DOI:
10.1039/D0RA03405C
(Paper)
RSC Adv., 2020,
10, 29139-29146
Nutrient removal by Rotala rotundifolia: a superior candidate for ecosystem remediation at low temperatures†
Received
17th April 2020
, Accepted 20th July 2020
First published on 6th August 2020
Abstract
Temperature is an extremely important factor affecting the nutrient (mainly nitrogen and phosphorus) removal of aquatic macrophytes. A novel submersed Rotala rotundifolia was separately cultivated at room and low temperatures to investigate its ability for nutrient removal. The physiological metabolism was analyzed to explore the mechanism of removing nutrients under a wide temperature range. The results showed that the removal efficiency (RE) of nutrients at low temperature was competitive with that obtained at normal temperature, demonstrating that temperature exerted no obvious influence on the nutrient removal by R. rotundifolia. The root vitality at 5 °C rose from the initial 0.26 to 1.5 mg g−1 h−1, whereas it fell by 38.66% at 10 °C, 28.74% at 20 °C and 5.15% at 30 °C. The peroxidase (POD) activity at 5 °C showed the maximum value on day 7 followed by a notable decline on day 21. All the peak values of soluble sugar and protein as well as MDA showed up at 5 °C and they were 5.5, 437.9 and 10.1 mg g−1, respectively. Chlorophyll a and b reached 8.4 and 4.4 mg g−1 on day 28, respectively, with a total chlorophyll content (a plus b) of 12.4 mg g−1 at 5 °C, all of which were higher than that at 30 °C. These results validated that R. rotundifolia could be a superior candidate suitable for in situ application.
1. Introduction
As key primary producers with rates of productivity greater than most terrestrial plant community systems, submersed aquatic vegetation (SAV) is an extremely valuable resource for the ecosystem, the value of which can be globally quantified and estimated at approximately $1.9 trillion per year.1 SAV in clear water systems simultaneously provides a habitat for invertebrate prey and acts as a refuge for small fishes, and thus could cause substantive shifts in the fish community.2 SAV beds can slow water movement and reduce bed shear stress, promoting sedimentation and reducing suspension, which makes it an important factor in the turbidity decline.3 Most noticeably, SAV is characterized by its ability to assimilate nutrients and limit the resuspension of nutrients in the overlying water, resulting in a lower nutrient level in waters dominated by SAV.4 Therefore, the re-establishment of SAV is recognized as an efficient approach to improve water quality and restore the ecological functions of degraded aquatic ecosystems.5
Owing to the seasonal changes of summer to cold winter, a big challenge for the sustainable operation of SAVs would have to be faced as a number of SAVs, such as Myriophyllum spicatum, Ceratophyllum demersum and Vallisneria natans, do not live through the winter.6 The debris of these plants would again release nutrients, not only leading to the pollution of both water and sediment but also impacting microbial metabolism and growth with subsequent depressed contaminant removal.7 Nonetheless, the SAVs with an ability to endure low temperatures are seldom reported.8 Thereby, it is quite meaningful to explore and cultivate cold-resistant species that contribute to ecological restoration and submersed aquatic plant diversity.
Rotala rotundifolia is a perennial aquatic plant with both submerged and emergent forms. Emergent leaves are bright green, fleshy, and nearly round, whereas submersed leaves range from green to burgundy, are much thinner than the emergent plant material, and are lanceolate in shape.9 This species is reputed to possess medical and anti-microbial properties,10,11 and other characteristics like lead uptake and nickel accumulation have also been well-documented,12,13 but these studies were almost always conducted at room temperature since it is regarded as a plant suitable for tropical aquariums with water temperature ranging from 21 to 30 °C.14 Nevertheless, the submerged Rotala rotundifolia found in the remote valley in Wenzhou city, Zhejiang Province, China, is able to survive the winter under temperatures as low as 4 °C,15 implying a cold-resistant potential of R. rotundifolia. As such, there is a need to investigate the nutrient removal capability and physiological characteristics of this species in response to different temperatures, particularly to low temperature.
The primary objective of this study is to (1) evaluate the effect of temperature on the nutrient (mainly nitrogen and phosphorus) removal by R. rotundifolia and (2) explore the mechanisms of removing nutrients by R. rotundifolia at low temperature on the basis of physiological metabolism. All these results might provide vital implications for the sustainable application of R. rotundifolia in ecosystem remediation, even during the low-temperature period.
2. Materials and methods
2.1. Plant culture
R. rotundifolia was collected from a river located in Wenzhou City, China, and rinsed to remove invertebrate grazers and cultured with sediments from the same river in a greenhouse for 30 days.
2.2. Nutrient removal tests
Nutrient removal was carried out in a set of devices consisting of a water tank (1 m3), a pump and an eco-tank (2.0 m × 0.5 m × 0.5 m) loaded with an appropriate height of sediments. The water depth of the eco-tank was fixed at 0.45 m by an adjustable effluent weir. After the sturdy and synchronous plants were transplanted into the eco-tank, an acclimation of 7 days was conducted, following which artificial wastewater (Table 1) with a flow rate of 200 L d−1 controlled by a precise pump was injected. The influent and effluent water samples were taken out periodically for analysis. Experimental devices were separately placed in a greenhouse (around 28 °C) and on a roof (ambient temperature, as low as 4 °C was observed). An appropriate amount of glucose was supplemented to investigate the COD removal by plants.
Table 1 Constituents of artificial wastewater
KH2PO4 (mg L−1) |
NH4Cl (mg L−1) |
MgSO4 (mg L−1) |
CaCl2 (mg L−1) |
KCl (mg L−1) |
Iron solution (mL L−1) |
0.7 |
6.0 |
9.8 |
10.1 |
7.1 |
2.0 |
2.3. Physiological characteristics
The sturdy and synchronous plants were transplanted into twelve 3 L beakers. In order to simulate the in situ environment, each beaker was wrapped with a black plastic bag and loaded with a mixture of sediments (TP = 0.853 mg g−1 DW; TN = 2.024 mg g−1 DW) and culture medium (KH2PO4 0.6 mg L−1, MgSO4·7H2O 9.8 mg L−1, NH4Cl 4.0 mg L−1, CaCl2 10.1 mg L−1, KCl 7.1 mg L−1, iron solution 2.0 mL L−1) with the volume ratio of solid to liquid being 1
:
4. The selected experimental temperatures were 5 °C, 10 °C, 20 °C and 30 °C.
The measurements of dissolved oxygen (DO), pH and oxidation–reduction potential (ORP) in the overlying water were carried out every day at noon, while plant weight, height, blades, tillers, leaf length and width were determined every seven days. Peroxidase (POD) is identified as a major contributor to reactive oxygen species (ROS) production in plants, while POD-dependent ROS plays a role in the regulation of root growth,16 and therefore there would be a close relationship between POD and nutrient removal. To cope with low temperatures, plants have evolved a variety of efficient mechanisms that allow them to adapt to the adverse conditions, like increasing the level of metabolites (malondialdehyde, soluble sugar and protein)17 to maintain their normal growth accompanied with nitrogen uptake/removal. Taking these into account, the root vitality, POD activity and metabolites mentioned above were analyzed every seven days at 5 and 30 °C. The entire experiment lasted 28 days, during which deionized water was supplemented to the initial volume every five days.
2.4. Analytical methods
NH4+-N, TN, TP and COD were analyzed using Standard Methods.18 A portable multi-parameter meter (HQ40d, Hatch Company) was used to analyze DO, pH and ORP. The plant height, leaf length and width were measured directly by a ruler, and plant weight was measured with an electronic balance (YP2002, Shanghai, China). Soluble protein, POD activity, root vitality and chlorophyll were respectively determined through Coomassie Brilliant Blue staining,19 guaiacol,20 TTC,21 and acetone extraction methods. Malondialdehyde (MDA) and soluble sugar were detected following modified TBA methods.22,23
2.5. Statistical analysis
Each measurement was performed in triplicate and denoted as the mean plus deviation. All data analysis was conducted by SPSS16.0 and Origin pro 8.0. One-way ANOVA and LSD methods were adopted to determine significant differences at a significant level of P < 0.05. When the data was abnormal, it would go through an appropriate transformation. While variables approached the normal distribution without abnormal values, the Pearson correlation analysis was executed.
3. Results and discussion
3.1. Nutrient removal at normal temperature
Nutrient removal by R. rotundifolia was conducted for 45 days at an average temperature of 28 °C (Fig. 1). All the REs of NH4+-N nearly surpassed 90% except for day 20 and 25, the corresponding RE of which was 86.% and 79.5% (Fig. 1a), respectively. Though the RE of TN was lower than that of NH4+-N, it maintained around 80% most of the time and exceeded 90% on day 30 and 35 (Fig. 1b). A few reports have identified the primary mechanisms responsible for nitrogen removal as volatilization, plant uptake and nitrification/denitrification,24,25 out of which volatilization usually occurs at pH > 8.5. As such, the transformation of NH4+ to NH3 gas could be neglected since the pH was usually less than 8.5 (Fig. S1†). Moreover, it could be found that the nitrogen removal with the presence of plants was much higher than the control (without plants), indicating the minor role of natural processes (Fig. S2†). Therefore, nitrogen removal should be mainly attributed to a combined effect of plant uptake and nitrification/denitrification under our experimental conditions.
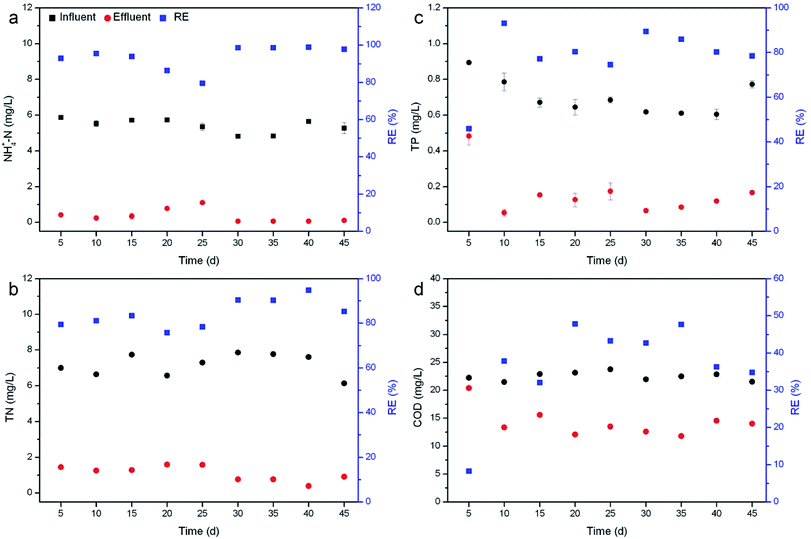 |
| Fig. 1 Removal of NH4+-N (a), TN (b), TP (c) and COD (d) by R. rotundifolia at normal temperature (approximately 28 °C). | |
The RE of TP fluctuated markedly within the first 25 days, with the greatest value of 93.1% on day 10 and the smallest value of 46% on day 5, and thereafter varied from 78.5% to 89.4% (Fig. 1c). Similarly, the RE of COD also showed the lowest value (8.3%) on day 5 but increased rapidly to 37.9% on day 10. From day 20 on, the RE ranged from 42.7% to 47.8%, yet it dropped to 34.8% at the end of the experiment (Fig. 1d). It was reported that phosphorus can be removed by sorption, complexation, precipitation and assimilation into microbial and plant biomass.26 While TP was not completely removed by R. rotundifolia, the RE still achieved 93.1%, indicative of its good ability for phosphorus removal. This was because R. rotundifolia has a high growth rate and strong reproduction ability (Table S1†) that was propitious for the P assimilation. Besides that, a strong root system could release more oxygen that was advantageous to the P precipitation. Likewise, the removal of COD was also closely related to the strong root system, which would provide both rhizosphere microorganisms and oxygen to facilitate the sedimentation, adsorption and degradation of organic substances. The corresponding conclusions have been demonstrated in several previous studies. For instance, rhizomicrobes could rapidly degrade phenol in illegally dumped industrial wastewater,27,28 indicative of the vital role rhizosphere microorganisms play in removing organic pollutants. In view of the synthetic wastewater used, however, the microbial diversity was not analyzed in the current study, yet it will be carried out in our ongoing experiments with real wastewater since the exploration on rhizomicrobial activities in real wastewater would be more meaningful.
3.2. Nutrient removal at low temperature
Nutrient removal by R. rotundifolia was conducted outdoors over a period from Oct 10th to Dec 15th (Fig. 2) in which the lowest temperature approached 4 °C (Fig. S3†). The REs of NH4+-N were all above 90%, other than on Nov 28th and Dec 4th at which the RE was 81.1% and 78.2% (Fig. 2a), respectively, while the TN maintained at approximately 50% after a month of operation (Fig. 2b). For TP removal, a watershed arose on Nov 8th, before which its RE was no more than 73% and after which the RE nearly rose linearly to 91.5% by Dec 15th (Fig. 2c). In regard to the COD, all the REs remained at around 60%, except for on Oct 10th, Oct 26th and Nov 21st (Fig. 2d).
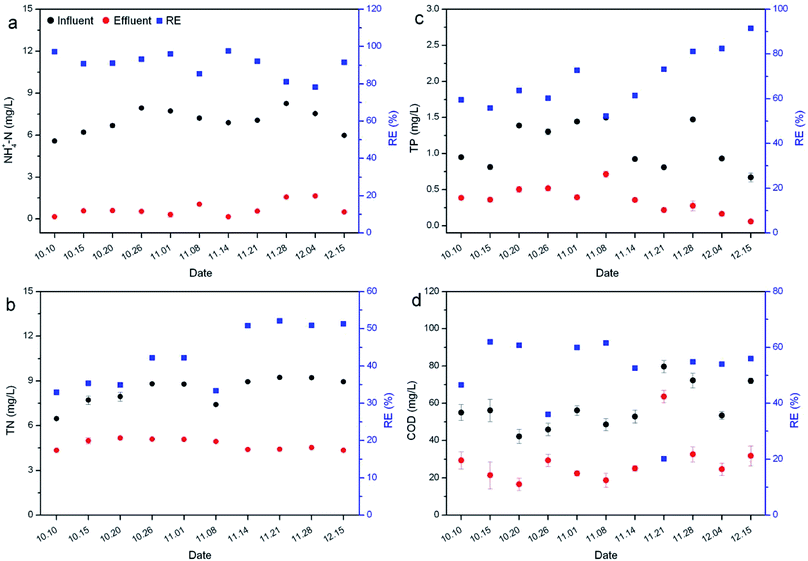 |
| Fig. 2 Removal of NH4+-N (a), TN (b), TP (c) and COD (d) by R. rotundifolia at low temperature. | |
In general, there would be a decline in the nitrification/denitrification rate at low temperatures owing to the decrease of oxygen availability. However, although a temperature of as low as 4 °C appeared in the outdoor experiments of this study, the REs of nutrients were comparable with those observed at normal temperatures. It is clear that the temperature did not significantly affect the nutrient removal by R. rotundifolia (p > 0.05), as opposed to a former report that the optimum temperature for nutrient removal was 30 °C,29 but consistent with another previous report in which the high average removal efficiencies of COD (92.45%), NH4+-N (93.70%) and TN (55.62%) were achieved in experimental SFCWs with Potamogeton crispus compared with SFCWs with other plants, indicating that SFCWs with hardy submerged plant P. crispus could be a more effective and sustainable strategy for removing organics and nitrogen in shallow nutrient-enriched river water ecosystems in cold climates.8 In consideration of the capability of nutrient removal under a broad temperature range, R. rotundifolia is a candidate comparable to P. crispus.
3.3. Mechanism discussion based on physiological metabolism
To clarify why temperature does not exert a great impact on the nutrient removal by R. rotundifolia, the physiological metabolism in response to different temperatures was explored in this section.
3.3.1. DO, pH and ORP in overlying water. The DO changing trend for the overlying water of R. rotundifolia is depicted in Fig. 3a and S4† (control). Overall, the DO climbed slowly regardless of temperature. DO varied from the initial 10.03, 10.86, 8.42 and 6.46 mg L−1 to 12.53, 11.67, 10.66 and 9.24 mg L−1 on day 28 when the temperature was 5, 10, 20 and 30 °C, respectively. Meanwhile, it can be observed that there was a marked difference among different temperatures. These phenomena may be due to the following reasons: (1) higher temperature increased the microbial activity, thus promoting oxygen consumption in the water,30 which contributed to a lower DO at 30 °C than at other temperatures; (2) oxygen produced by photosynthesis can be released from plant roots and leaves,31 consequently rendering DO in increased amounts for all the selected temperatures. Most importantly, the growth rate of DO at 5 °C was calculated to be 24.93%, which was slightly lower than that at 20 °C, suggesting that low temperature did not dramatically impact R. rotundifolia.
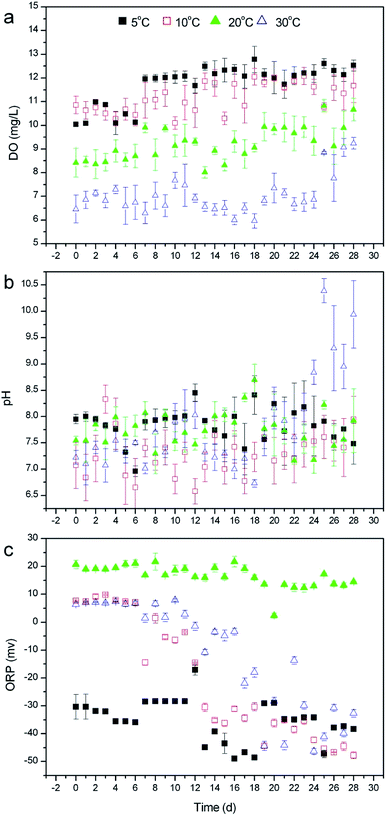 |
| Fig. 3 Changing trend of DO (a), pH (b) and ORP (c) in the overlying water of submersed R. rotundifolia at different temperatures. | |
Compared with DO, however, pH presented a totally distinct variation trend. As seen in Fig. 3b, the pH on day 28 was 7.48 at 5 °C, 7.95 at 10 °C, 7.92 at 20 °C and 9.94 at 30 °C, among which the pH at 5 °C dropped exclusively, whereas the pH at other temperatures increased and the most evident rise appeared at 30 °C. This result was most likely ascribed to the fact that a relatively low temperature generally generates a weak photosynthesis and a concurrently decreased CO2 consumption. In addition to DO and pH, ORP was also monitored throughout experiment. The ORPs for all selected temperatures clearly declined by the end of the operation (Fig. 3c), which is in good agreement with phytoremediation using vetiver for phenol degradation.28 As the previous literature describes,32 ORP is a vital indicator of the natural water condition, and a more negative ORP value corresponds to the better antioxidant properties of water. Accordingly, the ORP data in the current study implied that some reducing agents, like organic substances capable of being oxidized, might be produced during the cultivation of R. rotundifolia and released to the overlying water. Furthermore, there was a small decrease in the ORP at 20 °C, which probably resulted from a combination of oxygen and metabolite emission at this temperature.
3.3.2. Root vitality and POD activity. The root vitality under different temperatures is shown in Fig. 4a. At 5 °C, root vitality soared from the initial 0.26 to 1.5 mg g−1 h−1, whereas it fell by 38.66%, 28.74% and 5.15% when the temperature was 10, 20, and 30 °C, respectively. In comparison, the POD activity in Fig. 4b displayed a different trend, where it increased to a peak value of 445.40 U mg−1 on day 7 followed by decreasing gradually to 174 U mg−1 on day 28 at 5 °C, and it rose slowly to 255 U mg−1 on day 28 at 30 °C except for a sudden decline on day 14. It is well known that root vitality plays critical roles in the growth of plants since roots are multifunctional organs of adsorption, synthesis, assimilation, and transformation. Meanwhile, specific root temperature optima seem to exist, and increasing temperatures can induce an increased growth in many species.33 However, this appeared to be contradictory with R. rotundifolia as its root vitality at 5 °C was far higher than that at other temperatures (Fig. 4a), suggesting that the optimum root temperature of this species should be low. This conclusion can also be corroborated by the results presented in Fig. 4b, in which the highest POD activity occurred at 5 °C, revealing that cold temperatures improve the POD activities, thus contributing to the cold tolerance of R. rotundifolia to some extent. Notwithstanding, POD activities dropped rapidly later, indicating an adverse effect as a result of this temperature, which might be ascribed to the fact that low temperature affected RNA transcription and translation, reducing the synthesis of POD.17
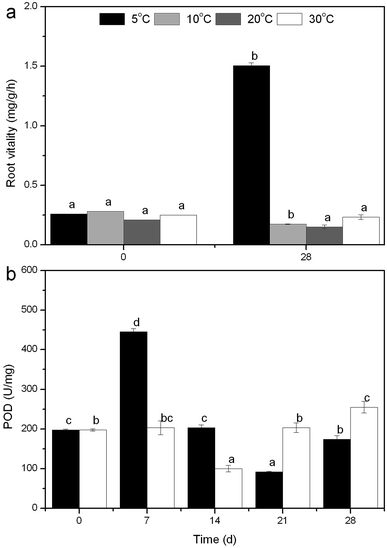 |
| Fig. 4 Root vitality (a) and POD activity (b) at different temperatures. | |
3.3.3. Soluble sugar and protein as well as MDA contents. Temperature was detrimental to the accumulation of soluble sugar and protein as well as MDA. At 5 °C, content went up to 5.5 mg g−1 on day 21 with a slight change on day 28 for soluble sugar (Fig. 5a), 437.9 mg g−1 on day 21 with a sharp decline on day 28 for soluble protein (Fig. 5b) and 10.1 mg g−1 on day 21 falling to 4.9 mg g−1 on day 28 for MDA (Fig. 5c). At 30 °C, soluble sugar and protein increased to 2.9 and 288.6 mg g−1 on day 14 followed by decreasing to 2.0 and 106.8 mg g−1 on day 28, respectively, while MDA rose to 6.8 mg g−1 and subsequently dropped rapidly to 0.8 on day 21 with nearly 1.8 mg g−1 on day 28. The complex essential role of soluble sugars in plant metabolism as products of hydrolytic processes, substrates in biosynthesis processes, and energy production, but also in sugar sensing and signaling systems, is well known. Furthermore, soluble sugars may also function as a typical osmoprotectant, stabilizing cellular membranes and maintaining turgor pressure,34 based on which more soluble sugar at 5 °C obtained under our experimental conditions was most likely used to protect cellular membranes since MDA contents were on the rise concurrently at this temperature. MDA is considered to be the final product of lipid peroxidation in the plant cell membrane22 and is referred to as an important indicator of membrane system injuries and cellular metabolism deterioration.35 Thereby, higher MDA contents (Fig. 5c) in this experiment indicated that cell membranes were injured by cold stress, but concomitantly this disadvantageous impact was alleviated by the enhanced soluble sugar to some degree. As for soluble protein, its reduction at high temperature might cause the decline of the photosynthetic rate and hasten leaf senescence,36 implying that a relatively low temperature was favorable for the physiological metabolism of R. rotundifolia.
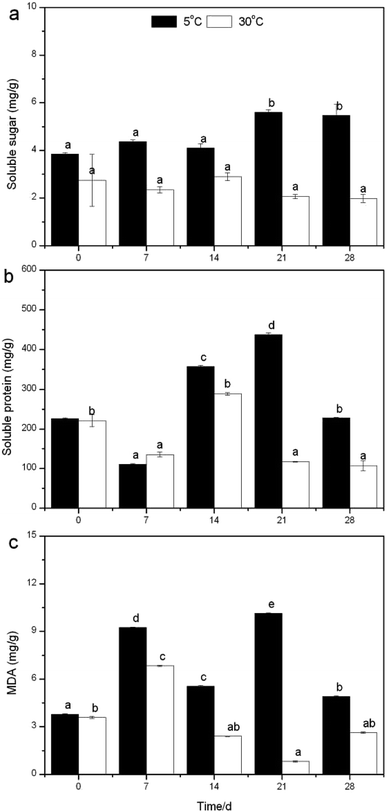 |
| Fig. 5 Changes of soluble sugar (a), protein (b), and MDA (c) contents at different temperatures. | |
3.3.4. Variation trend of chlorophyll. As R. rotundifolia grew, all chlorophyll contents were enhanced nearly linearly for both low and high temperatures. At 5 °C, chlorophyll a and b reached 8.4 and 4.4 mg g−1 on day 28, respectively, with the total chlorophyll (a plus b) at 12.4 mg g−1 (Fig. 6a). At 30 °C, content was 7.5 mg g−1 for chlorophyll a, 3.9 mg g−1 for chlorophyll b and 11.4 mg g−1 for the total chlorophyll (Fig. 6b). Chlorophyll is an extremely important biomolecule in photosynthesis with functions for light absorbance and light energy transformation.37 In general, low temperature inhibits chlorophyll accumulation in actively growing leaves to decrease the utilization of light, which would undoubtedly exert an adverse impact on the growth, thus further hindering nutrient uptake/removal. As shown in a prior report,17 the range of chlorophyll content in leaves of naked oats was 6.4–6.9 mg g−1 at room temperature, while the chlorophyll decreased greatly to 4.6 mg g−1 at low temperature. Compared to this plant, however, the chlorophyll content at 5 °C for R. rotundifolia were slightly more than those at 30 °C (Fig. 6), exhibiting the unique aspect of R. rotundifolia that can function well over a broader temperature range than other aquatic plants, which would ultimately make it a superior candidate for ecosystem remediation.
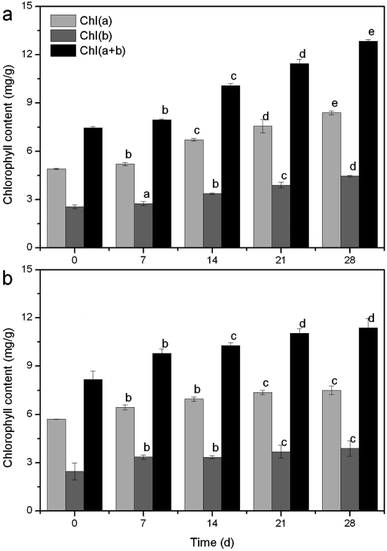 |
| Fig. 6 Variation trend of chlorophyll at 5 °C (a) and 30 °C (b). | |
Moreover, temperature is a major factor in plant growth and life history strategies.38 Most aquatic plant species exhibit optimal rates of photosynthesis at relatively high temperatures (between 20 and 35 °C).39 In a study of naturally occurring M. aquaticum populations, it was observed that seasonal water temperatures significantly influenced the total plant biomass, submersed shoot biomass, stolon biomass, and starch allocation patterns.40 Some of these results were equally verified in the present study. For example, stem weight (Fig. S5a†), blade number (Fig. S5c†) and leaf length (Fig. S6b†) had peak values at 30 °C, yet the highest fresh weight (Table S1†), tillers number (Table S2†) and leaf width (Fig. S6a†) rose at 10 °C and the greatest plant height (Fig. S5b†) appeared at 5 °C. Clearly, the growth properties further proved that R. rotundifolia could withstand a lower temperature than some other plants (Table S3†).
4. Conclusions
In this study, we investigated for the first time the nutrient removal and physiological changes of submersed R. rotundifolia in response to different temperatures. The REs of nutrients at low temperatures was comparable with those at normal temperatures. Root vitality, soluble substances (protein and sugar), MDA and chlorophyll presented the maximum value at low temperature. All the results demonstrated that R. rotundifolia is characterized by an excellent adaptation to a wide temperature range, and thus has potential for engineering applications, even at low temperatures.
Conflicts of interest
There are no conflicts to declare.
Acknowledgements
This research was supported by the Public Research Project of Zhejiang Province (LGF20E090001 and LGF19E090002) and the Basic Research Project of Wenzhou City (Z20160011).
References
- T. M. Arnold, R. C. Zimmerman, K. A. M. Engelhardt and J. C. Stevenson, Ecosystem Health and Sustainability, 2017, 3, 1353283 CrossRef.
- J. W. Miller, P. M. Kocovsky, D. Wiegmann and J. G. Miner, North American Journal of Fisheries Management, 2018, 38, 623–629 CrossRef.
- E. L. Hestir, D. H. Schoellhamer, J. Greenberg, T. Morgan-King and S. L. Ustin, Estuaries Coasts, 2016, 39, 1100–1112 CrossRef.
- M. J. Moreno, Water, Air, Soil Pollut., 2011, 214, 539–546 CrossRef CAS.
- W. Zou, L. Yuan and L. Zhang, Ecol. Eng., 2013, 57, 65–71 CrossRef.
- J. Luo, H. Duan, R. Ma, X. Jin, F. Li, W. Hu, K. Shi and W. Huang, Int. J. Appl. Earth Obs. Geoinf., 2017, 57, 154–165 CrossRef.
- Y. Pang, Y. Zhang, X. Yan and G. Ji, Environ. Sci. Technol., 2015, 49, 13550–13557 CrossRef CAS.
- J. Fan, J. Zhang, H. H. Ngo, W. Guo and X. Yin, Bioresour. Technol., 2016, 218, 1257–1260 CrossRef CAS.
- L. A. Gettys, W. O. Obando and F. C. Reed III, J. Aquat. Plant Manage., 2015, 53, 220–223 Search PubMed.
- H. Venkatasamy, A. Arivarasan, P. Raman, J. Jaidev, D. Thiyagarajan and B. Samabandam, Adv. Sci., Eng. Med., 2016, 8, 947–953 CrossRef CAS.
- L.-J. Zhang, S.-F. Yeh, Y.-T. Yu, L.-M. Y. Kuo and Y.-H. Kuo, Journal of Traditional and Complementary Medicine, 2011, 1, 57–63 CrossRef.
- D. Marbaniang and S. S. Chaturvedi, International Journal of Scientific and Research Publications, 2014, 4, 1–8 Search PubMed.
- D. Marbaniang and S. S. Chaturvedi, Journal of Sustainable Environmental Research, 2013, 2, 81–90 Search PubMed.
- K. C. Burks, D. W. Hall, V. V. Vandiver and C. C. Jacono, SIDA, Contributions to Botany, 2003, 20, 1765–1769 Search PubMed.
- C. Gu, F. Li, J. Xiao, S. Chu, S. Song and M. H. Wong, Sci. Rep., 2019, 9, 1–9 CrossRef.
- A. Baxter, R. Mittler and N. Suzuki, J. Exp. Bot., 2014, 65, 1229–1240 CrossRef CAS.
- W. Liu, K. Yu, T. He, F. Li, D. Zhang and J. Liu, Sci. World J., 2013, 2013, 1–7 Search PubMed.
- APHA, Standard Methods for the Examination of Water and Wastewater, American Public Health Association, Washington, DC, 1998 Search PubMed.
- X. Wang, X. Li and Y. Li, Biotechnol. Lett., 2007, 29, 1599–1603 CrossRef CAS.
- J. W. MacAdam, R. E. Sharp and C. J. Nelson, Plant Physiol., 1992, 99, 879 CrossRef CAS.
- P. L. Steponkus and F. O. Lanphear, Plant Physiol., 1967, 42, 1423 CrossRef CAS.
- D. M. Hodges, J. M. DeLong, C. F. Forney and R. K. Prange, Planta, 1999, 207, 604–611 CrossRef CAS.
- Y. Wang and S. Hu, Journal of Jinggangshan University (Natural Science), 2013, 34, 37–40 Search PubMed.
- J. Xiao, S. Chu, G. Tian, R. W. Thring and L. Cui, J. Hazard. Mater., 2016, 320, 564–570 CrossRef CAS.
- M. H. Hu, Y. S. Ao, X. E. Yang and T. Q. Li, Agric. Water Manage., 2008, 95, 607–615 CrossRef.
- C. C. Tanner, J. P. S. Sukias and M. P. Upsdell, Water Sci. Technol., 1999, 40, 147–154 CrossRef CAS.
- T. Phenrat, P. Teeratitayangkul, I. Prasertsung, R. Parichatprecha, P. Jitsangiam, N. Chomchalow and S. Wichai, Environ. Sci. Pollut. Res., 2017, 24, 13235–13246 CrossRef CAS.
- P. Teeratitayangkul, C. Phutthasimma, S. Wichai and T. Phenrat, Desalin. Water Treat., 2019, 159, 40–52 CrossRef CAS.
- S. L. Wood, E. F. Wheeler, R. D. Berghage and R. E. Graves, Trans. ASAE, 1999, 42, 185–190 CAS.
- P. Carvalho, S. M. Thomaz and L. M. Bini, Braz. J. Microbiol., 2005, 65, 51–60 CAS.
- H. Liu, Z. Hu, J. Zhang, H. H. Ngo, W. Guo, S. Liang, J. Fan, S. Lu and H. Wu, Bioresour. Technol., 2016, 214, 797–805 CrossRef CAS.
- V. V. Goncharuk, V. A. Bagrii, L. A. Mel'nik, R. D. Chebotareva and S. Y. Bashtan, J. Water Chem. Technol., 2010, 32, 1–9 CrossRef.
- H. Lyr, Ann. For. Sci., 1996, 53, 317–323 CrossRef.
- A. Mostajeran and V. Rahimi-Eichi, Agric. Environ. Sci., 2009, 5, 264–272 CAS.
- W. Fan, M. Zhang, H. Zhang and P. Zhang, PLoS One, 2012, 7, e37344 CrossRef CAS.
- H. Zhao, T. Dai, Q. Jing, D. Jiang and W. Cao, Plant Growth Regul., 2007, 51, 149–158 CrossRef CAS.
- W. Xu, D. T. Rosenow and H. T. Nguyen, Plant Breed., 2000, 119, 365–367 CrossRef CAS.
- R. M. Wersal and J. D. Madsen, J. Freshwater Ecol., 2013, 28, 147–164 CrossRef.
- L. Santamaría and W. Van Vierssen, Aquat. Bot., 1997, 58, 135–150 CrossRef.
- R. M. Wersal and J. D. Madsen, Weed Res., 2011, 51, 386–393 CrossRef.
Footnote |
† Electronic supplementary information (ESI) available. See DOI: 10.1039/d0ra03405c |
|
This journal is © The Royal Society of Chemistry 2020 |