DOI:
10.1039/D0RA01524E
(Paper)
RSC Adv., 2020,
10, 11365-11370
Synthesis, crystal structures and magnetic properties of two heterometallic {Ln8Cr4} (Ln = Gd3+ and Tb3+) complexes with one-dimensional wave chain structure†
Received
17th February 2020
, Accepted 11th March 2020
First published on 20th March 2020
Abstract
Two isomorphic heterometallic 3d–4f cluster-based materials, formulated [Gd8Cr4(IN)18(μ3-O)2(μ3-OH)6(μ4-O)4(H2O)10]·13H2O (1) and [Tb8Cr4(IN)18(μ3-O)2(μ3-OH)6(μ4-O)4(H2O)10]·13H2O (2) (abbreviation: {Ln8Cr4}: Ln = Gd3+ (1); Tb3+ (2); HIN = isonicotinic acid), were achieved by hydro-/solvothermal method through using the ligand HIN. X-ray diffraction analysis illustrates eight lanthanide ions (Ln = Gd3+, Tb3+) and four transition-metal ions (Cr3+) of {Ln8Cr4} were constructed from two classical “drum-like” {Ln4Cr2} structures associated by organic ligands HIN, displaying a one-dimensional wave chain structure, which is rare. The magnetic properties of {Gd8Cr4} were inspected and showed the existence of antiferromagnetic coupling interactions between contiguous metal ions. On top of this, the magnetic entropy change of ΔSm can attain 23.40 J kg−1 K−1 (44.90 mJ cm−3 K−1) at about 3 K and ΔH = 7 T. Besides, fluorescence measurements of {Tb8Cr4} display typical characteristic Tb-based luminescence.
Introduction
The study of heterometallic 3d–4f metal clusters has evoked extensive attention from researchers owing to their structural aesthetic and prominent application in optics and magnetism.1–10 Focused on 3d or 4f compounds, 3d–4f clusters combine the properties of different metal atoms in the same molecule, which can lead to tailorable topologies and interesting magnetic properties.11–13 As far as we know, magnetic refrigeration occurs because of the phenomenon named the magnetocaloric effect (MCE), which relies on the variation of isothermal magnetic entropy (−ΔSm) as well as adiabatic temperature (−ΔTad) under different applied magnetic field (ΔH).14–16 Magnetic materials as one of the most prevalent materials in the field of materials science, have always been a hotspot for their potential applications in environmentally friendly magnetic refrigeration materials with marvelous MCE.5,15,16 In order to acquire large −ΔSm, it is natural that a magnetic cooler should possess a high spin ground state, a large magnetic density and negligible magnetic anisotropy.17 Based on the above considerations, 3d–Gd metal clusters can be a good candidate to trigger a large MCE.18,19
By introducing 3d metal ions into lanthanide clusters can not only exhibit a more superior nature, but also have a broad application prospect in fields, including light, electricity, magnetism and catalysis.9,10 At present, more and more 3d–Gd metal clusters with large MCE have been prepared, ranging from molecular clusters to multi-dimensional coordination polymers where the maximum −ΔSm attains 42.8 J kg−1 K−1 at 3 K for ΔH = 7 T.20–26 Among plentiful 3d ions, Cr3+ ions, closing to isotropic, makes them a good selection as a raw material for magnetic refrigerants.27 But by reason of the inert nature of Cr3+ ions, making it has been untoward to combine them with lanthanide ions to synthesis polynuclear complexes and possess captivating structures.6 Therefore, works on Cr–Ln compounds are seldom been investigated and an effective synthesis to form Cr–Ln compounds has not been developed.
The progress in designing and constructing Cr–Ln compounds with giant MCE has been substantial but also uneven, such as {Cr4Ln},27 {Cr6Ln2},28 {Cr2Ln3},29 {Cr3Ln3},30 {Cr2Ln4},17,31 {Cr4Ln4},6,30 {Cr12Ln4},28 {Cr2Ln5},32 {Cr4Ln8}32 and {Cr8Ln8}15 clusters, where the maximum −ΔSm attains 38.33 J kg−1 K−1 at 2 K for ΔH = 7 T (see detailed in Table 2). Among reported literatures, Cr–Ln compounds have charming structures, it is more common that ring-like {Cr4Ln4},30 {Cr3Ln3},30 cage-like {Cr6Ln2},28 {Cr12Ln4}28 and wheel-like {Cr4Ln8},32 {Cr8Ln8}15 clusters. Although the charming structures, there are only a few cases of Cr–Ln clusters with more than ten cores up to now.
Table 1 Crystal data and structure refinements for 1–2
Compound |
1 |
2 |
R1 = Σ||Fo| − |Fc||/Σ|Fo|. wR2 = Σ[w(Fo2 − Fc2)2]/Σ[w(Fo2)2]1/2. |
Formula |
C108H124Cr4Gd8N18O71 |
C108H124Cr4Tb8N18O71 |
Formula weight |
4276.24 |
4289.60 |
T (K) |
296(2) |
296(2) |
Crystal system |
Triclinic |
Triclinic |
Space group |
P![[1 with combining macron]](https://www.rsc.org/images/entities/char_0031_0304.gif) |
P![[1 with combining macron]](https://www.rsc.org/images/entities/char_0031_0304.gif) |
a (Å) |
13.96(2) |
13.71(8) |
b (Å) |
14.47(2) |
14.20(8) |
c (Å) |
21.07(3) |
20.60(12) |
β (deg) |
80.221(16) |
80.096(10) |
V (Å3) |
4185(10) |
3945.4(4) |
Z |
1 |
1 |
Dc (mg m−3) |
1.697 |
1.805 |
μ (mm−1) |
3.458 |
3.891 |
F (000) |
2074 |
2082 |
θ range (deg) |
0.982–25.099 |
0.989–25.099 |
Limiting indices |
−16 ≦ h ≦ 16, −17 ≦ k ≦ 17, −21 ≦ l ≦ 24 |
−16 ≦ h ≦ 16, −16 ≦ k ≦ 16, −24 ≦ l ≦ 23 |
Reflections collected |
28 873 |
28 601 |
Independent reflections |
14 457 [R(int) = 0.0320] |
13 881 [R(int) = 0.0336] |
Data/restraints/parameters |
14 457/95/955 |
13 881/116/956 |
GOF |
1.061 |
1.100 |
R1a, wR2b [I > 2σ(I)] |
R1 = 0.0346 wR2 = 0.0931 |
R1 = 0.0310 wR2 = 0.0871 |
R1, wR2 (all data) |
R1 = 0.0436 wR2 = 0.1007 |
R1 = 0.0353 wR2 = 0.0899 |
Table 2 Comparison of −ΔSm for reported Gd-based polymetallic clusters
Complexes |
−ΔSm (J kg−1 K−1) |
ΔH (T) |
T (K) |
Ref. |
{Cr2Gd4} |
38.33 |
7.0 |
2.0 |
31c |
{Cr2Gd3} |
38.3 |
7.0 |
2.0 |
29 |
{Cr2Gd4} |
36.86 |
7.0 |
2.0 |
17 |
{Cr4Gd8} |
33.8 |
7.0 |
2.0 |
32 |
{Cr2Gd5} |
25.2 |
7.0 |
5.0 |
32 |
{Cr4Gd8} |
23.40 |
7.0 |
3.0 |
This work |
{Cr4Gd} |
18.31 |
8.0 |
3.5 |
27 |
{Cr4Gd4} |
18.08 |
7.0 |
3.0 |
6 |
Based on our group previous study in Cr–Gd compounds, we have done further investigation in the synthesis and magnetism of Cr–Ln compounds. Luckily, two isostructural one-dimensional chain-like compounds, {Ln8Cr4} (Ln = Gd3+ (1); Tb3+ (2)), were successfully synthesized by hydro-/solvothermal method, which are rare. The birth of these two twelve cores compounds enrich the family of Cr–Ln, which make a contribution to the field in magnetic refrigeration. In this work, magnetic and optical studies were performed on compounds 1 and 2, respectively. The magnetic study results demonstrate that compound 1 exhibits magnetocaloric effect values at about 3 K under ΔH = 7 T is 23.40 J kg−1 K−1. Simultaneously, fluorescence measurements of compound 2 reveal typical characteristic Tb-centered emissions in the visible region.
Experimental section
Materials and physical property studies
All reactants were reagent grade and used as received. The FT-IR spectrum was recorded in the span of 4000–400 cm−1 with a Nicolet Impact 410 FTIR spectrometer using pressed KBr pellets. The data of Powder X-ray diffraction (PXRD) (2θ = 5–50°) was obtained by a Bruker D8X diffractometer, which configured with monochromatized Cu-Kα (λ = 0.15418 nm) radiation. Thermogravimetric (TG) measurements were performed on a Diamond thermogravimetric analyzer in a flowing N2 with a heating ratio of 10 °C min−1 in the range of 25–1000 °C. The data of magnetic susceptibility was conducted by a Quantum Design MPMS-XL 7 SQUID magnetometer and diamagnetic corrections were estimated by means of Pascal's constants.
X-ray crystallography
The data of compounds 1–2 of the single-crystal X-ray diffraction were collected on a Bruker SMART Apex II CCD diffractometer by means of Mo-Kα radiation (λ = 0.71073 Å) through using ω–2θ scan technique. And then crystalline structures of the two compounds were figured out with the SHELX-2019/3 program package using direct methods and refined by the fullmatrix least-squares minimization.
Synthesis of compounds
[Gd8Cr4(IN)18(μ3-O)2(μ3-OH)6(μ4-O)4(H2O)10]·13H2O (1). A mixture of Gd2O3 (0.1994 g, 0.55 mmol), isonicotinic acid (0.2396 g, 2.00 mmol), CrCl3·6H2O (0.1464 g, 0.55 mmol) and HCOOH (0.0183 g, 0.35 mmol) was dissolved in distilled water (12 ml). Stirring for 12 h at the ambient temperature and then 2 mol l−1 NaOH was added into the above mixture (pH = 4.00). A Teflon-lined vessel was sealed and raised the vessel to the temperature of 180 °C for 7 days. Cooling the vessel to ambient temperature and then the expected magenta block crystals of 1 were obtained (yield: 31% basis on Gd). Elemental analysis found (%): C 31.19, H 2.69, N 6.06 (calcd (%): C 30.14, H 2.54, N 6.01).
[Tb8Cr4(IN)18(μ3-O)2(μ3-OH)6(μ4-O)4(H2O)10]·13H2O (2). The synthesis of compound 1 and 2 was similar to that of 2 except that Gd2O3 was used instead of Tb4O7 (0.4112 g, 0.55 mmol). (yield: 42% basis on Tb). Elemental analysis found (%): C 30.89, H 2.81, N 6.00 (calcd (%): C 29.53, H 2.67, N 5.94).
Results and discussion
Syntheses
Hydro-/solvothermal synthesis have recently been confirmed as a valid and simple approach to the synthesis of heterometallic 3d–4f metal clusters. Many variables factors have an impact on the final products, for example, the ligand/metal ratio, solvents, pH values, temperature, reaction time and duration. To achieve Cr–Ln compounds, we select Ln2O3, CrCl3·6H2O, isonicotinic acid, HCOOH, and H2O as the reactants. In this work, only two isostructural compounds, {Ln8Cr4} (Ln = Gd3+ (1); Tb3+ (2)), were successfully synthesized under hydrothermal conditions. Although HCOOH does not appear in the structure of the final product, but without adding HCOOH in the process of synthesis, we could not get the target product. At the same time, we have also changed other lanthanide sources, such as Er, Ho, Dy and Tb. Unfortunately, no other isomorphic compounds were obtained except for the isomorphic Tb compounds. In addition to this, we tried to replace CrCl3·6H2O with other chromium compounds, but also failed. It is tricky to synthesize the aforesaid compounds, and it perhaps that we do not find appropriate conditions for other isomorphic compounds. The synthesis is carried out at pH value (4.0–4.5) conditions. Moreover, we made attempts to convert the reaction temperature between 80 and 180 °C, but except the given synthesis temperature, no crystals were found (Table 1).
Structure of [Gd8Cr4(IN)18(μ3-O)2(μ3-OH)6(μ4-O)4(H2O)10]·13H2O (1)
Compounds 1–2 belong to the triclinic system, P1 space group and the two compounds are isomorphic, herein, the structure of compound 1 was taken as the example. In order to describe the structure clearly, free water molecules and H atoms are omitted. As shown on Fig. 1a, {Gd8Cr4} is composed of four Cr3+ cations, eight Gd3+ cations, eighteen IN− anions, two μ3-O2− group, six μ3-OH− group, four μ4-O2− group, ten coordination water molecules and seven lattice water molecules.
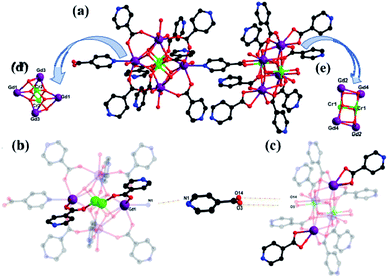 |
| Fig. 1 The unit of {Gd8Cr4} (a), FBU (b) and SBU (c); (d) and (e) the building unit of “drum-like” {Gd4Cr2} metallic framework from different directions. For clarity, free water molecules and H atoms are omitted. Colour codes: Gd3+, purple; Cr3+, green; O, red; N, blue. | |
The structural unit {Gd8Cr4} can be viewed as the interrelation of two {Gd4Cr2} building units, which are both made of two Cr3+ cations and four Gd3+ cations, named first building unit and secondary building unit (abbreviation: FBU (Fig. 1b) and SBU (Fig. 1c). Although two {Gd4Cr2} building units with the same “drum-like” metallic framework (Fig. 1d and e), there are obvious discrepancy in the coordination modes of HIN ligands. In the structure, the IN− ligands adopt four coordination modes (I–IV, Fig. 2a): μ3-η1: η1: η1; μ2-η1: η1: η0; μ2-η1: η1: η0; μ1-η1: η0: η0. For mode I, the IN− anion bridges one Gd3+ and Cr3+ ion and uses the nitrogen atom to bridge another Gd3+ ion while the IN− anion links to one Gd3+ and one Cr3+ ion in mode II. For mode III and IV, the IN− anion bridges two Gd3+ ions and one Gd3+ ion, respectively. In FBU, the IN− ligands adopt four mode II and four mode III, while in SBU, the IN− ligands addition to adopt two mode II and four mode III, but also in two mode IV, where the IN− anions bridge one Gd3+ ion. Here, the ligand HIN in mode I functions solely as a bridging ligand, N1 on HIN is linked to Gd1 in FBU and carboxyl oxygen O3 and O14 are linked to Cr1 and Gd2 in SBU, which makes neighboring drum-like FBU and SBU connect to form unit {Gd8Cr4}. The neighboring {Gd8Cr4} units are linked by HIN forming a rare one-dimensional (1D) wave chain structure (Fig. 2b), in which the adjacent chains interact with each other by π–π stacking interactions to form a two-dimensional (2D) layered structure between pyridyl rings and further interact with each other to build up a three-dimensional (3D) network by π–π interactions, as shown in Fig. 3.
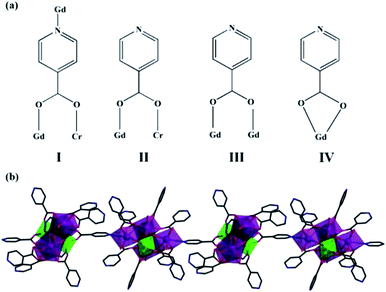 |
| Fig. 2 (a) Four kinds of coordinate modes of HIN in 1; (b) 1D chain structure in 1. | |
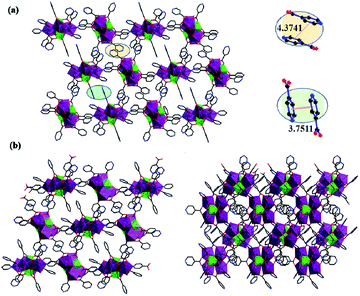 |
| Fig. 3 (a) Two-dimensional structure of 1; (b) three-dimensional structure of 1 (viewing from a axis and c axis). | |
Of the eight Gd3+ ions, with four coordination environments, which are all eight-coordinated (Fig. 4a–d). Among them, Gd1 is connected with seven O atoms (O11, O12, O13, N1, O6, O7, O10, O3W) from HIN ligands, bridging oxygen, and one water molecule. Gd2 is completed by three O atoms (O14, O15, O16) from HIN ligands, two water molecules (O4W, O5W) and three bridging oxygen (O1, O2, O4). Similarly, Gd3 is connected to two water oxygen (O1W, O2W), three bridged oxygen (O6, O7, O10) and three oxygen (O17, O18, O19) from HIN ligands. While Gd4 is connected to three bridged oxygen (O1, O2, O4) and the rest oxygen from HIN ligands. Beyond that, Cr3+ ions (Fig. 4e and f) are all six-coordinated with six oxygen atoms, and two of which are from HIN ligands and the rest are bridged oxygen. For compound 1, the interval value of Cr–O distances are 1.974(4) to 2.057(5) Å and the Gd–O are 2.367(5) to 2.577(5) Å, which are all in line with the literatures (Table S1†).27,32 Further, the bond angles around O–Cr–O and O–Gd–O, respectively, varying from 83.34(18) to 179.30(17)° and 51.43(17) to 148.96(14)° (Table S2†).33
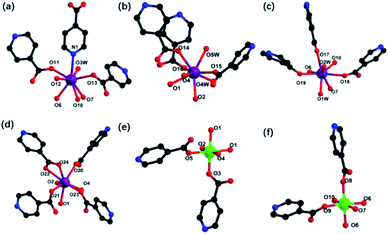 |
| Fig. 4 (a) Ball-and-stick of coordination mode of Gd1 (a), Gd2 (b), Gd3 (c), Gd4 (d), Cr1 (e), Cr2 (f). | |
In comparison with the previously reported hexanuclear {Ln4Cr2} clusters by Zhai et al. in 2018,31a which has the same metal skeleton {Ln4Cr2} as the compound we obtained. However, the reported {Ln4Cr2} cluster is composed of two symmetry-related units {Ln2Cr}, then is further linked together by sulfate ions, resulting in a 3D framework. While in this work, the two adjacent “drum-like” {Ln4Cr2} cluster is related to each other by IN− ligands, and then the carboxyl group on the ligand is bonded to the nitrogen to form a 1D chain structure, which is rare.
IR spectra
The IR spectra of the two compounds have similar infrared spectrum curves, which are presented in Fig. S5 and S6.† Taking compound 1 as an example: the strong peak at 3412 cm−1 could be considered as the stretching vibration of –OH. Contemporary, the sharp peak at 1608 cm−1 infers the existence of pyridine ring, while the peaks at 1547 cm−1 and 1417 cm−1 are largely thanks to the anti-symmetric stretching vibration and the symmetric stretching vibration of the carboxyl group, respectively. Other peaks of compound 1, such as peak at 774, 679, 563 cm−1, showing the stretching vibration of the metal oxygen bond.
Powder X-ray diffraction
As shown in the Fig. S7 and S8,† the measured values are basically consistent with the theoretical spectrum, indicating the two compounds are pure. At the same time, slight difference in intensity can be attributed to the limitations of instrument performance or the selective orientation effect of the sample.
Thermogravimetric analysis
As shown in Fig. S9 and S10,† weight loss curves of the two compounds are semblable, which can be divided into three stages. Taking compound 2 as an example: the first mass loss of 8.52% in the temperature range of 25–140 °C is caused by the removal of free water molecules and coordination water (theoretical 8.58%); the second part of the curve remains basically unchanged between 140 and 425 °C, which maybe due to the stability of the structure; when the temperature rises above 425 °C, the entire compound skeleton gradually collapses as the organic ligand isonicotinic acid is lost.
Magnetic studies
The solid-state magnetic susceptibility (χMT − T) of 1 was investigated between 300 and 1.8 K in an applied field of 1.0 kOe. As shown in Fig. 5, the observed χMT value for compound 1 at 300 K is 66.11 cm3 K mol−1, which is lower than the expected value of 70.5 calculated for eight isolated Gd3+ ions (S = 7/2, g = 2) and four isolated Cr3+ ions (S = 3/2, g = 2). With temperature dropping, the χMT value falling to 57.40 cm3 K mol−1 at 25 K. And on further cooling, the value declines abruptly at 1.8 K and reaches a minimum of 13.14 cm3 K mol−1, which are characteristic of antiferromagnetic coupling interactions. Fitting data with the Curie–Weiss law gives parameters C = 66.91 cm3 K mol−1 and θ = −4.34 K, further confirming the antiferromagnetic coupling performance.
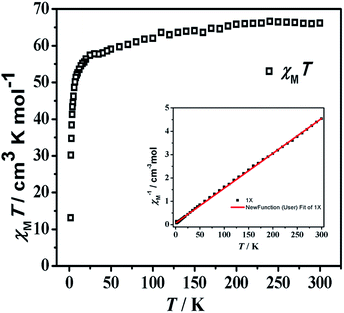 |
| Fig. 5 Plot of χMT vs. T for 1. Insert: Temperature dependence of 1/χM. | |
Magnetization measurements (M vs. H) of compound 1 was examined in multifarious temperatures between 1.8 and 10 K (Fig. S11†). It exhibits a rapid increase at lower filed and incline to keep jarless at 7 T. For compound 1, the magnetization reaches 52.06 Nβ at 1.8 K and 7 T. In order to estimate the magnetocaloric effect, the Maxwell equation ΔSm(T) = ∫[∂M(T, H)/∂T]HdH can be applied to calculate ΔSm to the experimental value.34 In Fig. 6, the magnetic entropy value has reached a value of about 14 J kg−1 K−1 at ΔH = 3 T. At last, the observed maximum −ΔSm for compound 1 of 23.40 J kg−1 K−1 is obtained at 3.0 K for ΔH = 7 T, which is less than the theoretical maximum entropy value is 44.39 (based on eight isolated Gd3+ ions and four isolated Cr3+ ions calculation), owing to the feeble antiferromagnetic interactions within the metal ions.
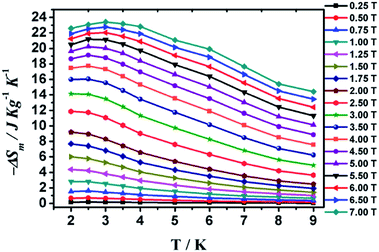 |
| Fig. 6 Values of −ΔSm calculated from the magnetization data for 1 at different fields and temperatures. | |
Luminescence properties
The solid-state fluorescence spectrum of 2 was measured under different excitation wavelengths. As can be seen in Fig. 7, the Tb3+ compound exhibits typical photoluminescence signals. When excited at 367 nm, the solid-state fluorescence peaks for compound 2 exhibits four bands from 490 to 624 nm, which are derived from the electronic transition of Tb3+ between 5D0 → 7Fj (j = 6, 5, 4, 3).17,35,36 Among them, the transition of the electric dipolar of 5D0 → 7F6 at 490 nm and the transition of the magnetic dipolar of the peak 5D0 → 7F5 at 546 nm. Additionally, the peaks at 586 nm and 624 nm pertain to the transition of 5D0 → 7F4 and 5D0 → 7F3 electrons, which are agreement with literatures.37–39
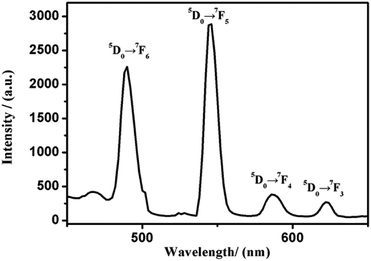 |
| Fig. 7 The solid-state fluorescence spectrum of 2 at room temperature. | |
Conclusions
In brief, two twelve-nuclear compounds {Ln8Cr4} were successfully obtained by hydro-/solvothermal method, displaying one-dimensional wave chain structure, which is rare been reported before. The MCE of compound 1 is 23.40 J kg−1 K−1 at about 3.0 K and ΔH = 7 T. In the meantime, fluorescence measurements of compound 2 reveal typical of Tb-based luminescence, which indicates it may be photoluminescence materials with great promise. These results enrich the existing field of Cr–Ln compounds, which played a certain driving force for the design and synthesis of molecular refrigerants.
Conflicts of interest
There are no conflicts to declare.
Acknowledgements
This work was supported by the Natural Science Foundation of Jiangsu Province (BK20191359) and China (Grant 21571103), the Major Natural Science Projects of the Jiangsu Higher Education Institution (Grant 16KJA150005).
Notes and references
- X. Y. Zheng, Y. H. Jiang, G. L. Zhuang, D. P. Liu, H. G. Liao, X. J. Kong, L. S. Long and L. S. Zheng, J. Am. Chem. Soc., 2017, 139, 18178–181811 CrossRef CAS PubMed
. - Q. F. Lin, J. Li, Y. Y. Dong, G. P. Zhou, Y. Song and Y. Xu, Dalton Trans., 2017, 46, 9745–9749 RSC
. - J. Dong, P. Cui, P. H. Shi, P. Cheng and B. Zhao, J. Am. Chem. Soc., 2015, 137, 15988–15991 CrossRef CAS PubMed
. - J. B. Peng, X. J. Kong, Q. C. Zhang, M. Orendač, J. Prokleška, Y. P. Ren, L. S. Long, Z. P. Zheng and L. S. Zheng, J. Am. Chem. Soc., 2014, 136, 17938–17941 CrossRef CAS PubMed
. -
(a) X. Y. Zheng, X. J. Kong, Z. P. Zheng, L. S. Long and L. S. Zheng, Acc. Chem. Res., 2018, 51, 517–525 CrossRef CAS PubMed
;
(b) X. Y. Zheng, J. Xie, X. J. Kong, L. S. Long and L. S. Zheng, Coord. Chem. Rev., 2019, 378, 222–2363 CrossRef CAS
. - C. H. Cui, J. P. Cao, X. M. Luo, Q. F. Lin and Y. Xu, Chem.–Eur. J., 2018, 24, 15295–15302 CrossRef CAS PubMed
. - X. Meng, W. Shi and P. Cheng, Coord. Chem. Rev., 2019, 378, 134–150 CrossRef CAS
. - J. Long, J. Rouquette, J. M. Thibaud, R. A. Ferreira, L. D. Carlos, B. Donnadieu, V. Vieru, L. F. Chibotaru, L. Konczewicz, J. Haines, Y. Guari and J. Larionova, Angew. Chem., Int. Ed., 2015, 54, 2236–2240 CrossRef CAS PubMed
. - M. Evangelisti and E. K. Brechin, Dalton Trans., 2010, 39, 4672–4676 RSC
. - F. B. Yu, Q. Gao, B. Zhang, Z. X. Meng and Z. Chen, Int. J. Refrig., 2003, 26, 622–636 CrossRef
. - Z. G. Gu, C. Zhan, J. Zhang and X. H. Bu, Chem. Soc. Rev., 2016, 45, 3122–3144 RSC
. - H. L. Qian, C. X. Yang and X. P. Yan, Nat. Commun., 2016, 7, 12104 CrossRef CAS PubMed
. - Z. Y. Li, Y. L. Xu, X. F. Zhang, B. Zhai, F. L. Zhang, J. J. Zhang, C. Zhang, S. Z. Li and G. X. Cao, Dalton Trans., 2017, 46, 16485–16492 RSC
. - R. Sibille, T. Mazet, B. Malaman and M. Francois, Chem.–Eur. J., 2012, 18, 12970–12973 CrossRef CAS PubMed
. - L. Qin, J. Singleton, W. P. Chen, H. Nojiri, L. Engelhardt, R. E. Winpenny and Y. Z. Zheng, Angew. Chem., Int. Ed., 2017, 56, 16571–16574 CrossRef CAS PubMed
. - J. W. Sharples, Y. Z. Zheng, F. Tuna, E. J. L. McInnes and D. Collison, Chem. Commun., 2011, 47, 7650–7652 RSC
. - C. H. Cui, W. W. Ju, X. M. Luo, Q. F. Lin, J. P. Cao and Y. Xu, Inorg. Chem., 2018, 57, 8608–8614 CrossRef CAS PubMed
. - J. A. Sheikh and A. Clearfield, Inorg. Chem., 2017, 56, 2843–2848 CrossRef CAS PubMed
. - S. Biswas, A. K. Mondal and S. Konar, Inorg. Chem., 2016, 55, 2085–2090 CrossRef CAS PubMed
. - J. L. Liu, Y. C. Chen, F. S. Guo and M. L. Tong, Coord. Chem. Rev., 2014, 281, 26–49 CrossRef CAS
. - W. P. Chen, P. Q. Liao, Y. Z. Yu, Z. P. Zheng, X. M. Chen and Y. Z. Zheng, Angew. Chem., Int. Ed., 2016, 55, 9375–9379 CrossRef CAS PubMed
. - S. J. Liu, C. Cao, S. L. Yao, T. F. Zheng, Z. X. Wang, C. Liu, J. S. Liao, J. L. Chen, Y. W. Li and H. R. Wen, Dalton Trans., 2017, 46, 64–70 RSC
. - X. Y. Zheng, J. Xie, X. J. Kong, L. S. Long and L. S. Zheng, Coord. Chem. Rev., 2019, 378, 222–236 CrossRef CAS
. - X. Y. Zheng, X. J. Kong, Z. Zheng, L. S. Long and L. S. Zheng, Acc. Chem. Res., 2018, 51, 517–525 CrossRef CAS PubMed
. - F. Torres, J. M. Hernandez and X. Bohigas, Appl. Phys. Lett., 2000, 77, 3248–3250 CrossRef CAS
. - W. P. Chen, J. Singleton, L. Qin, A. Camón, L. Engelhardt, F. Luis, R. E. P. Winpenny and Y. Z. Zheng, Nat. Commun., 2018, 9, 1–6 CrossRef PubMed
. - O. Blacque, A. Amjad, A. Caneschi, L. Sorace and P. E. Car, New J. Chem., 2016, 40, 3571–3577 RSC
. - A. McRobbie, A. R. Sarwar, S. Yeninas, H. Nowell, M. L. Baker, D. Allan, M. Luban, C. A. Muryn, R. G. Pritchard, R. Prozorov, G. A. Timco, F. Tuna, G. F. S. Whitehead and R. E. P. Winpenny, Chem. Commun., 2011, 47, 6251–6253 RSC
. - K. S. Pedersen, G. Lorusso, J. J. Morales, T. Weyhermüller, S. Piligkos, S. K. Singh, D. Larsen, M. Schau-Magnussen, G. Rajaraman, M. Evangelisti and J. Bendix, Angew. Chem., Int. Ed., 2014, 53, 2394–2397 CrossRef CAS PubMed
. -
(a) J. D. Leng, A. K. Kostopoulos, L. H. Isherwood, A. Ariciu, F. Tuna, I. J. Vitórica-Yrezábal, R. G. Pritchard, G. F. S. Whitehead, G. A. Timco, D. P. Mills and R. E. P. Winpenny, Dalton Trans., 2018, 47, 6361–6369 RSC
;
(b) J. Rinck, G. Novitchi, W. V. D. Heuvel, L. Ungur, Y. Lan, W. Wernsdorfer, C. E. Ason, L. F. Chibotaru and A. K. Powell, Angew. Chem., Int. Ed., 2010, 49, 7583–7587 CrossRef CAS PubMed
. -
(a) Z. Y. Li, J. J. Zhang, S. Q. Liu, H. Zhang, Y. J. Sun, X. Y. Liu and B. Zhai, Cryst. Growth Des., 2018, 18, 7335–7342 CrossRef CAS
;
(b) H. Xiang, W. G. Lu, W. X. Zhang and L. Jiang, Dalton Trans., 2013, 42, 867–870 RSC
;
(c) H. Xiang, W. G. Lu, L. Jiang, W. X. Zhang and Y. H. Lan, Eur. J. Inorg. Chem., 2016, 907–912 CrossRef CAS
. - J. J. Yin, C. Chen, G. L. Zhuang, J. Zheng, X. Y. Zheng and X. J. Kong, Inorg. Chem., 2020, 59(3), 1959–1966 CrossRef CAS PubMed
. - X. F. Tan, J. Zhou, H. H. Zou, L. S. Fu and Q. L. Tang, Inorg. Chem., 2017, 56, 10361–10369 CrossRef CAS PubMed
. - T. Q. Song, J. Dong, A. F. Yang, X. J. Che, H. L. Gao, J. Z. Cui and B. Zhao, Inorg. Chem., 2018, 57, 3144–3150 CrossRef CAS PubMed
. - J. Rinck, Y. Lan, C. E. Anson and A. K. Powell, Inorg. Chem., 2015, 54, 3107–3117 CrossRef CAS PubMed
. - X. X. He, Y. Liu, Y. Lv, Y. Y. Dong, G. H. Hu, S. Zhou and Y. Xu, Inorg. Chem., 2016, 55, 2048–2054 CrossRef CAS PubMed
. - Y. M. Zhou, H. G. Zhu, Z. X. Chen, M. Q. Chen, Y. Xu, H. Y. Zhang and D. Y. Zhao, Angew. Chem., Int. Ed., 2001, 40, 2166–2168 CrossRef CAS PubMed
. - K. H. Zangana, E. M. Pineda, J. Schnack and R. E. P. Winpenny, Dalton Trans., 2013, 42, 14045–14048 RSC
. - L. Qin, J. Singleton, W. P. Chen, H. Nojiri, L. Engelhardt, R. E. P. Winpenny and Y. Z. Zheng, Angew. Chem., 2017, 129, 16798–16801 CrossRef
.
|
This journal is © The Royal Society of Chemistry 2020 |