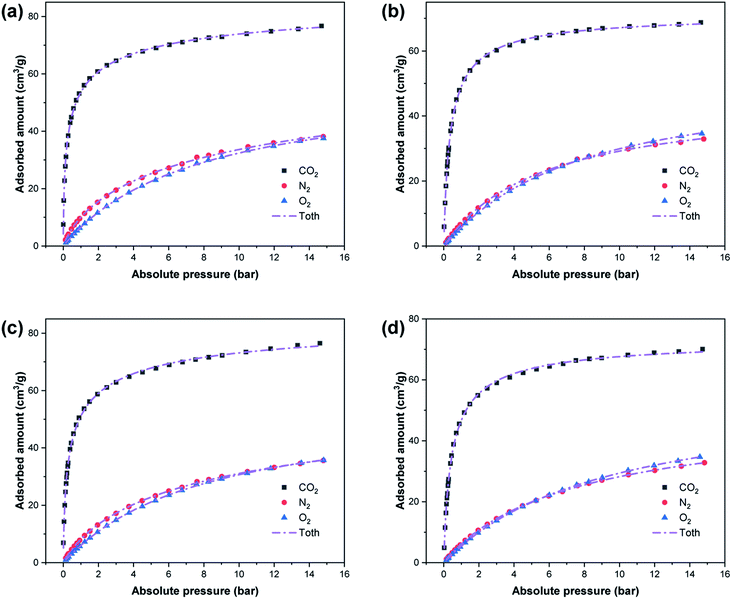 |
| Fig. 7 Adsorption isotherms and Toth model fitting for CO2, N2 and O2 on (a) W-ZSM-5, (b) W-silicalite-1, (c) ZSM-5 and (d) silicalite-1 at 0 °C. | |
Table 5 Toth isotherm parameters for N2 and O2 adsorption at 0 °C
Sample |
Gas |
qm (cm3 g−1) |
b |
t |
R2 |
q15 bar (cm3 g−1) |
W-ZSM-5 |
N2 |
77.195 |
0.262 |
0.555 |
0.9996 |
38.68 |
O2 |
58.385 |
0.128 |
0.986 |
0.9998 |
38.00 |
W-silicalite-1 |
N2 |
45.918 |
0.176 |
0.996 |
0.9997 |
33.22 |
O2 |
51.022 |
0.122 |
1.101 |
0.9999 |
35.05 |
ZSM-5 |
N2 |
62.738 |
0.195 |
0.695 |
0.9999 |
35.89 |
O2 |
54.106 |
0.123 |
1.041 |
0.9999 |
36.00 |
Silicalite-1 |
N2 |
52.235 |
0.141 |
0.896 |
0.9999 |
32.92 |
O2 |
57.041 |
0.106 |
1.002 |
0.9999 |
35.07 |
The molar ratios of the adsorbed amounts of CO2/N2 and N2/O2 at 0.1, 1 and 15 bar are presented in Fig. 8. The adsorption selectivity for CO2/N2 and N2/O2 is determined by the following equation:
|
 | (3) |
where
S is the adsorption selectivity,
q is the adsorption quantity (cm
3 g
−1) at partial pressure
p (bar), and
i and
j represent CO
2, N
2 or O
2.
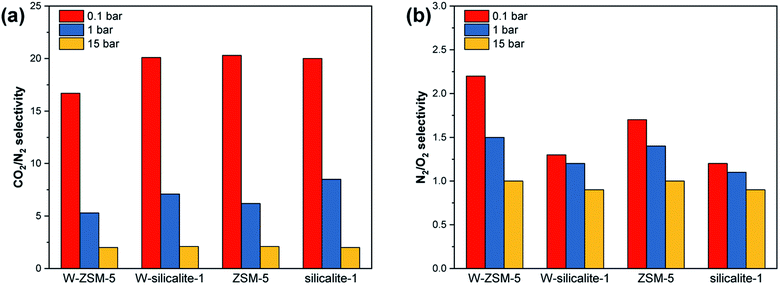 |
| Fig. 8 Adsorption selectivity for (a) CO2/N2 and (b) N2/O2. | |
There is a wider pressure range in the Henry type isotherm for N2 and O2 compared to CO2, and thus substantial selectivity is observed at less than 1 bar. The W and Al atoms in the framework have little effect on the selectivity at a high pressure of 15 bar, and mainly affect the selectivity in the low pressure region. The W-doped ZSM-5 exhibits reduced CO2/N2 selectivity and enhanced N2/O2 selectivity. The selectivity of the silicalite-1 type zeolite is hardly affected by the W-doping, so the selectivity difference is derived from the combined effects of W and Al atoms.
3.3. Water vapor adsorption
Fig. 9 shows the water vapor adsorption properties of the samples. It can be observed that all isotherms were linear and the adsorption process occurred in the Henry’s law region due to the low temperature and saturated vapor pressure.43 The H2O adsorption amounts of W-silicalite-1 and silicalite-1 are relatively low, indicating that the presence or absence of Al largely determines the capacity for adsorbing water. The H2O adsorption amounts of W-ZSM-5 and ZSM-5 are similar, and thus the doping with W has a greater influence on silicalite-1 in terms of absolute adsorption capacity. H2O is a polar molecule, and the presence of the polarization energy causes its adsorption affinity to be several times that of CO2.44 As shown in Fig. 9(b), the CO2/H2O adsorption selectivity of W-ZSM-5 is slightly higher than that of ZSM-5, and that of W-silicalite-1 is three times that of silicalite-1. The flexible coordination state of tungsten prevents the formation of silanol, thereby reducing the number of H2O adsorption sites.45 In the framework without aluminum, tungsten plays an important role in preventing water vapor adsorption. This demonstrates that under humid working conditions, the performance in adsorptive separation of CO2 is improved due to the W-doping in the framework structures of ZSM-5 and silicalite-1.
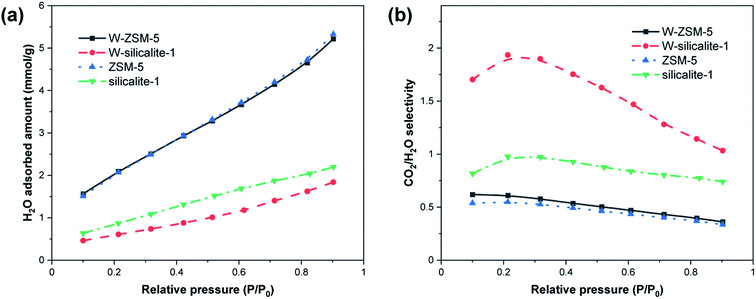 |
| Fig. 9 H2O vapor adsorption properties. (a) Isotherms at 25 °C and (b) selectivity for CO2/H2O. | |
4. Conclusions
In this work, framework hybrid zeolites with tungsten, W-ZSM-5/silicalite, were prepared from rice husk ash. The CO2 and water vapor adsorption properties of the samples were explored, and then the CO2/H2O adsorption selectivity was evaluated. The characterization results show that the involvement of tungsten does not affect the crystal structure type of the zeolite, but prevents the formation of silanol and O–H groups. Through morphology and pore analyses, it is shown that tungsten has no effect on the appearance of the morphology, but the change in the framework leads to changes in the intrinsic specific surface area, pore volume and pore size distribution. The adsorption capacities of W-ZSM-5 and W-silicalite-1 are significantly improved, and the maximum capacities measured are 81.69 and 69.96 cm3 g−1, respectively. The adsorption isotherms of CO2, N2 and O2 are perfectly fitted by the Toth model. The flexible coordination state of tungsten prevents the formation of silanol, and this leads to the CO2/H2O selectivity of W-ZSM-5 being slightly higher than that of ZSM-5, and the CO2/H2O selectivity of W-silicalite-1 being three times that of silicalite-1. In summary, the framework hybrids W-ZSM-5/silicalite-1 are potential adsorbents for separating CO2 under humid industrial conditions.
Conflicts of interest
The authors declare that they have no known competing financial interests or personal relationships that could have appeared to influence the work reported in this paper.
Acknowledgements
This work was funded by the National Natural Science Foundation of China (No. 51904073), the National Key Research and Development Project (No. 2019YFC1905200 and 2017YFB0304001), the Natural Science Foundation of Liaoning Province (No. 2020-BS-053), and the Postdoctoral Research Foundation of Northeastern University (No. 20190304).
References
- M. Obersteiner, J. Bednar, F. Wagner, T. Gasser, P. Ciais, N. Forsell, S. Frank, P. Havlik, H. Valin and I. A. Janssens, et al., How to spend a dwindling greenhouse gas budget, Nat. Clim. Change, 2018, 8(1), 7 CrossRef.
- D. Fang, X. Zhang, Q. Yu, T. C. Jin and L. Tian, A novel method for carbon dioxide emission forecasting based on improved Gaussian processes regression, J. Cleaner Prod., 2018, 173, 143–150 CrossRef CAS.
- G. Zhang, P. Zhao, Y. Xu, Z. Yang, H. Cheng and Y. Zhang, Structure property–CO2 capture performance relations of amine-functionalized porous silica composite adsorbents, ACS Appl. Mater. Interfaces, 2018, 10(40), 34340–34354 CrossRef CAS PubMed.
- A. A. Olajire, Recent progress on the nanoparticles-assisted greenhouse carbon dioxide conversion processes, J. CO2 Util., 2018, 24, 522–547 CrossRef CAS.
- IEA, CO2 emissions from fuel combustion 2018, International Energy Agency, Paris, 2018 Search PubMed.
- F. E. Epiepang, J. Li, Y. Liu and R. T. Yang, Low-pressure performance evaluation of CO2, H2O and CH4 on Li-LSX as a superior adsorbent for air prepurification, Chem. Eng. Sci., 2016, 147, 100–108 CrossRef CAS.
- F. Chen, Y. Wang, D. Bai, M. He, X. Gao and Y. He, Selective adsorption of C2H2 and CO2 from CH4 in an isoreticular series of MOFs constructed from unsymmetrical diisophthalate linkers and the effect of alkoxy group functionalization on gas adsorption, J. Mater. Chem. A, 2018, 6(8), 3471–3478 RSC.
- Y. Yang, C. Y. Chuah and T.-H. Bae, Polyamine-appended porous organic polymers for efficient post-combustion CO2 capture, Chem. Eng. J., 2019, 358, 1227–1234 CrossRef CAS.
- A. M. Yousef, W. M. El-Maghlany, Y. A. Eldrainy and A. Attia, New approach for biogas purification using cryogenic separation and distillation process for CO2 capture, Energy, 2018, 156, 328–351 CrossRef CAS.
- F. Vega, M. Cano, S. Camino, B. Navarrete and J. Camino, Evaluation of the absorption performance of amine-based solvents for CO2 capture based on partial oxy-combustion approach, Int. J. Greenhouse Gas Control, 2018, 73, 95–103 CrossRef CAS.
- C.-P. Zhang, B. Li and Z.-G. Shao, First-principle investigation of CO and CO2 adsorption on Fe-doped penta-graphene, Appl. Surf. Sci., 2019, 469, 641–646 CrossRef CAS.
- R. Castro-Muñoz, V. Fila, V. Martin-Gil and C. Muller, Enhanced CO2 permeability in Matrimid (R) 5218 mixed matrix membranes for separating binary CO2/CH4 mixtures, Sep. Purif. Technol., 2019, 210, 553–562 CrossRef.
- S. Xiang, Y. He, Z. Zhang, H. Wu, W. Zhou, R. Krishna and B. Chen, Microporous metal–organic framework with potential for carbon dioxide capture at ambient conditions, Nat. Commun., 2012, 3, 954–959 CrossRef PubMed.
- J. Yan, Y. Li, H. Li, Y. Zhou, H. Xiao, B. Li and X. Ma, Effective removal of ruthenium(III) ions from wastewater by amidoxime modified zeolite X, Microchem. J., 2019, 145, 287–294 CrossRef CAS.
- D. K. Wanigarathna, B. Liu and J. Gao, Adsorption separation of R134a, R125, and R143a fluorocarbon mixtures using 13X and surface modified 5A zeolites, AIChE J., 2018, 64(2), 640–648 CrossRef CAS.
- A. Zukal, M. Shamzhy, M. Kubů and J. Čejka, The effect of pore size dimensions in isoreticular zeolites on carbon dioxide adsorption heats, J. CO2 Util., 2018, 24, 157–163 CrossRef CAS.
- N. Setthaya, P. Chindaprasirt, S. Yin and K. Pimraksa, TiO2–zeolite photocatalysts made of metakaolin and rice husk ash for removal of methylene blue dye, Powder Technol., 2017, 313, 417–426 CrossRef CAS.
- R. Khoshbin and R. Karimzadeh, The beneficial use of ultrasound in free template synthesis of nanostructured ZSM-5 zeolite from rice husk ash used in catalytic cracking of light naphtha: effect of irradiation power, Adv. Powder Technol., 2017, 28(3), 973–982 CrossRef CAS.
- S. Jesudoss, J. J. Vijaya, K. Kaviyarasu, L. J. Kennedy, R. J. Ramalingam and H. A. Al-Lohedan, Anti-cancer activity of hierarchical ZSM-5 zeolites synthesized from rice-based waste materials, RSC Adv., 2018, 8(1), 481–490 RSC.
- R. Pode, Potential applications of rice husk ash waste from rice husk biomass power plant, Renewable Sustainable Energy Rev., 2016, 53, 1468–1485 CrossRef.
- J. Prasara-A and S. H. Gheewala, Sustainable utilization of rice husk ash from power plants: a review, J. Cleaner Prod., 2017, 167, 1020–1028 CrossRef.
- H. Chen, Y. J. Zhang, P. Y. He and C. J. Li, Synthesis, characterization and modification of monolithic ZSM-5 from geopolymer for CO2 capture: experiments and DFT calculations, Energy, 2019, 179, 422–430 CrossRef CAS.
- P. J. Harlick and F. H. Tezel, An experimental adsorbent screening study for CO2 removal from N2, Microporous Mesoporous Mater., 2004, 76(1–3), 71–79 CrossRef CAS.
- Q. Liu, P. He, X. Qian, Z. Fei, Z. Zhang, X. Chen, J. Tang, M. Cui, X. Qiao and Y. Shi, Enhanced CO2 adsorption performance on hierarchical porous ZSM-5 zeolite, Energy Fuels, 2017, 31(12), 13933–13941 CrossRef CAS.
- A. Kalantarifard, A. Ghavaminejad and G. S. Yang, High CO2 adsorption on improved ZSM-5 zeolite porous structure modified with ethylenediamine and desorption characteristics with microwave, J. Mater. Cycles Waste Manage., 2017, 19(1), 394–405 CrossRef CAS.
- Z. Lin, J. Wei, L. Geng, D. Mei and L. Liao, An amine double functionalized composite strategy for CO2 adsorbent preparation using a ZSM-5/KIT-6 composite as a support, Energy Technol., 2018, 6(9), 1618–1626 CrossRef CAS.
- S. J. Datta, C. Khumnoon, Z. H. Lee, W. K. Moon, S. Docao, T. H. Nguyen, I. C. Hwang, D. Moon, P. Oleynikov and O. Terasaki, et al., CO2 capture from humid flue gases and humid atmosphere using a microporous coppersilicate, Science, 2015, 350(6258), 302–306 CrossRef CAS PubMed.
- Q. Ke, T. Sun, X. Wei, Y. Guo and S. Wang, Enhanced trace carbon dioxide capture on heteroatom-substituted RHO zeolites under humid conditions, ChemSusChem, 2017, 10(21), 4207–4214 CrossRef CAS PubMed.
- J. Ding, H. Xu, H. Wu and P. Wu, Liquid-phase oxidation of ethylamine to acetaldehyde oximes over tungsten-doped zeolites, Sci. China: Chem., 2017, 60(7), 942–949 CrossRef CAS.
- Y. Wang, T. Du, Z. Qiu, Y. Song, S. Che and X. Fang, CO2 adsorption on polyethylenimine-modified ZSM-5 zeolite synthesized from rice husk ash, Mater. Chem. Phys., 2018, 207, 105–113 CrossRef CAS.
- Y. Liu, S. Han, D. Guan, S. Chen, Y. Wu, Y. Yang and N. Jiang, Rapid green synthesis of ZSM-5 zeolite from leached illite clay, Microporous Mesoporous Mater., 2019, 280, 324–330 CrossRef CAS.
- C. Zhang, Q. Wu, C. Lei, S. Pan, C. Bian, L. Wang, X. Meng and F.-S. Xiao, Solvent-free and mesoporogen-free synthesis of mesoporous aluminosilicate ZSM-5 zeolites with superior catalytic properties in the methanol-to-olefins reaction, Ind. Eng. Chem. Res., 2017, 56(6), 1450–1460 CrossRef CAS.
- Z. Lin, J. Wei, L. Geng, D. Mei and L. Liao, Adsorption of carbon dioxide by a novel amine impregnated ZSM-5/KIT-6 composite, RSC Adv., 2017, 7(86), 54422–54430 RSC.
- I. O. Ali, A. M. Hassan, S. M. Shaaban and K. S. Soliman, Synthesis and characterization of ZSM-5 zeolite from rice husk ash and their adsorption of Pb2+ onto unmodified and surfactant-modified zeolite, Sep. Purif. Technol., 2011, 83, 38–44 CrossRef.
- K. Barbera, F. Bonino, S. Bordiga, T. V. Janssens and P. Beato, Structure-deactivation relationship for ZSM-5 catalysts governed by framework defects, J. Catal., 2011, 280(2), 196–205 CrossRef CAS.
- Y. Gensterblum, A. Merkel, A. Busch and B. M. Krooss, High-pressure CH4 and CO2 sorption isotherms as a function of coal maturity and the influence of moisture, Int. J. Coal Geol., 2013, 118, 45–57 CrossRef CAS.
- V. Garshasbi, M. Jahangiri and M. Anbia, Equilibrium CO2 adsorption on zeolite 13X prepared from natural clays, Appl. Surf. Sci., 2017, 393, 225–233 CrossRef CAS.
- H. Yi, Z. Wang, H. Liu, X. Tang, D. Ma, S. Zhao, B. Zhang, F. Gao and Y. Zuo, Adsorption of SO2, NO, and CO2 on activated carbons: equilibrium and thermodynamics, J. Chem. Eng. Data, 2014, 59(5), 1556–1563 CrossRef CAS.
- D. P. Vargas, L. Giraldo and J. C. Moreno-Piraján, CO2 adsorption on activated carbon honeycomb-monoliths: a comparison of Langmuir and Toth models, Int. J. Mol. Sci., 2012, 13(7), 8388–8397 CrossRef CAS PubMed.
- M. G. Plaza, I. Durán, N. Querejeta, F. Rubiera and C. Pevida, Experimental and simulation study of adsorption in postcombustion conditions using a microporous biochar. 1. CO2 and N2 adsorption, Ind. Eng. Chem. Res., 2016, 55(11), 3097–3112 CrossRef CAS.
- S. J. Chen, M. Zhu, Y. Fu, Y. X. Huang, Z. C. Tao and W. L. Li, Using 13X, LiX, and LiPdAgX zeolites for CO2 capture from post-combustion flue gas, Appl. Energy, 2017, 191, 87–98 CrossRef CAS.
- Y. Fu, Y. Liu, X. Yang, Z. Li, L. Jiang, C. Zhang, H. Wang and R. T. Yang, Thermodynamic analysis of molecular simulations of N2 and O2 adsorption on zeolites under plateau special conditions, Appl. Surf. Sci., 2019, 480, 868–875 CrossRef CAS.
- M. Pera-Titus, On an isotherm thermodynamically consistent in Henry’s region for describing gas adsorption in microporous materials, J. Colloid Interface Sci., 2010, 345(2), 410–416 CrossRef CAS PubMed.
- T. D. Pham, Q. Liu and R. F. Lobo, Carbon dioxide and nitrogen adsorption on cation-exchanged SSZ-13 zeolites, Langmuir, 2013, 29(2), 832–839 CrossRef CAS PubMed.
- J. Grand, S. N. Talapaneni, A. Vicente, C. Fernandez, E. Dib, H. A. Aleksandrov, G. N. Vayssilov, R. Retoux, P. Boullay, J.-P. Gilson, V. Valtchev and S. Mintova, One-pot synthesis of silanol-free nanosized MFI zeolite, Nat. Mater., 2017, 16(10), 1010–1015 CrossRef CAS PubMed.
|
This journal is © The Royal Society of Chemistry 2020 |
Click here to see how this site uses Cookies. View our privacy policy here.