DOI:
10.1039/D0RA02380A
(Paper)
RSC Adv., 2020,
10, 15171-15178
Fabrication of magnetic iron oxide-supported copper oxide nanoparticles (Fe3O4/CuO): modified screen-printed electrode for electrochemical studies and detection of desipramine
Received
14th March 2020
, Accepted 2nd April 2020
First published on 17th April 2020
Abstract
The present investigation examines a sensitive electrochemical technique to detect desipramine through Fe3O4/CuO nanoparticles (NPs). Fe3O4/CuO NPs were synthesized via a coprecipitation procedure, and the products were characterized via energy disperse spectroscopy, X-ray diffraction, transmission electron microscopy, scanning electron microscopy, and vibrating sample magnetometer. The voltage–current curve and differential pulse voltammetry examinations of Fe3O4/CuO-modified screen-printed electrode (Fe3O4/CuO/SPE) were followed by the determination of electro-catalytic activities toward desipramine oxidation in a phosphate buffer solution (pH = 7.0). In addition, the value of diffusion coefficient (D = 3.0 × 10−6 cm2 s−1) for desipramine was calculated. Then, based on the optimum conditions, it was observed that the currents of the oxidation peak were linearly proportionate to the concentration of desipramine in the broad range between 0.08 and 400.0 μM and LOD of 0.03 μM (S/N = 3). Finally, our new sensor was successfully utilized to detect desipramine in the real samples, with reasonable recovery in the range of 97.2% to 102.7%.
Introduction
Currently, researchers have attempted to construct electrochemical sensors because they have properties such as selectivity, sensitivity, rapid responses, specificity, and simplified fabrication. In fact, electrochemical sensors contribute vitally to the medicine analyses.1–7 In electrochemical analysis, the key component is electrode modification, which is one of the reasonable and robust strategies to resolve the limitations of the unmodified electrode, including lower selectivity, sensitivity, and stability as well as the blockade of electron transfer. Thus, experts in the field used diverse electroactive compositions to modify the surface.8–15
According to the studies, nano-technology is rapidly progressing towards the industrial applications in the areas of catalysts, isolation, synthesis, luminescence, and sensing.16,17 Owing to their specific physicochemical features such as higher surface area and better sensitivity, nanomaterials have been considered for CL reactions as the catalyst, energy acceptor, enhancer, and platform of the CL resonance energy transfer.18,19
Current authors reported that nanoparticles (NPs) are largely involved in the quality of the sensing tools. According to the voltammetric examinations, they utilized such particles as the electrode modifiers to improve special surface areas, mass transport, and catalytic efficiency.20–27
Based on the investigations, the magnetic particles of the nano-metric size, particularly the iron oxide (Fe3O4) NPs, have been considered because of the respective super-paramagnetic characteristics; however, their utilization is restricted owing to the larger ratio of surface to volume and robust dipole–dipole attraction between the particles, as magnetic features of Fe3O4 are poor, and the functional groups are restricted for selective bindings. Of course, researchers applied substances such as metals and metal oxides to modify the Fe3O4 NP surface for avoiding the self-aggregation and improving chemical stability. However, for their specific and reasonable features, researchers considerably attended the metal oxides NPs. In fact, Fe3O4/CuO NPs have a widespread utilization to modify the electrodes since they augment the electrical conductivity and electrodes surface. Moreover, they enhance the electron transfer kinetics between the surface of the electrode and a wider range of the electro-active samples.28–32
Researchers believe that SPEs are a very beneficial means to design disposable sensors for applying in the electro-analysis. Actually, SPEs are very flexible, can be easily used, are affordable analytical instruments, and finally are appropriate for miniaturization.33–37 Thus, Au, Hg, and Bi films are used to coat SPEs for improving the respective electrochemical functions.
Some studies illustrated that tricyclic anti-depressants such as desipramine have a mechanism at the presynaptic level in obstructing the reuptake of the monoamines, in particular, dopamine, norepinephrine, and serotonin. It has also been found that the post-synaptic activities involve obstructing the muscarinic receptors (cholinergic), alpha-2, histamine type-1, beta-adrenergic, and anti-depressant effects. However, they suffer from some complications. In particular, the therapeutic range for desipramine lies between 115–250 ng mL−1, whereas the toxic dose starts above 500 ng mL−1, and the plasma half-life is 12–28 hours.38 Numerous papers published correlation of the tricyclic anti-depressant overdose and severe body dysfunctions such as sleepiness, seizure, respiratory issues, and coma. Therefore, the medicine dose in the organism should be highly monitored.39,40
It is notable that the most utilized analytical procedures to detect desipramine relied on gas and liquid chromatography,41,42 electrophoresis,43 and spectrophotometry.44 However, detecting desipramine via the above methods showed higher sensitivity. However, they require costly tools, laborious pretreatment phases, trained operators, and multiple organic solvents. In addition, it is not possible to use the mentioned procedures for in situ assays. In this way, the methodologies using the electrochemical techniques are interesting because of their rapidness, good sensitivity, and selectivity.45–48
Herein, the procurement and utilization of a novel Fe3O4/CuO/SPE for the determination of desipramine are described. Under optimum conditions, it was found that the modified electrode had higher sensitivity, lower LOD, and wider linear dynamic range compared to the bare electrode. Moreover, the analytical applicability of the modified sensor in quantifying desipramine in the real samples was evaluated with satisfactory results.
Experimental
Chemicals and apparatus
We conducted the electrochemical tests using an Autolab potentiostat/galvanostat (PGSTAT 302N, Eco Chemie: the Netherlands), and then, we monitored the system by a general-purpose electrochemical system software.
It should be noted that SPE (DropSens: DRP-110, Spain) has 3 traditional electrodes called the graphite counter electrode, an unmodified graphite working electrode, and a silver pseudo-reference electrode. Moreover, we used a Metrohm 710 pH-meter for measuring the pH.
Desipramine and all the available reagents used were of analytical grade. We chose Merck (Darmstadt: Germany) for purchasing the reagents. Thus, in order to procure the buffers, we applied the ortho-phosphoric acid and the respective salts for providing pH ranges between 2.0 and 9.0.
Preparation of magnetic iron oxide-supported copper oxide NPs (Fe3O4/CuO)
First, the magnetic NP of Fe3O4 was synthesized by an easy coprecipitation modified technique45 using a solution of FeCl2·4H2O (10 mmol) and FeCl3·6H2O (20 mmol) in 120 mL deionized water, and the solution was placed under nitrogen gas. The solution was stirred for 30 minutes, and at this time, 10 mL of NH4OH (8 M) was added drop by drop into the solution. The black resulting solids were separated by an exterior magnetic field. Subsequently, the deionized water was used to wash it numerous times and dried for 4 h at 80 °C (eqn (1)). |
2Fe3+ + Fe2+ + 8OH− → Fe3O4 + 4H2O
| (1) |
In the second section of this work, we synthesized magnetic iron oxide-supported copper oxide NPs using the layer to layer method. 0.5 g of nano-sized Fe3O4 was added to 25 mL of thioglycolic acid (10 mM) under shaking for 1 h at room temperature. Then, the product was gathered by an exterior magnetic field, followed by washing the product with ethanol and deionized water two times. The functionalized Fe3O4 core, synthesized as described above, was then dispersed in 45 mL of Cu(NO3)2 (10 mM) for 20 minutes under ultrasonication until a uniform solution was obtained. Then, the precipitate obtained was collected with an external magnetic field and was washed two times by ethanol. To the obtained and washed precipitates, 25 mL of thioglycolic acid solution (10 mM) was added and stirred for 30 minutes at 60 °C. Then, the magnetic particles were isolated with an exterior magnetic field and were washed with ethanol. These cycles were repeated for 30 times, and then the samples were washed two times with ethanol, with the finally obtained sample being dried under vacuum for 6 h at 170 °C.
Preparing the electrode
Based on the easy process presented below, the bare SPE was coated. 1 mg of Fe3O4/CuO NPs was dispersed in 1 mL of an aqueous solution via ultra-sonication with an interval of 45 minutes. Afterward, 3 μL of the procured suspension was dropped onto the carbon working electrode surface. Finally, it was dried at room temperature.
Preparation of real samples
Desipramine tablets (Ruzdarou, Iran [labelled value desipramine = 25 mgper tablet]) were purchased. The desipramine pills were thoroughly powdered and homogenized prior to the preparation of 20 mL of the 0.1 M stock solution. In fact, we sonicated the solution for assuring an ideal dissolution. Then, we filtered the solution. Afterward, we poured the determined volume of the transparent filtrate into the electrochemical cell consisting of 10 mL of 0.1 M PBS (pH = 7) for recording the DPV voltammogram.
In addition, we kept the urine specimens inside a refrigerator immediately after being collected. Then, 10 mL of the specimens was centrifuged at 2000 rpm for fifteen minutes. In the next stage, we filtered the supernatant through a 0.45 μm filter. Afterward, distinct contents of the solution were transported into a 25 mL volumetric flask, and the solution was diluted to the mark with PBS (pH = 7.0). Then, diverse amounts of desipramine were used to spike the diluted urine specimens. Finally, desipramine contents were examined by our technique via the standard addition procedure.
Electrochemical measurements
Desipramine detection was performed using the CV and DPV methods, with a potentiostat/galvanostat Autolab PGSTAT 302N with GPES software. A graphite electrode (modified with magnetic iron oxide-supported copper oxide NPs (Fe3O4/CuO)) was used as the working electrode, together with a graphite counter electrode, and a silver pseudo-reference electrode. CV and DPV were carried out by repeated potential scanning in the range of 0.34 to 0.98 V at a scan rate of 50 mV s−1 in the presence of different concentrations of desipramine (0.08–400.0 μM) dissolved in 0.1 M PBS pH = 7.0. All electrochemical measurements were carried out at room temperature.
Results and discussion
Morphology and structure of magnetic Fe3O4/CuO
Fig. 1 shows magnetic Fe3O4/CuO, which were characterized by TEM. Fig. 2 shows the spherical morphology for this compound obtained by SEM. Fig. 3 shows the magnetic measurements of magnetic Fe3O4/CuO at room temperature. Due to the hysteresis loops, the saturation magnetization (MS) was found to be 60 emu g−1 for the above compound. Fig. 4 shows the EDX analyses of the as-synthesized NPs, which depicts the presence of the Fe, Cu, and O elements in this figure, clearly indicating the purity of the as-synthesized NPs.
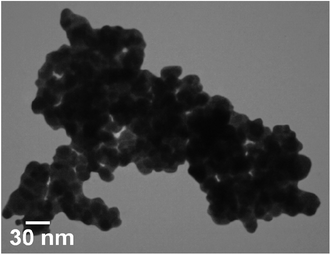 |
| Fig. 1 The TEM image of magnetic Fe3O4/CuO. | |
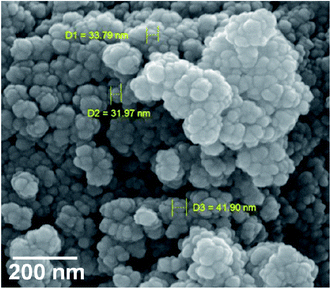 |
| Fig. 2 The SEM image of magnetic Fe3O4/CuO. | |
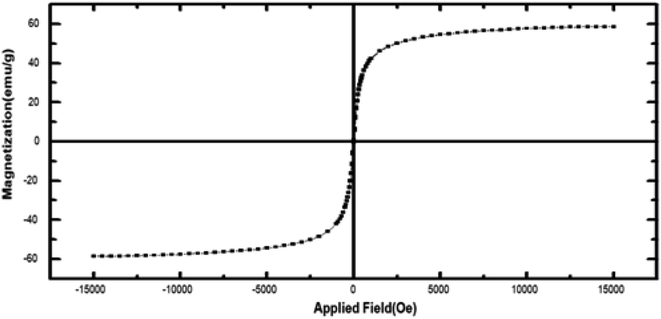 |
| Fig. 3 The VSM spectrum of magnetic Fe3O4/CuO. | |
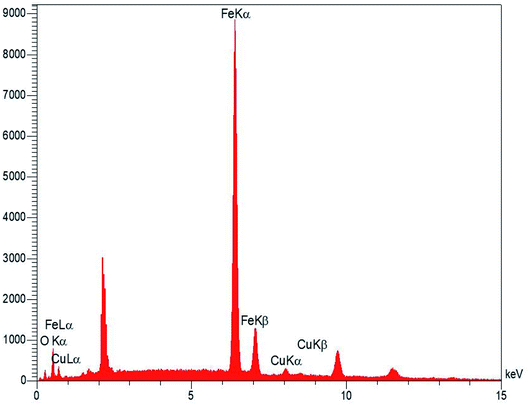 |
| Fig. 4 The EDS image of magnetic Fe3O4/CuO. | |
The XRD pattern in Fig. 5 confirmed the successful synthesis of the magnetic Fe3O4/CuO matched with the reference code: 00-025-0283 of the CuFe2O4 copper spinel form. By using the Scherer equation and 2θ, the mean size of the grain size for the magnetic Fe3O4/CuO (12 nm) can be obtained. Peaks for this compound were observed at 2θ = 18.64(111), 30.50(220), 35.89(400), 43.52(311), 53.97(511), 57.46(440), 63.11(533), and 71.70(444) planes, as shown in Fig. 5.46,47
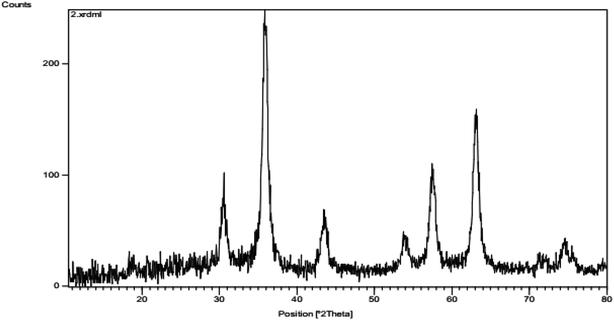 |
| Fig. 5 The XRD pattern of magnetic Fe3O4/CuO. | |
Electro-chemical behavior of desipramine on the Fe3O4/CuO/SPE
An optimum pH-value is required for studying the electrochemical behaviour of desipramine, which is pH-dependent so that we can obtain a specific output. However, we conducted the tests by employing the modified electrodes at distinct pH-values in the range between 2.0 and 9.0, with the occurrence of the most acceptable outputs for desipramine electro-oxidation at pH equal to 7.0.
Fig. 6 portrays the final cyclic voltammogram in the presence of 100.0 μM desipramine through the bare SPE (Curve a) and the Fe3O4/CuO/SPE (Curve b). As seen from the CV outputs, the greatest level of desipramine oxidation on the Fe3O4/CuO/SPE occurs at 670 mV, which is nearly 110 mV more negative in comparison to the unmodified SPE.
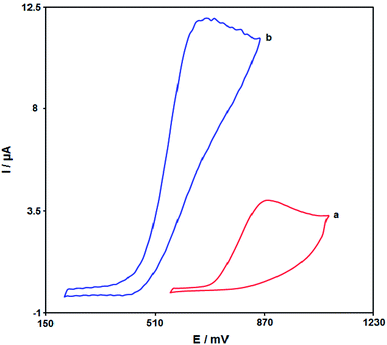 |
| Fig. 6 The cyclic voltammogram of (a) bare SPE and (b) Fe3O4/CuO/SPE in 0.1 M PBS (pH = 7.0) in the presence of 100.0 μM desipramine at the scan rate of 50 mV s−1. | |
Effects of the scan rate on the outputs
It is notable that the enhancement of the scan rate causes greater oxidation peak currents with regard to the outputs gained by examining the effects of the potential scan rates on desipramine oxidation current (Fig. 7). In addition, we found a linear association between Ip and the square root of the potential scan rate (ν1/2), indicating that diffusion controls the desipramine oxidation process.
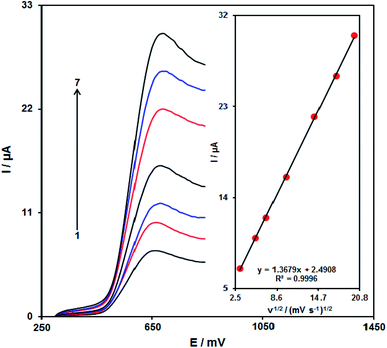 |
| Fig. 7 Linear sweep voltammogram of Fe3O4/CuO/SPE in 0.1 M PBS (pH = 7.0) consisting of 100.0 μM desipramine at diverse scan rates. Numbering 1–7 refer to 10, 30, 50, 100, 200, 300, and 400 mV s−1, respectively. Inset: variation of anodic peak current vs. ν1/2. | |
Chronoamperometric analysis
Analysis of chrono-amperometry for desipramine samples was done using the Fe3O4/CuO/SPE at 0.72 V. Fig. 8 represents the chronoamperometric results of the distinct concentrations of desipramine samples in PBS (pH = 7.0). Here, the Cottrell equation for analysing the chronoamperometry of the electro-active moiety under the mass transfer-limited condition will be presented as
where D refers to the diffusion coefficient (cm2 s−1), and Cb is the applied bulk concentration (mol cm−3). Fig. 8A depicts the experimental outputs of I versus t−1/2 so that the best fits were obtained for distinct concentrations of desipramine. Moreover, Fig. 8B is a representation of the resultant slopes relative to the straight line in Fig. 8A versus desipramine concentration. In addition, the mean value of D was calculated to be 3.0 × 10−6 cm2 s−1 based on the Cottrell equation and the final slope.
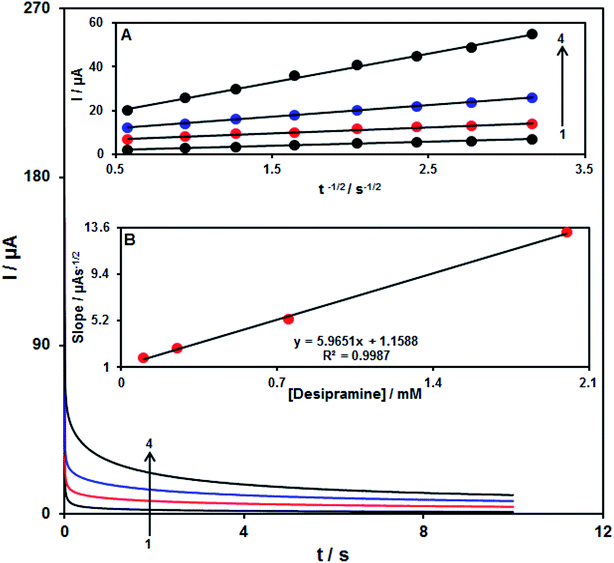 |
| Fig. 8 Chrono-amperograms achieved at Fe3O4/CuO/SPE in 0.1 M PBS (pH = 7.0) for distinct concentration of desipramine. Numbering 1–4 refer to 0.1, 0.25, 0.75, and 2.0 mM of desipramine, respectively. Insets: (A) I plot vs. t−1/2 achieved from chrono-amperograms 1 to 4. (B) The slope plot of straight lines versus desipramine concentration. | |
Calibration curve
With regard to the desipramine resultant peak currents through Fe3O4/CuO/SPE, desipramine was quantitatively analyzed in the water solution (Fig. 9). We also utilized the modified electrode (Fe3O4/CuO/SPE) as the working electrode within a range of desipramine. DPV, because of its benefits like greater sensitivity and more reasonable functions in the analytical utilizations, was used. However, the outputs implied the linear relationship between the peak current and desipramine concentrations in the concentration range between 0.08 and 400.0 μM. Finally, LOD was calculated to be 0.03 μM. These values were comparable to the values reported by other research groups for the determination of desipramine (Table 1). As can be seen in some cases that the linear dynamic range in the case of our method was better than other cases, and in some cases, the detection limit of our work was better than other works.
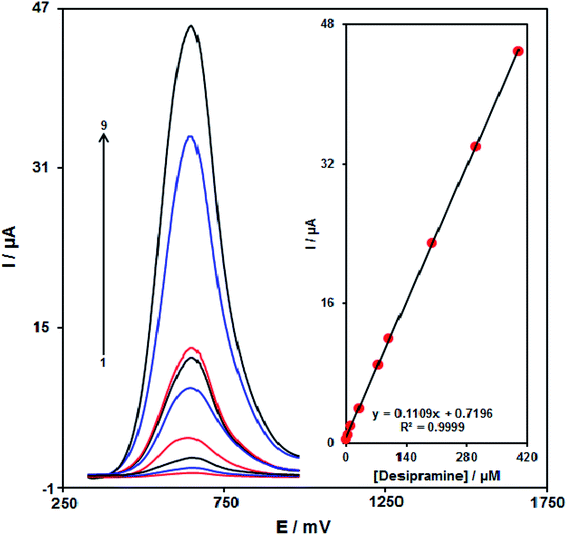 |
| Fig. 9 Differential pulse voltammograms of Fe3O4/CuO/SPE in 0.1 M PBS (pH = 7.0) consisting of distinct concentrations of desipramine. Numbering 1–9 refer to 0.08, 3.0, 10.0, 30.0, 75.0, 100.0, 200.0, 300.0, and 400.0 μM of desipramine, respectively. Inset: the peak current plot as a function of desipramine concentrations ranging between 0.08 and 400.0 μM. | |
Table 1 Comparison of the efficiency of some methods used in the detection of desipramine
Method |
Modifier |
LOD |
LDR |
Ref. |
High performance liquid chromatography |
— |
1.0 ng ml |
15.0–400.0 ng ml |
41 |
High performance liquid chromatography |
— |
0.03 μg L−1 |
0.05–0.684 μg L−1 |
48 |
Voltammetry |
Reduced graphene oxide |
1.04 nM |
0.3–2.5 μM |
49 |
Voltammetry |
Poly(methyl methacrylate) |
— |
0.01–0.1 μM |
50 |
Potentiometry |
N-(1-Naphthyl)ethylenediamine dihydrochloride-tetraphenyl borate |
0.09 |
1–10 000 |
51 |
Voltammetry |
Fe3O4/CuO NPs |
0.03 μM |
0.08–400.0 μM |
This work |
Generalizability and stability of Fe3O4/CuO/SPE
We kept our sensor at pH equal to 7.0 in PBS for 17 days in order to test the Fe3O4/CuO/SPE stability. Afterward, we recorded the cyclic voltammogram of the solution consisting of 35.0 μM of desipramine in order to compare it with the cyclic voltammogram achieved prior to immerging. According to the analyses, the desipramine oxidation peak did not change, and the current exhibited ∼2.2% reduction in the signals as compared with the early responses, proposing that Fe3O4/CuO/SPE enjoys acceptable stability.
It is notable that the CV was used to determine the antifouling property of the modified SPE towards the oxidation of desipramine and their products for the modified SPE prior to and following the utilization in the presence of desipramine. In addition, we registered the cyclic voltammograms in the presence of desipramine after 15 times cycling of potential of 50 mV s−1. The analysis showed a decline in the currents by ∼2.1%, with no alteration in the peak potential.
Analysis of real samples
We used desipramine in the desipramine tablet and the urine samples via the above procedure in order to assess the proposed modified electrode utility to detect the real samples. Therefore, the standard addition procedure was utilized. Table 2 reports the analysis outputs. Correspondingly, the outputs obtained for desipramine recovery were very good so that they could be generalized using the mean relative standard deviation.
Table 2 The application of Fe3O4/CuO/SPE for the determination of desipramine in desipramine tablet and urine samples (n = 5). All concentrations are in μM
Sample |
Spiked |
Found |
Recovery (%) |
R.S.D. (%) |
Desipramine tablet |
0 |
4.5 |
— |
3.1 |
3.0 |
7.7 |
102.7 |
2.4 |
8.0 |
12.4 |
99.2 |
2.9 |
13.0 |
17.6 |
100.6 |
3.1 |
18.0 |
22.2 |
98.7 |
1.8 |
Urine |
— |
— |
— |
— |
6.0 |
5.9 |
98.3 |
2.7 |
12.0 |
12.3 |
102.5 |
3.5 |
18.0 |
17.5 |
97.2 |
1.9 |
24.0 |
24.3 |
101.2 |
2.8 |
Conclusions
In this report, Fe3O4/CuO was procured by a coprecipitation procedure. Moreover, the Fe3O4/CuO generation was confirmed by the EDS analysis, XRD, SEM, TEM, and VSM. Further, the as-prepared Fe3O4/CuO/SPE was followed by reasonable electro-catalytic abilities to detect desipramine. Based on the results, our new Fe3O4/CuO/SPE sensor possesses very good analytical functions with multiple beneficial features, like a wider linear range (0.08–400.0 μM), low limit of detection (0.03 μM), better storage, and stability. Additionally, the urine specimen and desipramine tablet were used to assess the functional utility of the sensor, which shows very acceptable results and make Fe3O4/CuO one of the hopeful electrocatalysts to detect desipramine in the drug and biological specimens.
Conflicts of interest
All the authors declare no conflict of interest.
Acknowledgements
The authors acknowledge the financial support provided for this project (Project No. 98000791) by Neuroscience Research Center, Kerman University of Medical Sciences, Kerman, Iran. This research was supported by the Future Material Discovery Program (2016M3D1A1027666), Basic Science Research Program (2017R1A2B3009135) through the National Research Foundation of Korea, and China Scholarship Council (201808260042) is appreciated.
References
- M. Mazloum-Ardakani, H. Beitollahi, M. K. Amini, F. Mirkhalaf, B. F. Mirjalili and A. Akbari, Analyst, 2011, 136, 1965–1970 RSC.
- X. Yang, M. Gao, H. Hu and H. Zhang, Phytochem. Anal., 2011, 22, 291–295 CrossRef CAS PubMed.
- N. Rabiee, M. Safarkhani and M. Rabiee, Asian J. Nanosci. Mater., 2018, 1, 63–73 Search PubMed.
- H. Karimi-Maleh and O. A. Arotiba, J. Colloid Interface Sci., 2020, 560, 208–212 CrossRef CAS PubMed.
- D. Sun, F. Wang, K. Wu, J. Chen and Y. Zhou, Microchim. Acta, 2009, 167, 35–39 CrossRef CAS.
- J. B. Raoof, N. Teymoori, M. A. Khalilzadeh and R. Ojani, Mater. Sci. Eng., C, 2015, 47, 77–84 CrossRef CAS PubMed.
- M. A. Khalilzadeh, S. Tajik, H. Beitollahi and R. A. Venditti, Ind. Eng. Chem. Res., 2020, 59, 4219–4228 CrossRef CAS.
- M. A. Khalilzadeh and M. Borzoo, J. Food Drug Anal., 2016, 24, 796–803 CrossRef CAS PubMed.
- W. H. Elobeid and A. A. Elbashir, Prog. Chem. Biochem. Res., 2019, 2, 24–33 CrossRef.
- H. Beitollahi, S. Tajik, M. H. Asadi and P. Biparva, J. Anal. Sci. Technol., 2014, 5, 1–9 Search PubMed.
- M. Portaccio, D. Di Tuoro, F. Arduini, D. Moscone, M. Cammarota, D. G. Mita and M. Lepore, Electrochim. Acta, 2013, 109, 340–347 CrossRef CAS.
- M. Payehghadr, S. Adineh Salarvand, F. Nourifard, M. K. Rofouei and N. Bahramipanah, Adv. J. Chem., Sect. A, 2019, 2, 377–385 CAS.
- H. Karimi-Maleh, C. T. Fakude, N. Mabuba, G. M. Peleyeju and O. A. Arotiba, J. Colloid Interface Sci., 2019, 554, 603–610 CrossRef CAS PubMed.
- C. Rajkumar, B. Thirumalraj, S. M. Chen and S. Palanisamy, RSC Adv., 2016, 6, 68798–68805 RSC.
- S. Tajik, M. A. Taher, H. Beitollahi and M. Torkzadeh-Mahani, Talanta, 2015, 134, 60–64 CrossRef CAS PubMed.
- Nanomaterials and Nanochemistry, ed. C.B. Echignac, P. Houdy and M. Lahmani, Springer-Verlag, Berlin, Heidelberg, 2007 Search PubMed.
- H. Karimi-Maleh, P. Biparva and M. Hatami, Biosens. Bioelectron., 2013, 48, 270–275 CrossRef CAS PubMed.
- Y. Su, Y. Xie, X. Hou and Y. Lv, Appl. Spectrosc. Rev., 2014, 49, 201–232 CrossRef CAS.
- P. Biparva, S. M. Abedirad and S. Y. Kazemi, Talanta, 2014, 130, 116–121 CrossRef CAS PubMed.
- H. Beitollahi, M. A. Khalilzadeh, S. Tajik, M. Safaei, K. Zhang, H. W. Jang and M. Shokouhimehr, ACS Omega, 2020, 5, 2049–2059 CrossRef CAS PubMed.
- S. Tajik, H. Beitollahi and P. Biparva, J. Serb. Chem. Soc., 2018, 83, 863–874 CrossRef CAS.
- H. Beitollahi, M. Safaei and S. Tajik, Electroanalysis, 2019, 31, 1135–1140 CrossRef CAS.
- H. Karimi-Maleh, C. T. Fakude, N. Mabuba, G. M. Peleyeju and O. A. Arotiba, J. Colloid Interface Sci., 2019, 554, 603–610 CrossRef CAS PubMed.
- H. Ibrahim, Y. Temerk and N. Farhan, RSC Adv., 2016, 6, 90220–90231 RSC.
- H. Beitollahi, H. Karimi-Maleh and H. Khabazzadeh, Anal. Chem., 2008, 80, 9848–9851 CrossRef CAS PubMed.
- T. Pagar, S. Ghotekar, K. Pagar, S. Pansambal and R. Oza, J. Chem. Rev., 2019, 1, 260–270 Search PubMed.
- M. M. Motaghi, H. Beitollahi, S. Tajik and R. Hosseinzadeh, Int. J. Electrochem. Sci., 2016, 11, 7849–7860 CrossRef CAS.
- B. Fang, G. Wang, W. Zhang, M. Li and X. Kan, Electroanalysis, 2005, 17, 744–748 CrossRef CAS.
- H. Yin, Y. Zhou, X. Meng, T. Tang, S. Ai and L. Zhu, Food Chem., 2011, 127, 1348–1353 CrossRef CAS PubMed.
- L. Yang, X. Ren, F. Tang and L. Zhang, Biosens. Bioelectron., 2009, 25, 889–895 CrossRef CAS PubMed.
- J. Qiu, H. Peng and R. Liang, Electrochem. Commun., 2007, 9, 2734–2738 CrossRef CAS.
- C. Yu, J. Guo and H. Gu, Electroanalysis, 2010, 22, 1005–1011 CrossRef CAS.
- H. Beitollahi, H. Mahmoudi-Moghaddam and S. Tajik, Anal. Lett., 2019, 52, 1432–1444 CrossRef CAS.
- T. Dayakar, K. V. Rao, K. Bikshalu, V. Malapati and K. K. Sadasivuni, Biosens. Bioelectron., 2018, 111, 166–173 CrossRef CAS PubMed.
- B. Pérez-Fernández, D. Martín-Yerga and A. Costa-García, RSC Adv., 2016, 6, 83748–83757 RSC.
- M. Khairy, H. A. Ayoub and C. E. Banks, Food Chem., 2018, 255, 104–111 CrossRef CAS PubMed.
- M. R. Ganjali, Z. Dourandish, H. Beitollahi, S. Tajik, L. Hajiaghababaei and B. Larijani, Int. J. Electrochem. Sci., 2018, 13, 2448–2461 CrossRef CAS.
- P. Knihnicki, M. Wieczorek, M. Bienias, R. Wietecha-Posłuszny, M. Woźniakiewicz and P. Kościelniak, Procedia Eng., 2012, 47, 1342 CrossRef CAS.
- M. W. Linder and P. E. Keck, Standards of laboratory practice: antidepressant drug monitoring, Clin. Chem., 1998, 44, 1073–1084 CAS.
- G. Sahin, B. Giray and P. Erkekoglu, Turk. Klin. Tip Bilimleri Derg., 2008, 28, 533–540 CAS.
- A. Onal and A. Oztunc, Rev. Anal. Chem., 2011, 30, 165–171 CAS.
- R. de la Torre, J. Ortuno, J. A. Pascual, S. Gonzalez and J. Ballesta, Ther. Drug Monit., 1998, 20, 340–346 CrossRef CAS PubMed.
- M. I. Acedo-Valenzuela, N. Mora-Diez, T. Galeano-Diaz and A. Silva-Rodriguez, Anal. Sci., 2010, 26, 699–702 CrossRef CAS PubMed.
- H. N. Deepakumari, M. K. Prashanth, B. C. V. Kumar and H. D. Revanasiddappa, J. Appl. Spectrosc., 2015, 81, 1004–1011 CrossRef CAS.
- Y. Wei, B. Han, X. Hu, Y. Lin, X. Wang and X. Deng, Procedia Eng., 2012, 27, 632–637 CrossRef CAS.
- P. Scherrer, Göttinger Nachrichten Gesell., 1918, 2, 98–100 Search PubMed.
- A. Patterson, Phys. Rev., 1939, 56, 978–982 CrossRef CAS.
- M. Wozniakiewicz, J. Kuczara and P. Koscielniak, Probl. Forensic Sci., 2007, 69, 90–97 CAS.
- F. H. Cincotto, D. L. C. Golinelli, S. A. S. Machado and F. C. Moraes, Sens. Actuators, B, 2017, 239, 488–493 CrossRef CAS.
- P. Knihnicki, M. Wieczorek, A. Moos, P. Kościelniak, R. Wietecha-Posłuszny and M. Woźniakiewicz, Sens. Actuators, B, 2013, 189, 37–42 CrossRef CAS.
- A. A. Ensafi and A. R. Allafchian, IEEE Sens. J., 2011, 11, 2576–2582 CAS.
|
This journal is © The Royal Society of Chemistry 2020 |