DOI:
10.1039/D0RA01830A
(Paper)
RSC Adv., 2020,
10, 11750-11754
Access to N-unprotected 2-amide-substituted indoles from Ugi adducts via palladium-catalyzed intramolecular cyclization of o-iodoanilines bearing furan rings†
Received
17th January 2020
, Accepted 28th February 2020
First published on 23rd March 2020
Abstract
A variety of N-unprotected 2-amide-substituted indoles were synthesized from readily available furfural-based Ugi adducts in moderate to good yields via palladium-catalyzed intramolecular cyclization of o-iodoanilines bearing furan rings. These reactions involved a cascade sequence consisting of dearomatizing arylation, opening of the furan ring, and deprotection of the N atom.
Polyfunctionalized indoles, including 2-amide-substituted indoles, are privileged motifs in medicinal chemistry and synthetic organic chemistry.1 The indole ring is probably the most common heterocycle found in natural products and pharmaceuticals,2 and functionalized indoles are versatile building blocks for the preparation of structurally complex and novel indolines, many of which show potent bioactivities (Fig. 1).3 Thus, much effort has been devoted to the development of strategies for the synthesis and functionalization of indoles and their derivatives.4 Among them, the most attractive routes are those involving transition-metal-catalyzed intermolecular or intramolecular cyclization of o-haloanilines with alkenes,5 alkynes,6 or allenes.7 Despite the attractiveness of these routes, it would be desirable to develop efficient catalytic methods for the preparation of functionalized indoles from o-haloanilines and furans, which are readily available, alternatives to alkenes for diversity-oriented synthesis strategies.8,9
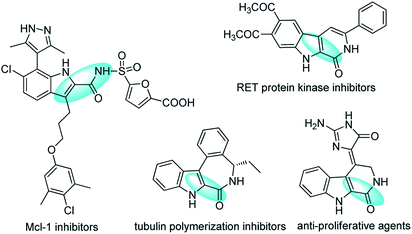 |
| Fig. 1 Bioactive 2-amide-substituted indoles. | |
We speculated that Ugi adducts might be useful for this purpose. Ugi reactions involve four components—an aldehyde or ketone, an isocyanide, an amine, and a carboxylic acid—and afford a diverse array of functionalized α-acylamino amides,10 which can be subjected to a wide variety of postcondensation transformations to achieve further structural diversity.11 Recently, we and other groups developed a route to functionalized indoles via palladium-catalyzed intramolecular arylative dearomatization of 2-bromo-N-(furan-2-ylmethyl)anilines.5f,12 In this paper, we report a convenient protocol for the synthesis of α-amide-substituted indoles via palladium-catalyzed intramolecular arylative cyclization of furans that were generated by Ugi reactions of furfurals and o-haloanilines (Scheme 1).
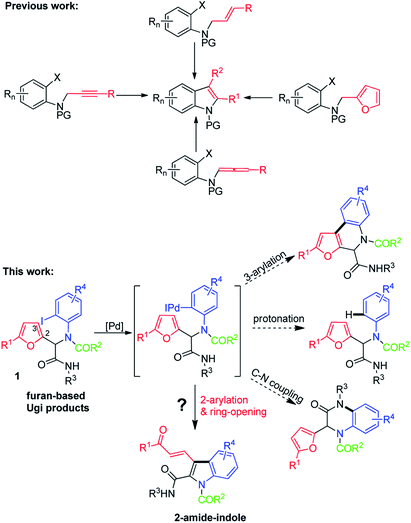 |
| Scheme 1 Pd-catalyzed approaches to polyfunctionalized indoles from o-haloanilines. | |
The success of this protocol relies on suppression of the following side reactions: β-arylation of the furan ring, protonation of the ArI, and intramolecular C–N coupling. With this in mind, we chose N-(tert-butyl)-2-(furan-2-yl)-2-(N-(2-iodophenyl)acetamido)acetamide (1a)—which was prepared by means of a Ugi reaction of furfural, 2-iodoaniline, acetic acid, and tert-butyl isocyanide—as the substrate for optimization of the reaction conditions. We were pleased to find that upon treatment of 1a with Pd(PPh3)4 (0.05 equiv.), PPh3 (0.1 equiv.), and K2CO3 (2 equiv.) in 1,4-dioxane at 70 °C for 12 h, polysubstituted N-unprotected indole 2a was obtained in 30% yield along with unidentified by-products (Table 1, entry 1). This transformation clearly involved a cascade sequence consisting of arylation, ring-opening, and N-deacetylation. The in situ N-deacetylation is particularly interesting and useful and may have resulted from the weaker nucleophilicity of the N atom of the indole ring relative to that of the amide N of 1a. Other bases (Cs2CO3, NaHCO3, Na2CO3, and 1,8-diazabicyclo[5.4.0]undec-7-ene (DBU)) were also tested, but 2a was not detected in any of these reactions (entries 2–5). Stronger base of Cs2CO3 resulted in side-reaction of C–N coupling. NaHCO3 and Na2CO3 as the base mostly led to the protonated product. DBU led to no reaction. Next, we attempted to improve the yield of 2a by increasing the reaction temperature (entries 6–9), and an 89% yield was obtained at 110 °C. Screening of various ligands other than PPh3 failed to produce better results (entries 10–13), and Pd(PPh3)4 was the optimal catalyst (compare entry 2 with entries 14–17). Evaluation of other solvents (THF, toluene, and DMSO) did not improve the yield (entries 18–20). Therefore, we concluded that the optimal conditions involved the use of Pd(PPh3)4 (0.05 equiv.) as the catalyst, K2CO3 (2.0 equiv.) as the base, 1,4-dioxane as the solvent, and 110 °C as the reaction temperature.
Table 1 Optimization of reaction conditionsa
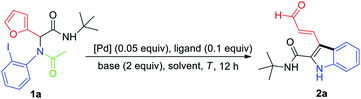
|
Entry |
[Pd] |
Ligand |
Base |
T (°C) |
Yieldb (%) |
Reaction conditions: 1a (0.2 mmol), catalyst (0.05 equiv.), ligand (0.1 equiv.), and base (2 equiv.) in 2.0 mL of 1,4-dioxane were allowed to react under nitrogen for 12 h. DBU, 1,8-diazabicyclo[5.4.0]undec-7-ene; DPPP, 1,3-bis(diphenylphosphino)propane; DPPB, 1,4-bis(diphenylphosphino)butane; DPPF, 1,1′-bis(diphenylphosphino)ferrocene; xantphos, 4,5-bis(diphenylphosphino)-9,9-dimethylxanthene. Yields were determined by 1H NMR spectroscopy. ND = not detected. THF was the solvent. Toluene was the solvent. DMSO was the solvent. |
1 |
Pd(PPh3)4 |
PPh3 |
K2CO3 |
70 |
30 |
2 |
Pd(PPh3)4 |
PPh3 |
Cs2CO3 |
70 |
ND |
3 |
Pd(PPh3)4 |
PPh3 |
NaHCO3 |
70 |
ND |
4 |
Pd(PPh3)4 |
PPh3 |
Na2CO3 |
70 |
ND |
5 |
Pd(PPh3)4 |
PPh3 |
DBU |
70 |
ND |
6 |
Pd(PPh3)4 |
PPh3 |
K2CO3 |
80 |
31 |
7 |
Pd(PPh3)4 |
PPh3 |
K2CO3 |
100 |
44 |
8 |
Pd(PPh3)4 |
PPh3 |
K2CO3 |
110 |
89 |
9 |
Pd(PPh3)4 |
PPh3 |
K2CO3 |
120 |
25 |
10 |
Pd(PPh3)4 |
DPPP |
K2CO3 |
110 |
18 |
11 |
Pd(PPh3)4 |
DPPB |
K2CO3 |
110 |
19 |
12 |
Pd(PPh3)4 |
DPPF |
K2CO3 |
110 |
12 |
13 |
Pd(PPh3)4 |
Xantphos |
K2CO3 |
110 |
48 |
14 |
Pd2(dba)3 |
PPh3 |
K2CO3 |
110 |
50 |
15 |
Pd(OAc)2 |
PPh3 |
K2CO3 |
110 |
18 |
16 |
Pd(PPh3)2Cl2 |
PPh3 |
K2CO3 |
110 |
54 |
17 |
Pd(CH3CN)2Cl2 |
PPh3 |
K2CO3 |
110 |
31 |
18c |
Pd(PPh3)4 |
PPh3 |
K2CO3 |
110 |
45 |
19d |
Pd(PPh3)4 |
PPh3 |
K2CO3 |
110 |
21 |
20e |
Pd(PPh3)4 |
PPh3 |
K2CO3 |
110 |
66 |
With the optimized conditions in hand, we prepared a series of Ugi adducts 1 with various R1–R4 groups and a furan moiety in moderate yields, and we subjected the resulting compounds to the arylative cyclization conditions to investigate the substrate scope (Table 2). In all cases, the reaction proceeded smoothly to afford corresponding indoles 2 in moderate to good isolated yields (40–77%). Specifically, with R1 = H, R2 = Me, and R4 = t-Bu, several R3 groups (H, Me, F, and Cl) were screened and found to provide corresponding indolyl aldehydes 2a–2d in 45–66% yields (entries 1–4). Reaction of 1c, which bears an electron-withdrawing 4-F group, gave a substantial amount of a by-product generated by protonation without opening of the furan ring, which resulted in a relatively low yield of 2c (45%). Similarly, with R1 = Me, R2 = Me, and R4 = t-Bu, compounds with H, Me, MeO, and CF3 at R3 afforded 2e–2h in 60–77% yields (entries 5–8). Substrate 1h, which has an electron-withdrawing 4-CF3 at R3, gave a lower yield (60%) than the other three substrates. In addition to H or Me, R1 could be Ph or 4-Me-Ph: 2i and 2j were obtained in 67% and 72% yields, respectively (entries 9 and 10). Notably, when R2 was an aryl group (4-MeO-Ph), 2e was produced in 77% yield (entry 11). In contrast, when R3 was n-Pr, the yield of 2e was only 40% (entry 12). Finally, when R4 was cyclohexyl, 2m–2o were obtained in good yields (entries 13–15).
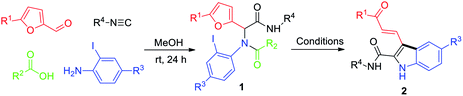
|
Entry |
R1 |
R2 |
R3 |
R4 |
1 (% yieldb) |
2 (% yieldb) |
Reaction conditions: 1 (0.2 mmol), catalyst (0.05 equiv.), ligand (0.1 equiv.), and base in 2.0 mL solvent were allowed to react at 110 °C for 12 h. Cy, cyclohexyl. Isolated yields are given. |
1 |
H |
Me |
H |
t-Bu |
1a (50) |
2a (66) |
2 |
H |
Me |
Me |
t-Bu |
1b (45) |
2b (63) |
3 |
H |
Me |
F |
t-Bu |
1c (52) |
2c (45) |
4 |
H |
Me |
Cl |
t-Bu |
1d (41) |
2d (64) |
5 |
Me |
Me |
H |
t-Bu |
1e (42) |
2e (77) |
6 |
Me |
Me |
Me |
t-Bu |
1f (42) |
2f (63) |
7 |
Me |
Me |
MeO |
t-Bu |
1g (40) |
2g (70) |
8 |
Me |
Me |
CF3 |
t-Bu |
1h (40) |
2h (60) |
9 |
Ph |
Me |
H |
t-Bu |
1i (46) |
2i (67) |
10 |
p-Tolyl |
Me |
H |
t-Bu |
1j (33) |
2j (72) |
11 |
Me |
PMB |
H |
t-Bu |
1k (55) |
2e (77) |
12 |
Me |
n-Pr |
H |
t-Bu |
1l (32) |
2e (40) |
13 |
Me |
Me |
H |
Cy |
1m (57) |
2m (50) |
14 |
Me |
Me |
MeO |
Cy |
1n (53) |
2n (61) |
15 |
Me |
Me |
CF3 |
Cy |
1o (42) |
2o (66) |
Products 2 bear amide, carbonyl and alkenyl functional groups, all of which are amenable to numerous further transformations that can be used to prepare structurally diverse indoles. For example, hydrogenation of the double bonds of 2e–2g and 2i afforded the corresponding products (3e–3g and 3i) in good yields (Scheme 2).
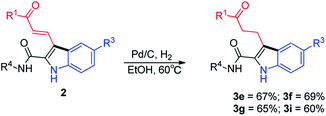 |
| Scheme 2 Hydrogenation of 2. | |
In Scheme 3, we depict two possible pathways for this transformation (electrophilic palladation and carbopalladation) on the basis of the above-described experimental results and previously reported results regarding arylation of furans.12,13 Specifically, an oxidative addition reaction between aryl iodide 1 and palladium(0) forms intermediate A. Intramolecular electrophilic palladation of the furan ring of A at the α-position results in the generation of intermediate B, which undergoes base-mediated furan ring-opening and β-elimination to afford intermediate C. A reductive elimination reaction of C provides F and palladium(0), completing the catalytic cycle. Deprotection of F yields 2. Alternatively, A undergoes carbopalladation to form intermediate D, which isomerizes to π-allylic palladium complex E. Ring-opening of E produces F.
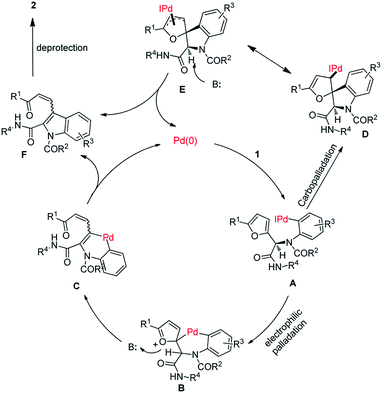 |
| Scheme 3 Possible pathway for the formation of 2. | |
Conclusions
In summary, we have developed a protocol for the synthesis of N-unprotected 2-amide-substituted indoles by means of Pd-catalyzed dearomatizing intramolecular arylation reactions of readily available furfural-based Ugi adducts. This protocol involves an intramolecular condensation of an o-haloaniline bearing a furan ring and a subsequent cascade involving dearomatizing arylation, opening of the furan ring, and N-deprotection. The bioactivities of the obtained polysubstituted indoles are being explored in our laboratory, and the results will be reported in due course.
Conflicts of interest
There are no conflicts to declare.
Acknowledgements
This work was supported by grants from the National Program on Key Research Project (no. 2016YFA0602900), the National Natural Science Foundation of China (no. 21871094), the Science and Technology Program of Guangzhou, China (no. 201707010057), Guangdong Natural Science Foundation (no. 2017A030312005), and the Science and Technology Planning Project of Guangdong Province, China (no. 2017A020216021).
Notes and references
-
(a) A. R. Katritzky and A. F. Pozharskii, Handbook of Heterocyclic Chemistry, Pergamon, Oxford, 2000, ch. 4 Search PubMed
;
(b) R. J. Sundberg, Indoles, Academic Press, San Diego, 1996 Search PubMed
. - For reviews on bioactive indoles:
(a) T. Eicher, S. Hauptmann and P. A. Speicher, The Chemistry of Heterocycles: Structure, Reactions, Syntheses, and Applications, Wiley-VCH: Veriag GmbH, 2nd edn, 2003 CrossRef
;
(b) L. F. Fu, Advances in the Total Syntheses of Complex Indole Natural Products, Springer-Verlag, Berlin, Heidelberg, 2010 Search PubMed
. - For selected reviews on using indoles as building blocks in organic synthesis:
(a) A. A. Festa, L. G. Voskressensky and E. V. Van der Eycken, Chem. Soc. Rev., 2019, 48, 4401–4423 RSC
;
(b) J. M. Saya, E. Ruijter and R. V. A. Orru, Chem.–Eur. J., 2019, 25, 8916–8935 CrossRef CAS PubMed
;
(c) Y. S. Wang, F. K. Xie, B. Lin, M. S. Cheng and Y. X. Liu, Chem.–Eur. J., 2018, 24, 14302–14315 CrossRef CAS PubMed
;
(d) J.-B. Chen and Y.-X. Jia, Org. Biomol. Chem., 2017, 15, 3550–3567 RSC
;
(e) W. W. Zi, Z. W. Zuo and D. W. Ma, Acc. Chem. Res., 2015, 48, 702–711 CrossRef CAS PubMed
;
(f) S. P. Roche, J.-J. Y. Tendoung and B. Treguier, Tetrahedron, 2015, 71, 3549–3591 CrossRef CAS
;
(g) L. M. Repka and S. E. Reisman, J. Org. Chem., 2013, 78, 12314–12320 CrossRef CAS PubMed
;
(h) D. Zhang, H. Song and A. Y. Qin, Acc. Chem. Res., 2011, 44, 447–457 CrossRef CAS PubMed
. - For recent reviews on the synthesis of indoles:
(a) X.-Y. Liu and Y. Qin, Acc. Chem. Res., 2019, 52, 1877–1891 CrossRef CAS PubMed
;
(b) K. Nagaraju and D. W. Ma, Chem. Soc. Rev., 2018, 47, 8018–8029 RSC
;
(c) L. L. Anderson, M. A. Kroc, T. W. Reidl and J. Son, J. Org. Chem., 2016, 81, 9521–9529 CrossRef CAS PubMed
;
(d) M. Platon, R. Amardeil, L. Djakovitchb and J.-C. Hierso, Chem. Soc. Rev., 2012, 41, 3929–3968 RSC
;
(e) M. Shiri, Chem. Rev., 2012, 112, 3508–3549 CrossRef CAS PubMed
. - For selected recent examples of condensation of o-haloanilines with alkenes to indoles:
(a) D. S. Chen, Y. Y. Chen, Z. L. Ma, L. Zou, J. Q. Li and Y. Liu, J. Org. Chem., 2018, 83, 6805–6814 CrossRef CAS PubMed
;
(b) S. J. Gharpure and D. Anuradha, Org. Lett., 2017, 19, 6136–6139 CrossRef CAS PubMed
;
(c) M. Paraja and C. Valdés, Chem. Commun., 2016, 52, 6312–6315 RSC
;
(d) A. P. Kale, G. S. Kumar and M. Kapur, Org. Biomol. Chem., 2015, 13, 10995–11002 RSC
;
(e) A. P. Kale, G. S. Kumar, A. R. K. Mangadan and M. Kapur, Org. Lett., 2015, 17, 1324–1327 CrossRef CAS PubMed
;
(f) B. L. Yin, C. B. Cai, G. H. Zeng, R. Q. Zhang, X. Li and H. F. Jiang, Org. Lett., 2012, 14, 1098–1101 CrossRef CAS PubMed
;
(g) T. Jensen, H. Pedersen, B. Bang-Andersen, R. Madsen and M. Jørgensen, Angew. Chem., Int. Ed., 2008, 47, 888–890 CrossRef CAS PubMed
. - For selected recent examples of condensation of o-haloanilines with alkynes to indoles:
(a) D. P. Chen, J. Z. Yao, L. L. Chen, L. F. Hu, X. F. Li and H. W. Zhou, Org. Chem. Front., 2019, 6, 1403–1408 RSC
;
(b) P. K. R. Panyam, R. Sreedharan and T. Gandhi, Org. Biomol. Chem., 2018, 16, 4357–4364 RSC
;
(c) P. K. R. Panyama and T. Gandhi, Adv. Synth. Catal., 2017, 359, 1144–1151 CrossRef
;
(d) T. A. Moss, A. S. Lister and J. Wang, Tetrahedron Lett., 2017, 58, 3136–3138 CrossRef CAS
;
(e) X. H. Pan, C. Y. Yang, J. L. Cleveland and T. D. Bannister, J. Org. Chem., 2016, 81, 2194–2200 CrossRef CAS PubMed
;
(f) K. V. Chuang, M. E. Kieffer and S. E. Reisman, Org. Lett., 2016, 18, 4750–4753 CrossRef CAS PubMed
;
(g) G. P. da Silva, A. Ali, R. C. da Silva, H. Jiang and M. W. Paixão, Chem. Commun., 2015, 51, 15110–15113 RSC
;
(h) H. C. Lin and U. Kazmaier, Eur. J. Org. Chem., 2009, 1221–1227 CrossRef CAS
. - For selected recent examples of condensation of o-haloanilines with allenes to indoles:
(a) Y. Higuchi, T. Mita and Y. Sato, Org. Lett., 2017, 19, 2710–2713 CrossRef CAS PubMed
;
(b) H. Iwasaki, K. Suzuki, M. Yamane, S. Yoshida, N. Kojima, M. Ozeki and M. Yamashita, Org. Biomol. Chem., 2014, 12, 6812–6815 RSC
;
(c) S. Z. He, R. P. Hsung, W. R. Presser, Z.-X. Ma and B. J. Haugen, Org. Lett., 2014, 16, 2180–2183 CrossRef CAS PubMed
;
(d) M.-G. Braun, M. H. Katcher and A. G. Doyle, Chem. Sci., 2013, 4, 1216–1220 RSC
. - For reviews on diversity-oriented synthesis:
(a) E. Lenci, G. Menchi, F. I. Saldívar-Gonzalez, J. L. Medina-Franco and A. Trabocchi, Org. Biomol. Chem., 2019, 17, 1037–1052 RSC
;
(b) H. H. Kinfe, Org. Biomol. Chem., 2019, 17, 4153–4182 RSC
;
(c) T. J. Pawar, H. Jiang, M. A. Vázquez, C. V. Gómez and D. C. Cruz, Eur. J. Org. Chem., 2018, 1835–1851 CrossRef CAS
;
(d) D. Tejedor, S. López-Tosco, G. Méndez-Abt, L. Cotos and F. García-Tellado, Acc. Chem. Res., 2016, 49, 703–713 CrossRef CAS PubMed
;
(e) S. Kotha, D. Deodhar and P. Khedkar, Org. Biomol. Chem., 2014, 12, 9054–9091 RSC
. - For reviews on the furans as building blocks in organic synthesis:
(a) M. Decostanzi, R. Auvergne, B. Boutevin and S. Caillol, Green Chem., 2019, 21, 724–747 RSC
;
(b) X. Kong, Y. Y. Zhu, Z. Fang, J. A. Kozinski, I. S. Butler, L. Xu, H. Song and X. J. Wei, Green Chem., 2018, 20, 3657–3682 RSC
;
(c) S. Chen, R. Wojcieszak, F. Dumeignil, E. Marceau and S. Royer, Chem. Rev., 2018, 118, 11023–11117 CrossRef CAS PubMed
;
(d) I. V. Trushkov, M. G. Uchuskin and A. V. Butin, Eur. J. Org. Chem., 2015, 2999–3016 CrossRef CAS
;
(e) F. van der Pijl, F. L. van Delft and F. P. J. T. Rutjes, Eur. J. Org. Chem., 2015, 4811–4829 CrossRef CAS
. - For reviews on Ugi reaction:
(a) Q. Wang, D.-X. Wang, M.-X. Wang and J. Zhu, Acc. Chem. Res., 2018, 51, 1290–1300 CrossRef CAS PubMed
;
(b) S. Shaabani and A. Dçmling, Angew. Chem., Int. Ed., 2018, 57, 16266–16268 CrossRef CAS PubMed
;
(c) B. H. Rotstein, S. Zaretsky, V. Rai and A. K. Yudin, Chem. Rev., 2014, 114, 8323–8359 CrossRef CAS PubMed
. - For selected recent examples of further transformation of Ugi-adducts:
(a) H. J. Ghazvini, T. J. J. Müller, F. Rominger and S. Balalaie, J. Org. Chem., 2019, 84, 10740–10748 CrossRef PubMed
;
(b) Y. He, Z. Liu, D. J. Wu, Z. H. Li, K. Robeyns, L. V. Meervelt and E. V. Van der Eycken, Org. Lett., 2019, 21, 4469–4474 CrossRef CAS PubMed
;
(c) A. Zidan, M. Cordier, A. M. El-Naggar, N. E. A. A. El-Sattar, M. A. Hassan, A. K. Ali and L. E. Kaïm, Org. Lett., 2018, 20, 2568–2571 CrossRef CAS PubMed
;
(d) A. Zidan, J. Garrec, M. Cordier, A. M. El-Naggar, N. E. A. Abd El-Sattar, A. K. Ali, M. A. Hassan and L. E. Kaim, Angew. Chem., Int. Ed., 2017, 56, 12179–12183 CrossRef CAS PubMed
;
(e) X. Du, J. Yu, J. Gong, M. Zaman, O. P. Pereshivko and V. A. Peshkov, Eur. J. Org. Chem., 2019, 2502–2507 CrossRef CAS
;
(f) Y. He, Z. Li, K. Robeyns, L. V. Meervelt and E. V. V. Eycken, Angew. Chem., Int. Ed., 2018, 57, 272–276 CrossRef CAS PubMed
. - L. Kaim, L. Grimaud and S. Wagschal, Chem. Commun., 2011, 47, 1887–1889 RSC
. -
(a) J. Liu, X. Xu, J. Li, B. Liu, H. Jiang and B. Yin, Chem. Commun., 2016, 52, 9550–9553 RSC
;
(b) J. Liu, H. Peng, L. Lu, X. Xu, H. Jiang and B. Yin, Org. Lett., 2016, 18, 6440–6443 CrossRef CAS PubMed
.
Footnote |
† Electronic supplementary information (ESI) available. See DOI: 10.1039/d0ra01830a |
|
This journal is © The Royal Society of Chemistry 2020 |