DOI:
10.1039/D0RA01244K
(Paper)
RSC Adv., 2020,
10, 19397-19400
A triflate and alkynyl protected Ag43 nanocluster with a passivated surface†
Received
9th February 2020
, Accepted 13th May 2020
First published on 20th May 2020
Introduction
Metal NCs have attracted a great amount of attention during the past decades not only for their aesthetic values but also for their potential applications in self-assembly, surface plasmon resonance (SPR), bio-markers, (electro)catalysis, etc.1–6 Fundamentally relating their structures with their properties, however, has encountered huge challenges.7 One obstacle is the difficulty in precisely characterizing the structures of NCs. To this end, many practical methodologies have been brought forward. Among them, single-crystal crystallography has special advantages in determining the total structures of NCs.8 Aiming to resolve the target's structure at atomic level, this methodology has found incomparable advantage in revealing the organic–metal interfacial structure of NCs. For example, the unprecedented “staple” bonding motif of thiolate of Au102 NC was firstly revealed by this method.9,10 There has since been a boom in the study of the potential applications of the atomically precise NCs, especially catalysis.11 The promoting effect of the surface ligands have been repeatedly observed in different types of reactions.12,13 However, to obtain the single crystals of NCs, the strong interaction between the NCs and ligands is usually necessary for stabilizing the NCs. Thus, the surface catalytically active sites might be blocked by the organic ligands. However, the researches addressing this issue of Ag NCs seem rare.
On the other side, a large number of thiolate and(or) phosphine protected Au or Ag NCs have been synthesized so far, such as Au25,14 Au38,15 Au279,16 Ag40,17 Ag44,18,19 Ag67,20 Ag146,21 Ag141,22 and many others.23 Beyond the conventional ligands, alkynes are drawing fast-increasing attentions, thanks to the flexible bonding modes of alkynes with metals.24–28 In recent years, there have been a considerable amount of NCs containing anions in their structures. Most of them, however, function as templates or counterions.29–32 Ag NCs or complexes featuring direct Ag–O bonding are mostly those with Ag(I) species.33 Examples of Ag(0) NCs protected by inorganic oxo anions are rare. Sun etc. recently reported a Ag(0) NC co-protected by CrO42− and tBuC
C−, [Ag48(C
CtBu)20(CrO4)7].34 Herein, we report the first example of a Ag(0) NC co-protected by triflate (OTf) and tert-butylacetylene (tBuC
C−), namely, Ag43(tBuC
C)24(CF3SO3)8 (denoted as Ag43). The detailed structure of Ag43 is analyzed and discussed. Significantly, we have found a strong passivation effect in the reaction of silanes with alcohol or H2O catalyzed by Ag43. This effect not only sharply decreased the reaction rate, but also exerted a direct influence on the reaction selectivity.
Results and discussion
Ag43 was synthesized by introducing a reducing agent, NaBH4 into an ethanol solution of AgSO3CF3, tert-butylacetylene (tBuC
C−), 1,4-bis-(diphenylphosphino)butane (dppb), and triethylamine (Et3N). The reaction was aged for 24 hours during which the colour gradually changed from pale-yellow to dark green. A block-shaped single crystal was obtained by slowly diffusing a mixed solvent of hexane and ether into the mother solution (ESI, Fig. S1†). Ag43 crystallizes in I2/m space group and its overall structure is shown in Fig. 1 and S2.† It consists of 43 silver atoms, 24 tBuC
C− and 8 OTf ligands. The Ag atoms are arranged into a three-shell Russian doll structure, Ag@Ag12@Ag30 (Fig. 1b). The Ag@Ag12 shells make up an icosahedron with a dodeca-coordinated Ag atom at the center (Fig. 1c). Bearing a Ag atom bonding with only Ag atoms endows Ag43 with partial Ag(0) character. The rest 30 Ag atoms of the outermost shell form 12 pentagons and 20 triangles. The two regular polygons are connected through edge-sharing, which makes each pentagon be enclosed by five triangles and each triangle by three pentagons. Geometrically, such an arrangement forms an icosidodecahedron, an Archimedean solid (Fig. 1d). The outermost shell is closely related with the ligands' distribution (see below). The average Ag–Ag bond length between the central Ag to Ag12 shell is 2.858 Å, while the value is 3.1 Å between Ag12 and Ag30 shells. Both are less than the sum of the van der Waals radii of two Ag atoms (3.44 Å), indicating argyrophilic interactions between the shells.
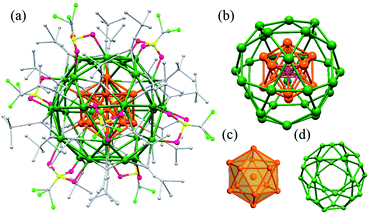 |
| Fig. 1 (a) The overall structure of Ag43(tBuC C)24(CF3SO3)8, Ag43. (b) The three shells of Ag@Ag12@Ag30. (c) The Ag@Ag12 centered icosahedron. (d) The outer Ag30 shell. Color legend: olive green and orange, Ag; pink, O; yellow, S; light green, F; gray, C. H atoms are omitted for clarity. | |
As mentioned, the distribution of peripheral ligands is dependent on the arrangement of silver atoms, especially the Ag30 shell (Fig. 2a). There are 24 tBuC
C− ligands which can be equally divided into two groups. Each of the first half penetrates one of the twelve pentagons of Ag30 shell and bonded to Ag12 shell through σ bond. Each of the twelve tBuC
C− also bonds with the pentagon through π bonds (Fig. 2a). The rest 12 tBuC
C− ligands along with 8 OTf ligands, which amount to 20, coordinated with the 20 triangles of the Ag30 shell respectively. It is noteworthy that every tBuC
C− and OTf has the similar μ3 coordination mode (Fig. 2b and c). It is also worth noting that although OTf bonding with Ag atoms are commonly seen in Ag(I) complexes or clusters, especially those with low nuclearity,35,36 Ag(0) NC protected by OTf has barely been reported. The bond length of the Ag–O bond is 2.415 Å, which is within the common range typical Ag–O bonds.37,38
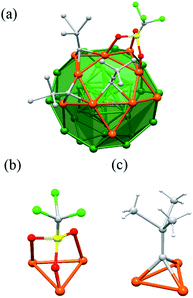 |
| Fig. 2 (a) The coordination mode of Ag43 surface ligands. The μ3 bonding motifs of OTf (b) and tBuC C− (c) with the Ag30 shell. For clarity, only parts of the ligands are shown. | |
We investigated the optical properties of Ag43 in CH2Cl2 (Fig. 3). As illustrated in Fig. 3a, the UV-vis absorption spectrum of Ag43 shows two absorption features: one major peak at 434 nm and a shoulder at 620 nm. FT-IR spectra of Ag43, tBuC
C− and the precursor AgSO3CF3 are shown in Fig. 3b. The stretching of
C–H at 3272 cm−1 is no longer present in Ag43, indicating the bonding of tBuC
C− with Ag.39 The characteristic peaks of SO3CF3− ranging from 1000 to 1500 cm−1, on the other hand, are still prominent in the spectrum of Ag43. These information further confirmed both ligands are present on Ag43.
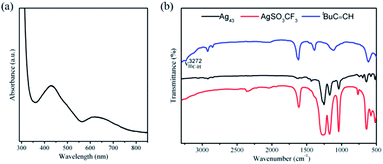 |
| Fig. 3 (a) UV-vis absorption spectrum of Ag43 in CH2Cl2 solution. (b) FT-IR spectrum of Ag43 (black), tBuC C− (blue) and AgSO3CF3 (red). | |
The possible influence of the Ag43 surface ligands on catalysis was evaluated in the reaction of a sterically demanding substrate dimethylphenylsilane (denoted as silane) with H2O or 1-butanol (Scheme 1).40,41 As a benchmark, polyvinylpyrrolidone (PVP) stabilized Ag nanoparticles (Ag/PVP) were also fabricated following a reported procedure.42 The results are summarized in Fig. 4. We can see that for either H2O or alcohol as substrate, the reaction catalyzed by Ag43 always showed significantly lower reactivity than Ag/PVP, although the amount of Ag as well as the other reaction conditions were kept the same (Fig. 4a and c). Despite the small size of Ag43 (less than 1 nm), the surface ligands still exerted a direct impact on limiting the contact of substrate with Ag. This also influenced the product distribution. For Ag/PVP, full selectivity towards the target was always achieved. Ag43, however, afforded a significant amount of 1,3-diphenyl-1,1,3,3-tetramethyldisiloxane (denoted as disiloxane). As the reaction proceeded, the byproduct kept accumulating. For example, in hydrolysis reaction catalyzed by Ag43, when half the silane was consumed, the byproduct disiloxane accounted for 54% of the total product (Fig. 4b). The situation was similar in dehydrogenative coupling with 1-butanol (Fig. 4d). Not only the reaction rate was slower for Ag43, but disiloxane was also found as a major part of the products. Ag was fairly stable under such reaction condition. The light absorption Ag43 after reaction has similar features (Fig. S3†). Generally, the rate-limiting step of this reaction is the activation of alcohol. Thus, given the full occupation of the surface Ag site, this step might be inhibited, which gave silane more chance to react with each other. The exact mechanism, however, still requires further thorough investigation.
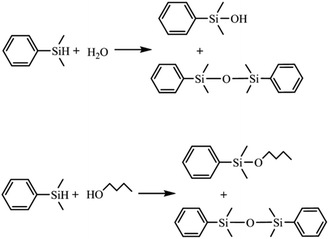 |
| Scheme 1 Reaction scheme of silane with H2O and 1-butanol. The side reaction is the condensation of two silane molecules. | |
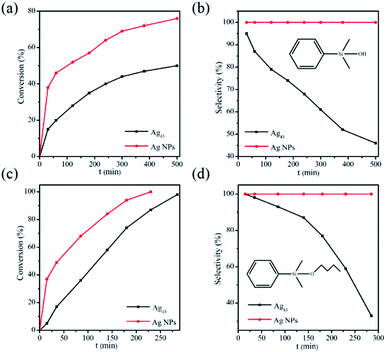 |
| Fig. 4 Tracing the reaction of silane with H2O catalyzed by Ag43 or Ag/PVP. (a) Conversion of silane and (b) the selectivity towards the target whose structure is shown in the figure. (c and d) are those for silane reacting with butanol. Reaction conditions: Ag (0.2 mmol), dimethylphenylsilane (2 mmol), H2O or 1-butanol (22 mmol), toluene (2 mL), 30 °C. The byproduct is 1,3-diphenyl-1,1,3,3-tetramethyldisiloxane for both reactions. | |
Conclusions
In conclusion, a triflate and tert-butylacetylene protected Ag43 is synthesized and characterized. For the first time the triflate is shown to function as an effective surface ligand for Ag(0) nanocluster. It bonds with Ag atoms in μ3 mode. Although Ag43 has a very small size, its catalytic ability is significantly worse than the PVP protected Ag nanoparticles. The surface ligands of Ag43 showed strong passivation effect for the reaction of silane with H2O or butanol. Such an effect also sharply decreased the selectivity towards the desired target, yielding a significant percentage of disiloxane as byproduct.
Conflicts of interest
There are no conflicts to declare.
Acknowledgements
We express our gratitude to National Natural Science Foundation of China (21972080, 21503123, 91961201 & 21871167), Sanjin Scholar, Shanxi “1331 Project” Key Innovative Research Team for financial support. We are also grateful for the help from the State Key Laboratory for Physical Chemistry of Solid Surfaces of Xiamen University.
Notes and references
- Z. Wu, Q. Yao, S. Zang and J. Xie, ACS Mater. Lett., 2019, 1, 237–248 CrossRef CAS.
- T. Higaki, Y. Li, S. Zhao, Q. Li, S. Li, X.-S. Du, S. Yang, J. Chai and R. Jin, Angew. Chem. Int. Ed., 2019, 58, 8291–8302 CrossRef CAS PubMed.
- J. Chai, H. Chong, S. Wang, S. Yang, M. Wu and M. Zhu, RSC Adv., 2016, 6, 111399–111405 RSC.
- Y. Wang, J. Zhang, L. Huang, D. He, L. Ma, J. Ouyang and F. Jiang, Chem.–Eur. J., 2012, 18, 1432–1437 CrossRef CAS PubMed.
- H. Qian, D.-E. Jiang, G. Li, C. Gayathri, A. Das, R. R. Gil and R. Jin, J. Am. Chem. Soc., 2012, 134, 16159–16162 CrossRef CAS PubMed.
- C.-A. J. Lin, C.-H. Lee, J.-T. Hsieh, H.-H. Wang, J. K. Li, J.-L. Shen, W.-H. Chan, H.-I. Yeh and W. H. Chang, J. Med. Biol. Eng., 2009, 29, 276–283 Search PubMed.
- A. W. Cook and T. W. Hayton, Acc. Chem. Res., 2018, 51, 2456–2464 CrossRef CAS PubMed.
- L. J. Farrugia, J. Appl. Crystallogr., 1999, 32, 837–838 CrossRef CAS.
- D.-e. Jiang, M. L. Tiago, W. Luo and S. Dai, J. Am. Chem. Soc., 2008, 130, 2777–2779 CrossRef CAS PubMed.
- P. D. Jadzinsky, G. Calero, C. J. Ackerson, D. A. Bushnell and R. D. Kornberg, Science, 2007, 318, 430–433 CrossRef CAS PubMed.
- G. Li and R. Jin, Acc. Chem. Res., 2013, 46, 1749–1758 CrossRef CAS PubMed.
- Y. Wang, X.-K. Wan, L. Ren, H. Su, G. Li, S. Malola, S. Lin, Z. Tang, H. Häkkinen, B. K. Teo, Q.-M. Wang and N. Zheng, J. Am. Chem. Soc., 2016, 138, 3278–3281 CrossRef CAS PubMed.
- X.-K. Wan, J.-Q. Wang, Z.-A. Nan and Q.-M. Wang, Sci. Adv., 2017, 3, e1701823 CrossRef PubMed.
- Z. Wu, J. Suhan and R. Jin, J. Mater. Chem., 2009, 19, 622–626 RSC.
- R. L. Donkers, D. Lee and R. W. Murray, Langmuir, 2004, 20, 1945–1952 CrossRef CAS.
- N. A. Sakthivel, S. Theivendran, V. Ganeshraj, A. G. Oliver and A. Dass, J. Am. Chem. Soc., 2017, 139, 15450–15459 CrossRef CAS PubMed.
- J. Chai, S. Yang, Y. Lv, T. Chen, S. Wang, H. Yu and M. Zhu, J. Am. Chem. Soc., 2018, 140, 15582–15585 CrossRef CAS PubMed.
- H. Yang, Y. Wang, H. Huang, L. Gell, L. Lehtovaara, S. Malola, H. Häkkinen and N. Zheng, Nat. Commun., 2013, 4, 2422 CrossRef PubMed.
- Y. Cao, J. Guo, R. Shi, G. I. N. Waterhouse, J. Pan, Z. Du, Q. Yao, L.-Z. Wu, C.-H. Tung, J. Xie and T. Zhang, Nat. Commun., 2018, 9, 2379–2384 CrossRef PubMed.
- M. J. Alhilaly, M. S. Bootharaju, C. P. Joshi, T. M. Besong, A. H. Emwas, R. Juarez-Mosqueda, S. Kaappa, S. Malola, K. Adil, A. Shkurenko, H. Häkkinen, M. Eddaoudi and O. M. Bakr, J. Am. Chem. Soc., 2016, 138, 14727–14732 CrossRef CAS PubMed.
- Y. Song, K. Lambright, M. Zhou, K. Kirschbaum, J. Xiang, A. Xia, M. Zhu and R. Jin, Nanoscale, 2018, 12, 9318–9325 CAS.
- L. Ren, P. Yuan, H. Su, S. Malola, S. Lin, Z. Tang, B. K. Teo, H. Häkkinen, L. Zheng and N. Zheng, J. Am. Chem. Soc., 2017, 139, 13288–13291 CrossRef CAS PubMed.
- J. Yan, B. K. Teo and N. Zheng, Acc. Chem. Res., 2018, 51, 3084–3093 CrossRef CAS PubMed.
- Z. Lei, X.-K. Wan, S.-F. Yuan, Z.-J. Guan and Q.-M. Wang, Acc. Chem. Res., 2018, 51, 2465–2474 CrossRef CAS PubMed.
- X.-K. Wan, Z.-J. Guan and Q.-M. Wang, Angew. Chem. Int. Ed., 2017, 56, 11494–11497 CrossRef CAS PubMed.
- M. Qu, H. Li, L.-H. Xie, S.-T. Yan, J.-R. Li, J.-H. Wang, C.-Y. Wei, Y.-W. Wu and X.-M. Zhang, J. Am. Chem. Soc., 2017, 139, 12346–12349 CrossRef CAS PubMed.
- G.-X. Duan, L. Tian, J.-B. Wen, L.-Y. Li, Y.-P. Xie and X. Lu, Nanoscale, 2018, 10, 18915–18919 RSC.
- S.-F. Yuan, P. Li, Q. Tang, X.-K. Wan, Z.-A. Nan, D. Jiang and Q.-M. Wang, Nanoscale, 2017, 9, 11405–11409 RSC.
- Q. M. Wang, Y. M. Lin and K. G. Liu, Acc. Chem. Res., 2015, 48, 1570–1579 CrossRef CAS PubMed.
- S.-D. Bian, H.-B. Wu and Q.-M. Wang, Angew. Chem. Int. Ed., 2009, 48, 5363–5365 CrossRef CAS PubMed.
- Z. Wang, R. K. Gupta, G. G. Luo and D. Sun, Chem. Rec., 2020, 20, 389–402 CrossRef CAS PubMed.
- S.-D. Bian, J.-H. Jia and Q.-M. Wang, J. Am. Chem. Soc., 2009, 131, 3422–3423 CrossRef CAS PubMed.
- R. Terroba, M. B. Hursthouse, M. Laguna and A. Mendia, Polyhedron, 1999, 18, 807–810 CrossRef CAS.
- S. S. Zhang, F. Alkan, H. F. Su, C. M. Aikens, C. H. Tung and D. Sun, J. Am. Chem. Soc., 2019, 141, 4460–4467 CrossRef CAS PubMed.
- S. L. James, D. M. P. Mingos, A. J. P. White and D. J. Williams, Chem. Commun., 1998, 2323–2324 RSC.
- M. Bardají, O. Crespo, A. Laguna and A. K. Fischer, Inorg. Chim. Acta, 2000, 304, 7–16 CrossRef.
- I. Diez and R. H. Ras, Nanoscale, 2011, 3, 1963–1970 RSC.
- Z. Wang, H. F. Su, C. H. Tung, D. Sun and L. S. Zheng, Nat. Commun., 2018, 9, 2094 CrossRef PubMed.
- M. Qu, F.-Q. Zhang, D.-H. Wang, H. Li, J.-J. Hou and X.-M. Zhang, Angew. Chem. Int. Ed., 2020, 132, 6569–6574 CrossRef.
- A. Dhakshinamoorthy, I. E. Adell, A. Primo and H. Garcia, ACS Sustain. Chem. Eng., 2017, 5, 2400–2462 CrossRef CAS.
- Y. Kikukawa, Y. Kuroda, K. Yamaguchi and N. Mizuno, Angew. Chem. Int. Ed., 2012, 51, 2434–2437 CrossRef CAS PubMed.
- H. Wang, X. Qiao, J. Chen and S. Ding, Colloids Surf. A Physicochem. Eng. Asp., 2005, 256, 111–115 CrossRef CAS.
Footnotes |
† Electronic supplementary information (ESI) available. CCDC 1981614. For ESI and crystallographic data in CIF or other electronic format see DOI: 10.1039/d0ra01244k |
‡ These authors contributed equally to this work. |
|
This journal is © The Royal Society of Chemistry 2020 |
Click here to see how this site uses Cookies. View our privacy policy here.