DOI:
10.1039/D0RA00658K
(Paper)
RSC Adv., 2020,
10, 8866-8879
Efficient fluorescent OLEDS based on assistant acceptor modulated HLCT emissive state for enhancing singlet exciton utilization†
Received
21st January 2020
, Accepted 20th February 2020
First published on 2nd March 2020
Abstract
Phenylamine phenanthroimidazole based bipolar compounds with donor–acceptor (D–A) architecture namely, 4-(1-(2,3-dihydrobenzo[b][1,4]dioxin-5-yl)-6,9-di(pyren-4-yl)-1H-phenanthro[9,10-d]imidazol-2-yl)-N,N-diphenylaniline (DDPPPA) and 4′-(1-(2,3-dihydrobenzo[b][1,4]dioxin-5-yl)-6,9-di(pyren-4-yl)-1H-phenanthro[9,10-d]imidazol-2-yl)-N,N-diphenyl-[1,1′-biphenyl]-4-amine (DDPBA) have been synthesized with highly fluorescent pyrene moieties at C6- and C9-positions. The C6 and C9 modification enhanced the thermal, photochemical and electroluminescent properties. Both molecules were employed as blue emitters in non doped organic light emitting devices (OLEDs) and show high performances due to hybridized local and charge-transfer properties. An OLED with DDPPPA/DDPBA emissive layer shows deep-blue emission with maximum external quantum efficiency (ηex), current efficiency (ηc) and power efficiency (ηp) of 5.7/6.0%, 10.5/12.0 cd A−1 and 8.3/9.2 lm W−1, respectively. Both devices show high singlet exciton utilizing efficiency (ηs) of DDPPPA-31.33% and DDPBA-35.29%. The doped device m-MTDATA:DDPPPA/m-MTDATA:DDPBA shows maximum efficiencies of ηc −7.4/8.23 cd A−1; ηp −5.8/6.13 lm W−1; ηex −4.72/5.63% (5 wt%):ηc −8.36/9.15 cd A−1; ηp −6.32/6.65 lm W−1; ηex −4.86/5.45% (10 wt%):ηc −9.58/10.02 cd A−1; ηp −7.8/8.25 lm W−1; ηex −5.96/6.25% (20 wt%). The doped device based on TAPC host TAPC:DDPPPA/TAPC:DDPBA exhibits maximum efficiencies of ηc −9.60/10.03 cd A−1; ηp −7.81/8.26 lm W−1; ηex −5.96/6.25% (20 wt%).
1. Introduction
Efficient deep blue emitters are very important in flat-panel displays because of reduced power consumption1–3 and to sensitize the dopants for white light emission,4–6 however, development of blue OLEDs lags behind that of green and red counterparts due to the wider energy gap which leads to unbalanced charge injection/transportation results in poor efficiency.7–11 Phenanthroimidazole (PI) with pyrrole (N1) and pyridine-like (N3) nitrogen atoms exhibits bipolar characteristics, unmodified PI hinders its application as a potential EL material.12,13 Therefore, efforts have been made to address these issues by substitution at various positions: C2 substitution induced extended conjugation leads to intramolecular charge transfer (ICT),14–16 however, N1 substitution does not promote significant photophysical change because of perpendicular configuration17 and hence, PI derivatives have been designed to have bulky moieties at N1 to control intermolecular interaction in the solid state. The bulky side capping could prevent interactions thereby enhancing the formation of amorphous film and exhibit high PLQY. Therefore it is aimed to incorporate bulky side capping, dihydrobenzodioxin at imidazole nitrogen to reduce packing between the molecules which limits emission quenching leading to enhanced quantum efficiency.18–22 Designing donor–acceptor (D–A) structure is an effective solution for triplet utilization by reducing exchange energy (J) of exciton and increasing rate of reverse intersystem crossing (RISC):23–26 |
 | (1) |
where, |
 | (2) |
where, |rh−e| – distance between hole (h+) and electron (e−). Large |rh−e| CT excited state afford ΔEST to ∼zero which induce fast RISC for triplet harvesting27–33 material exhibit high external quantum efficiency (ηex). Absolute CT excited state materials show poor photoluminescent efficiency (ηPL) since large charge separation |rh−e| results minimised overlap between hole and electron moieties
which leads to decreasing oscillator strength (f) of emissive excited state: |
 | (3) |
Therefore, it is important to construct a material to show high PLQY and exciton utilisation efficiency (ηs) in one excited state. D–A compounds with HLCT (hot exciton model) emissive state show high-exciton utilization efficiency.34–40 Mixing of LE and CT states are possible when LE as well as CT states are closure in energy, Ψ(S1) = Ψ(LE) + λ × Ψ(CT); λ = |〈ΨLE|H|ΨCT〉/ECT − ELE. The low-lying higher % LE state provides high radiative rate (kr) leads to high photoluminescence efficiency (ηPL) whereas low-lying higher % CT state provides high ηs through reverse intersystem crossing (RISC) with hot exciton model.41 The larger energy gap between T2 and T1 states reduced internal conversion (IC)
results hot RISC
than cold RISC
:42 hot exciton with HLCT increases ηEQE due to coexistence of high ηPL and high ηs. The ηEQE and ηs can be calculated by, ηEQE = ηout × ηIQE = ηout × ηrec × ηγ × ΦPL; ηs = ηout × ηrec × ηPL,43,44, [ηout – light out-coupling efficiency (20%), ηrec – product of the charge recombination efficiency (100%), ηγ – efficiency of radiative exciton production (25%) and ΦPL – photoluminescence quantum yield].
To date, numerous reports have been published on phenanthroimidazole with C2 and N1 substitution, however, substitution at C6 and C9 positions have not been widely studied.45 Herein, we have designed and synthesized phenanthroimidazole with pyrene substituent at C6 and C9 positions which provides moderate conjugation compared to C2 modification. Photophysical study demonstrates that character transfer of DDPPPA and DDPBA increase as increasing the bulkiness of C6 and C9 substituent. The blue device with DDPBA emissive layer show sky blue emission (470 nm, CIE-0.15, 0.10) with maximum efficiencies: ηc −12.0 cd A−1; ηp −9.2 lm W−1; ηex −6.0% at 2.2 V. The doped device m-MTDATA:DDPPPA/m-MTDATA:DDPBA show maximum efficiencies of ηc −7.4/8.23 cd A−1; ηp −5.8/6.13 lm W−1; ηex −4.72/5.63% (5 wt%):ηc −8.36/9.15 cd A−1; ηp −6.32/6.65 lm W−1; ηex −4.86/5.45% (10 wt%):ηc −9.58/10.02 cd A−1; ηp −7.8/8.25 lm W−1; ηex −5.96/6.25% (20 wt%). The doped device based on TAPC host TAPC:DDPPPA/TAPC:DDPBA exhibit maximum efficiencies of ηc −9.60/10.03 cd A−1; ηp −7.81/8.26 lm W−1; ηex −5.96/6.25% (20 wt%).
2. Experimental section
2.1. Measurements
1H and 13C NMR measurements were recorded on Bruker 400 MHz spectrometer using TMS as internal standard and mass spectra was recorded on Agilent LCMS VL SD. The UV-optical spectra were measured on Lambda 35 PerkinElmer (solution)/Lambda 35 spectrophotometer (RSA-PE-20) (film). The emission spectra were recorded with PerkinElmer LS55 spectrometer. The quantum yield was measured with fluorescence spectrometer Model-F7100 with integrating sphere. The decomposition temperature (Td) and Tg (glass transition temperature) was measured with PerkinElmer thermal analysis system (10 °C min−1; N2 flow rate – 100 ml min−1) and NETZSCH (DSC-204) (10 °C min−1; N2 atmosphere), respectively. Fluorescence lifetime was estimated by time correlated single-photon counting (TCSPC) method on Horiba Fluorocube-01-NL lifetime system: nano LED is excitation source with TBX-PS is detector; DAS6 software was employed to analyze the decay by reconvolution method. Oxidation potential of emissive materials was measured from potentiostat electrochemical analyzer (CHI 630A). Ferrocene was used as internal standard with HOMO of −4.80 eV and 0.1 M tetrabutylammoniumperchlorate in CH2Cl2 as supporting electrolyte.
2.2. Synthesis of materials
2.2.1. 4-(6,9-Dibromo-1-(2,3-dihydrobenzo[b][1,4]dioxin-5-yl)-1H-phenanthro[9,10-d]imidazol-2-yl)-N,N-diphenylaniline (DPPB). A mixture of 3,6-dibromophenanthrene-9,10-dione (3 g, 8.2 mmol), 4-(diphenylamino)benzaldehyde (3.38 g, 12.3 mmol), 1,4-benzodioxane-6-amine (4.65 g, 50 mmol) and ammonium acetate (7.85 g, 101.8 mmol) in glacial acetic acid (40 ml) was refluxed for 12 h (N2 stream). The yellow solution was poured into ethanol and the separated solid was washed with methanol and dried. The solid was purified by column chromatography (hexane
:
ethylacetate) yield: 66%. 1H NMR (400 MHz, CDCl3): 4.36 (s, 4H), 6.46–6.52 (m, 6H), 6.62–6.7 (m, 5H), 7.01 (q, 2H), 7.23 (d, 2H), 7.71 (s, 4H), 7.82–7.93 (m, 10H), 8.04–8.18 (m, 8H), 9.15 (s, 2H). 13C NMR (CDCl3, 100 MHz): 64.3, 114.5, 114.6, 118.1, 122.0, 122.3, 122.7, 124.0, 124.9, 125.1, 126.0, 126.3, 126.6, 128.0, 128.3, 128.4, 129.7, 132.0, 133.3, 133.4, 137.0, 137.7, 141.0, 147.6, 149.4. MS: m/z. 753.06 [M+]; calcd 753.04.
2.2.2 4-(1-(2,3-Dihydrobenzo[b][1,4]dioxin-5-yl)-6,9-di(pyren-4-yl)-1H-phenanthro[9,10-d]imidazol-2-yl)-N,N-diphenylaniline (DDPPPA). A mixture of DPPB (1.5 g, 2 mmol), pyren-1-yl-1-boronic acid (0.97 g, 4.4 mmol), Pd(PPh3)4 (0.26 g, 0.40 mmol) and aqueous Na2CO3 (2 M, 8 ml) in toluene (30 ml) and ethanol (6 ml) was refluxed (36 h; N2 stream). The solution was cooled to room temperature and extracted with dichloromethane. The residue was purified by column chromatography (hexane
:
ethylacetate) to obtain light yellow powder. Yield: 69%. 1H NMR (400 MHz, CDCl3): 4.36 (s, 4H), 6.52–6.46 (m, 6H), 6.62–6.7 (m, 5H), 7.01 (t, 4H), 7.23 (d, 2H), 7.71 (d, 2H), 7.82–7.93 (m, 10H), 8.04–8.18 (m, 8H), 9.15 (d, 2H). 13C NMR (CDCl3, 100 MHz): 64.3, 114.5, 114.6, 118.1, 121.9, 122.0, 122.3, 122.7, 123.2, 124.9, 125.1, 125.4, 125.7, 126.3, 126.5, 126.6, 128.3, 128.4, 129.7, 132.0, 133.4, 134.3, 137.0, 137.7, 141.0, 147.6, 149.4. MS: m/z. 996.16 [M+]; calcd 996.19.
2.2.3. 4′-(6,9-Dibromo-1-(2,3-dihydrobenzo[b][1,4]dioxin-5-yl)-1H-phenanthro[9,10-d]imidazol-2-yl)-N,N-diphenyl-[1,1′-biphenyl]-4-amine (DPDA). A mixture of 3,6-dibromophenanthrene-9,10-dione (3 g, 8.2 mmol), 4′-(diphenylamino)-[1,1′-biphenyl]-4-carbaldehyde (3.38 g, 12.3 mmol), 1,4-benzodioxane-6-amine (4.65 g, 50 mmol) and ammonium acetate (7.85 g, 101.8 mmol) in glacial acetic acid (40 ml) was refluxed (12 h; N2 stream). The yellow coloured solution was poured into ethanol and the solid was purified by column chromatography (hexane
:
ethylacetate) yield: 52%. 1H NMR (400 MHz, CDCl3, ppm): 4.36 (s, 4H), 6.46–6.52 (m, 6H), 6.62–6.7 (m, 5H), 7.01 (q, 4H), 7.23 (d, 2H), 7.71 (s, 4H), 7.82–7.93 (m, 10H), 8.04–8.18 (m, 8H), 9.15 (s, 2H). 13C NMR (CDCl3, 100 MHz): 64.3, 114.5, 114.6, 118.1, 122.0, 122.3, 122.7, 124.0, 124.9, 125.1, 126.0, 126.3, 126.6, 128.0, 128.3, 128.4, 129.7, 132.0, 133.3, 133.4, 137.0, 137.7, 141.0, 147.6, 149.4. MS: m/z. 995.38 [M]+; calcd: 995.35. Anal. calcd (%) for C73H45N3O4: C, 88.02; H, 4.55; N, 4.22. Found: C, 87.97; H, 4.60; N, 4.18.
2.2.4. 4′-(1-(2,3-Dihydrobenzo[b][1,4]dioxin-5-yl)-6,9-di(pyren-4-yl)-1H-henanthro[9,10-d]imidazol-2-yl)-N,N-diphenyl-[1,1′-biphenyl]-4-amine (DDPBA). A mixture of DPDA (1.5 g, 2 mmol), pyren-1-yl-1-boronic acid (0.97 g, 4.4 mmol), Pd(PPh3)4 (0.26 g, 0.40 mmol) and aqueous Na2CO3 (2 M, 8 ml) in a mixture of toluene (30 ml) and ethanol (6 ml) was refluxed (36 h; N2 stream). The solution was extracted with dichloromethane and the residue was purified by column chromatography (hexane
:
ethylacetate) yield: 69%. 1H NMR (400 MHz, CDCl3): 4.36 (s, 4H), 6.46–6.52 (m, 6H), 6.62–6.7 (m, 5H), 7.01 (q, 4H), 7.23 (d, 2H), 7.54 (s, 4H), 7.71 (d, 4H), 7.82–7.93 (m, 10H), 8.04–8.18 (m, 8H), 9.15 (d, 2H). 13C NMR (CDCl3, 100 MHz): 64.3, 114.5, 114.6, 118.1, 121.9, 122.0, 122.7, 123.2, 124.9, 125.2, 125.4, 126.3, 126.5, 128.0, 128.3, 128.4, 128.8, 129.6, 129.7, 130.9, 132.0, 133.2, 133.3, 134.4, 136.5, 137.7, 139.9, 141.0, 147.6, 149.4. MS: m/z. 1071.44 [M+]; calcd 1071.38.
2.3. Device fabrication and measurement
ITO glass (resistance 20 Ω sq−1) were cleaned with acetone, deionized water and isopropanol, dried (120 °C) followed by UV–ozone treatment (20 min) and transferred into deposition system. The devices were fabricated by the multiple source beam deposition method in a vacuum at a pressure of 4 × 10−5 mbar. Evaporation rates of 2–4 Å s−1 (organic materials) and 0.1 and 4 Å s−1 for LiF and metal electrodes were applied, respectively. The thickness of each deposition layer was monitored with quartz crystal thickness monitor. The EL measurement with CIE coordinates was recorded with USB-650-VIS-NIR spectrometer (Ocean Optics, Inc, USA). The current density–voltage–luminance (J–V–L) characteristics was performed using source meter (Keithley 2450) equipped with LS-110 light intensity meter. The ηex (external quantum efficiency) was determined from luminance, current density and EL spectrum assuming Lambertian distribution.
2.4. Computational details
The ground (S0) (DFT)/excited
(TD-DFT) states of emissive materials were analyzed by Gaussian 09 program46 and multifunctional wavefunction analyzer (Multiwfn).46 The natural transition orbitals (HONTOs & LUNTOs) with hole-particle contribution were studied in detail. The TDM (transition density matrix) map of emissive materials was analyzed to support HLCT character.
3. Results and discussion
The synthetic route for the emissive materials is displayed in Scheme S1:† efficient blue emitters namely, 4-(1-(2,3-dihydrobenzo[b][1,4]dioxin-5-yl)-6,9-di(pyren-4-yl)-1H-phenanthro[9,10-d]imidazol-2-yl)-N,N-diphenylaniline (DDPPPA) and 4′-(1-(2,3-dihydrobenzo[b][1,4]dioxin-5-yl)-6,9-di(pyren-4-yl)-1H-phenanthro[9,10-d]imidazol-2-yl)-N,N-diphenyl-[1,1′-biphenyl]-4-amine (DDPBA) were synthesized by Suzuki-coupling reaction with appreciable yield and characterized by spectral techniques.
3.1. Thermal and electrochemical properties
The twisting angle between pyrene and phenanthroimidazole plane of D–π–A structured DDPPPA and DDPBA is about ∼55° which effectively suppress the conjugation and intermolecular π–π stacking. The twisted molecular architecture has been increased the thermal stability of DDPPPA and DDPBA and the excellent thermal stability was analyzed by high decomposition temperature (Td, 5% weight loss). The high Td (556 °C-DDPPPA; 562 °C-DDPBA) reveals that these compounds would be capable of enduring vacuum thermal sublimation process. Both compounds show high glass transition temperature (Tg: 201 °C-DDPPPA; 223 °C-DDPBA) indicating that pyrene substituent at C6 and C9 positions and bulky substituent at N-side coupling of rigid phenanthroimidazole enhanced their morphological stability (Table 1 and Fig. 1). The interaction of substituent at C2- and N1 of phenanthroimidazole core improved Tg and induced more condensed molecular packing. The higher Tg reveal that morphologically stable amorphous film will form on thermal evaporation which reduced the phase separation on heating and improving the lifetime of devices. The thermal morphological stability of thin film of these compounds was examined by atomic-force microscopy at 30 °C and 90 °C for 12 h. The root-mean-square (0.24 nm-DDPPPA; 0.28 nm-DDPBA) reveal absence of remarkable surface modification before and after annealing which further supports the suitability of these materials for fabrication.
Table 1 Optical and thermal properties of DDPPPA and DDPBA
Characteristics |
DDPPPA |
DDPBA |
λab (nm) (sol) |
248, 275, 342 |
240, 268, 334 |
λem (nm) (sol/film) |
480/456 |
469/448 |
Tg/Td (°C) |
201/556 |
223/562 |
ϕ (soln/film) |
96/83 |
98/85 |
HOMO/LUMO (eV) |
−5.22/−2.39 |
−5.20/−2.41 |
Eg (eV) |
−2.83 |
−2.79 |
τ (ns) |
1.38 |
1.13 |
kr × 108 (s−1) |
0.69 |
0.03 |
knr × 108 (s−1) |
0.87 |
0.01 |
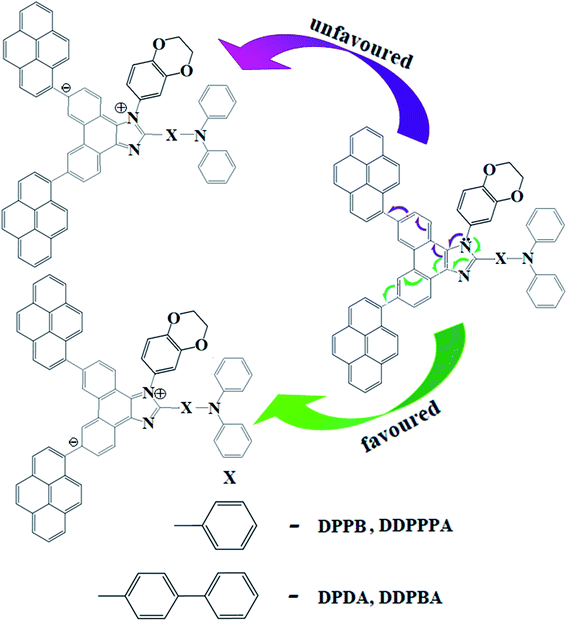 |
| Fig. 1 Favoured and unfavoured configurations of blue emitters. | |
The DDPPPA and DDPBA shows quasi-reversible oxidation and reduction process with an oxidation onset potential (Eonset) of 0.82 (DDPPPA) and 0.80 V (DDPBA), implying that these materials are electrochemically stable and are bipolar carrier-transporting materials.47 The EHOMO[−(Eonset + 4.8 − Eferrocene)] eV and ELUMO[EHOMO − 1239/λonset] have been calculated as DDPPPA (HOMO −5.22 eV; LUMO −2.39 eV) and DDPBA (HOMO −5.20 eV; LUMO −2.41 eV).
3.2. Photophysical properties
The absorption and emission of DDPPPA and DDPBA was studied with various solvents and also in film (Fig. 1). The strong absorption around 236 nm can be attributed to π–π* transition of phenyl ring attached to N-atom in imidazole ring.48 The absorption around 268 nm can be ascribed to absorption of diphenylamine unit.49 The extinction coefficient (εmax) of D–π–A molecules is due to increase of conjugation length:50,51 DDPPPA and DDPBA show very strong absorption at 240 nm (εmax = 41
666 cm−1 M−1) and 236 nm (εmax = 42
372 cm−1 M−1) due to ICT transition from donor to acceptor (Fig. 2). The film state of blue emissive materials show absorption at 244 nm (DDPPPA) and 240 nm (DDPBA) and the small shift shows the existence of weak π–π* intermolecular stacking.52 The shoulder peak at longer wavelength around 334 nm might be attributed to an intramolecular charge transfer (ICT) from electron donating diphenylamine to electron-withdrawing imidazole unit.53 The solution/film PL spectra of DDPPPA and DDPBA show emission maxima at 480/456 and 469/448 nm, respectively (Fig. 2). These compounds DDPPPA and DDPBA show shorter wavelength emission relative to C2-substituted compounds. Overall, substitution at C6 and C9 positions of phenanthroimidazole ring in DDPPPA and DDPBA induces moderate π-conjugated extension comparing to C2-modified ones.
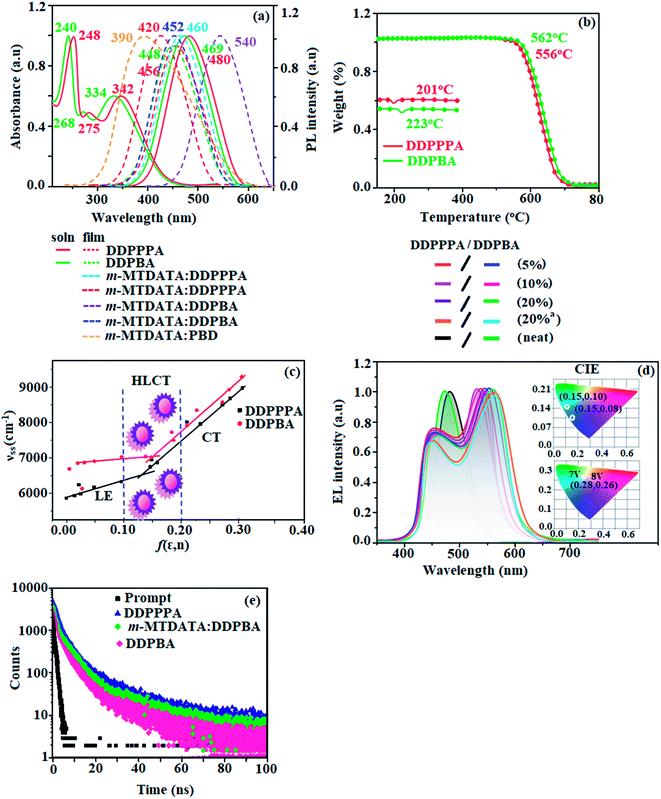 |
| Fig. 2 (a) Normalized absorption and emission spectra; (b) TGA and DSC; (c) Lippert–Mataga plot; (d) decay curve; (e) EL spectra (inset: CIE coordinates) of DDPPPA and DDPBA. | |
The solvatochromic effect using Lippert–Mataga plot has been displayed in Fig. 2. When solvent polarity increased the blue emitters exhibit a larger red shift which supports the charge transfer in these molecules. The emission spectra gradually broadened with large red shift of 30 nm (DDPPPA) and 65 nm (DDPBA) reveal that their excited state possess strong CT characteristics (Fig. S1†).54 The red shifted emission is due to twisted conformation of DDPPPA and DDPBA which leads to easier charge transfer from donor to accept via aryl linker. The intramolecular charge transfer is further confirmed by molecular electrostatic potential (MEP) (Fig. 2).55,56 Interaction between solvent and dipole moment of solute can be described by the Lippert–Mataga model.57 hc(
abs −
flu) = hc(hcΔνacabs − hcΔνacflu) + 2(μe − μg)2/ao3[(ε − 1/2ε + 1) − ½(n2 − 1/2n2 + 1)] [μg and μe – ground state and excited state dipole moment,
abs and Δνacabs − solvent-equilibrated absorption maxima (λabs) and extrapolated to gas phase,
flu and Δνacflu – solvent equilibrated fluorescence maxima (λemi) and extrapolated to gas-phase, respectively, ao – onsager cavity and ε and n – solvent dielectric constant and refractive index, respectively].
The ground state dipole moment (μg) of blue emitting materials, DDPPPA and DDPBA could be estimated from DFT calculation as 8.67 and 7.45 D, respectively which is attributed by local exciton (LE) transition. From Lippert–Mataga plot (Fig. 2, Table S1 – DDPPPA and Table S2 – DDPBA†), the excited state dipole moment (μe) in high polar solvents is calculated to be 25.6 and 27.3 D, respectively. A transformation in the slope of the fitted line is observed between ether (f = 0.10) and ethyl acetate (f = 0.20) solvents. This implies that these blue emitters show HLCT excited state. The fluorescent solvatochromic experiments and non-linear relationship of Stokes shift with solvent polarity indicate that our new born molecules DDPPPA and DDPBA possess intercrossed excited state of LE and CT. The large μe in high polar medium is in close with μe of charge-transfer molecule, 4-(N,N-dimethylamino)benzonitrile (23.0 D). All these results show that CT dominates in more polar medium (f ≥ 0.2) and LE dominates in low polar solvents (f ≤ 0.1) and there is mixed contribution of LE and CT in medium polar solvents. The new born blue emitters show high quantum yield (solution/film) of DDPPPA (0.96/0.83) and DDPBA (0.98/0.85) and high fluorescence yield is essential for efficient blue OLEDs (Table 1). It is unique that CT material shows efficient deep-blue emission; the co-emission from LE and CT (intercrossed CT and LE state) is likely to be the reason for high fluorescence yield. The oscillator strength (f) for λabs/λemi of DDPPPA (gas phase – 372 (f – 1.5283)/383 (f – 1.7692); CHCl3 – 368 (f – 1.7982)/412 (f – 2.0462)) and DDPBA [gas phase – 380 (f – 1.5846)/392 (f – 1.8146); CHCl3 – 370 (f – 1.8268)/422 (f – 1.9432)] reveal that oscillator strength of these compounds in CHCl3 is high when compared to gas phase which reveal the higher luminescence of intercrossed excited state in CHCl3.
3.3. Theoretical calculation
To gain a better insight into the electronic structure of DDPPPA and DDPBA, DFT calculations were carried out at B3LYP/6-31G (d) level. As shown in (Fig. 3), in DDPPPA and DDPBA, HOMO orbital was partially extends to pyrene plane whereas due to stronger electron withdrawing ability of pyrene, phenanthroimidazole unit makes much less contribution to LUMO. The LUMO orbital localized on C9-pyrene with less contribution from C6 pyrene and this asymmetry originates from the difference in LUMO contribution of C6 and C9. In C4 and C7-modified benzimidazole asymmetry compounds having different reactivity difference at C4 and C7 brominated sites in Suzuki coupling reaction due to two different nitrogen atoms in imidazole ring,57 thus, similar properties can be expected in phenanthroimidazole: obey Huckel's (4n + 2) rule (Fig. 3). The lone pair electrons at N1 involved in the formation of conjugated system whereas the lone pair on N3 has no contribution. The conjugated lone pair electrons can be transferred toward C6 or C9: electron migration toward C6 is more favourable since it results in largest charge-separated distance than C9 (green arrow) (Fig. 3). This preferred electronic structure leads to charge accumulation at C6 position. This unique electronic structure induced the electron delocalisation on HOMO and LUMO of DDPPPA and DDPBA at C6 and C9 substitutions, respectively and hence, this asymmetry enhanced the electron injection, however, there is small overlap in HOMO and LUMO orbitals. This is more likely to endow DDPPPA and DDPBA with bipolar charge transporting ability become more efficient emitters in OLEDs.58 The HONTOs and LUNTOs of DDPPPA (Fig. S2 and Table S3†) and DDPBA (Fig. S3 and Table S4†) exhibit a hybrid splitting state character from interstate coupling of LE and CT levels (Tables S5 and S6 – DDPPPA and Tables S7 and S8 – DDPBA†). The hole contour on TPA is in the opposite phases between S1 and S2 states whereas the particle contour on phenanthroimidazole moiety is same between S1 and S2 states for DDPPPA and DDPBA. This reveal that the interstate hybridization coupling between LE and CT state wave function: ΨS1/S2 = cLEΨLE ± cCTΨCT. The % CT of DDPPPA and DDPBA increases as increasing the aromatic fragment size and also partially influenced by steric hindrance. As increasing % LE in S1 state of DDPPPA (LE ∼ 30%) and DDPBA (LE ∼ 40%) these emitters exhibit higher photoluminescence efficiency (ηPL). The formation of single emissive state can be analyzed through excitation energies of LE and CT states. A large energy gap between T1 and T2 of DDPPPA (0.75 eV) and DDPBA (1.07 eV) arising from the same phenanthroimidazole acceptor group and the energy gap between T1 and T2 of DDPBA is larger than DDPPPA (Fig. S4†).59,60 A very small ΔEST ≈ 0 between S1 and T2 states facilitating RISC (T2 → S1) process in both DDPPPA (Tables S9 and S10†) and DDPBA (Tables S11 and S12†) as a result of HLCT character (Fig. S5†). Thus, compared with DDPPPA, DDPBA exhibits high photoluminescence efficiency (ηPL), high exciton utilisation efficiency (ηs) and high external quantum efficiency (ηEQE) as a result of increased LE component in S1 state.
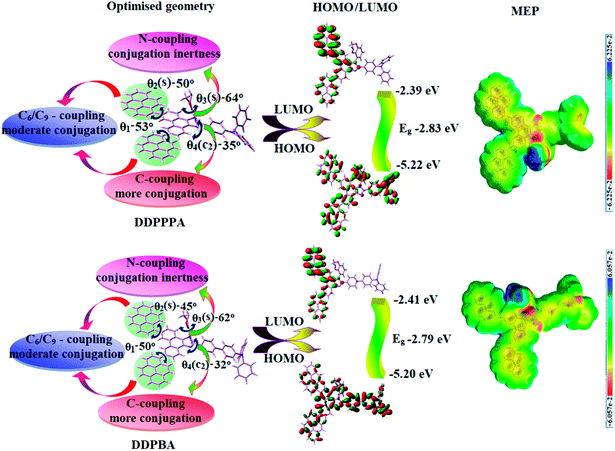 |
| Fig. 3 Optimised geometry with dihedral angles, HOMO–LUMO map and MEP of DDPPPA and DDPBA. | |
Similar hole–electron wave function between S1 and S2 in both DDPPPA and DDPBA indicates quasi-equivalent hybridization between LE and CT states due to isoenergies of initial LE and CT states (Fig. S5†). Therefore, degree of hybridization between LE and CT states depend not only initial ELE–ECT energy gap but also their interstate coupling strength.61 Compared with non-equivalent hybridization, quasi-equivalent hybridization leads to high ηPL and high ηs to maximize EL efficiency of fluorescent OLED materials due to more balanced LE and CT components in HLCT state of DDPPPA and DDPBA. In DDPPPA and DDPBA, the LE state is stabilized than CT state and energy gap (ES2–ES1) is small when compared with their parent compounds results effective quasi hybridization (Fig. S5†) and improves OLED efficiency. Composition of HLCT can be discussed from wave function of electron–hole pairs transition density matrix (TDM) and plot them in two-dimension colour-filled map (Fig. S6 – DDPPPA and Fig. S7 – DDPBA†). The diagonal region represents LE component and off-diagonal region shows CT component. Upon excitation, electron is transferred from donor and localized on acceptor: depending upon intramolecular geometrical and electronic coupling, transferred electron is delocalized from the region of nearby donor to the vicinity of acceptor. This effect can be qualitatively studied by analyzing electron density distribution at ground and excited states. Computed electron–hole properties, distance between hole and electron, transition density, H and t indexes and RMSD of electron and hole of DDPPPA and DDPBA are displayed in Tables S9–S12,† respectively.
The integral value of hole and electron of DDPPPA (Table S5†) is higher than DDPBA (Table S6†) with transition density. The integral overlap of hole–electron distribution (S) is a measure of spatial separation of hole and electron. The integral overlap (S) of hole and electron and distance (D) between centroids of hole and electron evidences the existence of LE and CT states (Tables S5 and S7†). The emitters DDPPPA and DDPBA show small D and high S, however, small D and high S of DDPPPA on comparison with DDPBA indicates higher charge transfer (CT) for DDPPPA. The variation of dipole moment with respect to S0 state is outputted which is directly evaluated based on the position of centroid of hole and electron. RMSD of hole or electron characterizes their distribution breadth: RMSD of both electron and hole in DDPPPA (Tables S9 and S10†) and DDPBA (Tables S11 and S12†) is higher in Z direction indicates electron and hole distribution is much broader in Z direction whereas RMSD of electron and hole of DDPPPA is higher than DDPBA. The overlap between the region of density depletion and increment have been visualised by using two centroids of charges (eqn (S9) and (S10)†). Using this arbitrarily condensed function, computed overlap of ρ+ and ρ− regions for DDPPPA and DDPBA is 0.9843 and 0.9985, respectively (Table S13†). Similarly, H index of DDPPPA is higher than DDPBA. The CT index, i.e., t index difference between DCT and H index is another measure of the separation of hole–electron (eqn (S15) and (S16)†); t index of DDPPPA is higher than DDPBA. The DCT/μCT of DDPPPA and DDPBA was calculated to be 1.201/0.003 and 0.429/59.23, respectively (Fig. S8 and Table S13†). For both DDPPPA and DDPBA, the non-zero t is negative in all directions: overlap of hole and electron is very severe (Fig. S8†) and eign value is greater than 0.94, supports the hybridization with dominant excitation pair in term of 92% of transition.
The hybridization and HLCT is further evidenced by Δr index (Table S14 – DDPPPA and Table S15 – DDPBA†). The Δr index (eqn (S1)†) is average of hole (h+)–electron (e−) distance (dh+–e−) upon excitation which implies the nature of the excitation type, LE or CT: valence excitation (LE) is related to short distances (dh+–e−) while larger distances (dh+–e−) related to CT excitation. The triplet exciton is transformed to singlet exciton in DDPPPA and DDPBA via RISC process with high energy excited state (hot CT channel)62 which is beneficial for triplet exciton conversion in electroluminescence process without delayed fluorescence (Fig. S4†). The CT excitons are formed with weak binding energy (Eb) on higher excited states.63 As a result, the exciton utilization (ηs) can be harvested in DDPPPA and DDPBA like phosphorescent materials. The quasi-equivalent hybridized materials DDPPPA and DDPBA show excellent device performances due to fine modulation in excited states: enhanced LE component and hybridization between LE and CT components results high ηPL and high ηs and enhanced OLEDs performances (Table 2). These compounds exhibit blue emission with high quantum yield (solution/film) of DDPPPA (0.96/0.83) and DDPBA (0.98/0.85) and high fluorescence efficiencies are essential for efficient blue OLEDs. The ΔEST of these hosts are relatively small which arises from spatially separated HOMO and LUMO of those hosts. The small ΔEST are advantageous for efficient energy transfer from triplet excited state of hosts to green and red phosphorescent emitters.64
Table 2 Comparative non-doped and doped device efficiencies
Emitters |
Concentration |
Von (V) |
L (cd m−2) |
EL (nm) |
ηc (cd A−1) |
ηp (lm W−1) |
ηex (%) |
ηIQE (%) |
ηs (%) |
CIE (x, y) |
ITO/NPB (40 nm)/TAPC:DDPPPA or TAPC:DDPBA (20 nm)/TPBi(25 nm)/LiF (1 nm)/Al (100 nm). |
DDPPPA |
5% |
4.6 |
8123 |
448, 536 |
7.40 |
5.8 |
4.72 |
23 |
25.8 |
0.28, 0.28 |
10% |
4.2 |
9082 |
448, 535 |
8.36 |
6.32 |
4.86 |
24 |
26.9 |
0.28, 0.27 |
20% |
3.8 |
11 260 |
448, 537 |
9.58 |
7.80 |
5.96 |
29 |
32.5 |
0.28, 0.26 |
20%a |
3.8 |
11 265 |
448, 540 |
9.60 |
7.81 |
5.96 |
29 |
32.5 |
0.28, 0.26 |
Non-doped |
2.3 |
10 582 |
480 |
10.50 |
8.30 |
5.70 |
28 |
31.4 |
0.15, 0.08 |
DDPBA |
5% |
4.5 |
10 462 |
488, 538 |
8.23 |
6.13 |
5.63 |
28 |
31.1 |
0.28, 0.28 |
10% |
4.0 |
11 862 |
488, 537 |
9.15 |
6.65 |
5.45 |
27 |
30.0 |
0.28, 0.27 |
20% |
3.2 |
14 021 |
448, 539 |
10.02 |
8.25 |
6.25 |
31 |
34.4 |
0.28, 0.26 |
20%a |
3.2 |
13 897 |
447, 540 |
10.03 |
8.26 |
6.25 |
31 |
34.4 |
0.28, 0.26 |
Non-doped |
2.2 |
12 634 |
470 |
12.00 |
9.20 |
6.00 |
30 |
33.3 |
0.15, 0.10 |
The potential energy surfaces (PES) have been plotted as a function of twist angle between C2 substituent and phenanthroimidazole core in gas phase and different polarity solvents (Fig. S9†). In gas phase, it is impossible for S3 mixing with S1 state as a result of large energy gap between them. An increasing solvent polarity, the large dipole moment of S3 state leads to a significant energetic stabilization. In low polarity solvent (hexane), S3 state can intercross with S1 state whereas in high polarity solvent (acetonitrile), S3 state energy decreases sharply and becomes lowest excited state. In moderate polar solvents (chloroform), the energetic closeness results in an enhanced mixing of S3 with S1. Therefore, in low polarity, S1 state is dominated by LE character; in moderate polarity, S1 state is dominated by mixing LE and CT character; in high polarity, S1 state is dominated by CT character.
3.4. Electroluminescent properties
To understand the carrier transport abilities of DDPPPA or DDPBA, hole-only and electron-only devices have been fabricated with configuration of ITO/NPB (8 nm)/DDPPPA or DDPBA (40 nm)/NPB (8 nm)/Al (100 nm) (hole-only device): NPB (LUMO: −2.3 eV) in the hole-only device can prevent electron injection from Al cathode (Ef – 4.3 eV)65 and ITO/TPBi (8 nm)/DDPPPA or DDPBA (40 nm)/TPBi (8 nm)/LiF (1 nm)/Al (100 nm) (electron-only device): TPBi (HOMO: −6.2 eV) in the electron-only device with ITO (Ef – 4.8 eV) as the anode can prevent hole injection.66 The current density versus voltage characteristics of hole-only and electron-only devices was displayed in Fig. 4. The hole-only and electron-only devices show the same current density values at same voltage indicating that these compounds are bipolar materials. The electron current density of DDPPPA or DDPBA based device is higher than CBP-based device (Fig. 4) which reveals that these compounds are bipolar-transporting materials with effectively transporting electrons and or holes than CBP.67–69
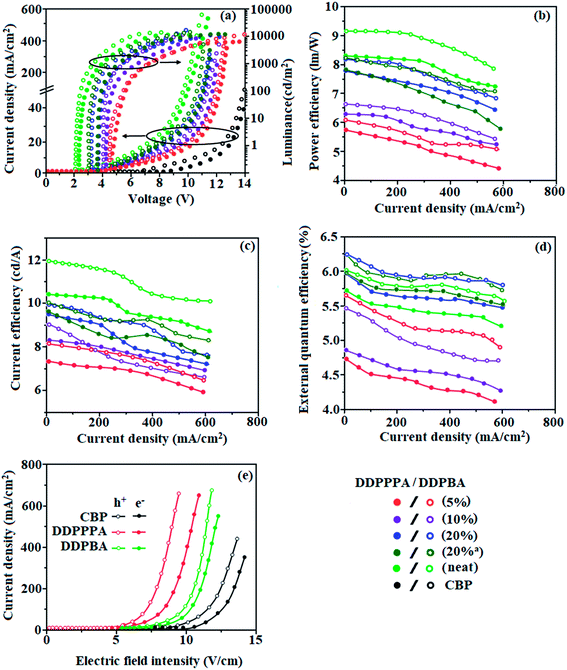 |
| Fig. 4 Device efficiencies: (a) luminance/current density–voltage; (b) power efficiency–current density; (c) current efficiency–current density; (d) external quantum efficiency–current density and (e) current density–electrical field density of DDPPPA and DDPBA. | |
Two non-doped OLEDs were fabricated: ITO/NPB (70 nm)/TCTA (5 nm)/DDPPPA and DDPBA (30 nm)/TPBI (40 nm)/LiF (1 nm)/Al (150 nm) NPB – hole-transporting layer (HTL), TPBI – electron transporting layer (ETL) as well as hole blocking layer, DDPPPA and DDPBA – emitting layer (EML) and ITO and LiF/Al are used as anode and cathode, respectively (Fig. 5). Because of high HOMO energies of DDPPPA (−5.22 eV) and DDPBA (−5.20 eV) there is negligible hole injection barrier at TCTA:EML interface [DDPPPA (−0.48 eV) and DDPBA (−0.50 eV)]. Similarly, electron-injection barrier at TPBI:EML interface also small [DDPPPA (−0.31 eV) and DDPBA (−0.29 eV)], hence, small injection barrier promotes efficient hole and electron injection as well as transportation into the emission layer.70 Therefore, devices based on DDPPPA and DDPBA show maximum efficiency at low turn-on voltages of 2.3 and 2.2 V, respectively (Fig. 4). Electroluminescence (EL) spectra of DDPPPA (480 nm) and DDPBA (470 nm) show sky-blue emission with CIE (0.15, 0.08) and (0.15, 0.10), respectively (Fig. 1). Their EL spectra are almost same as that of PL spectra of the corresponding film. Absences of other peaks reveal that carrier recombination is well confined within the emissive layer.71
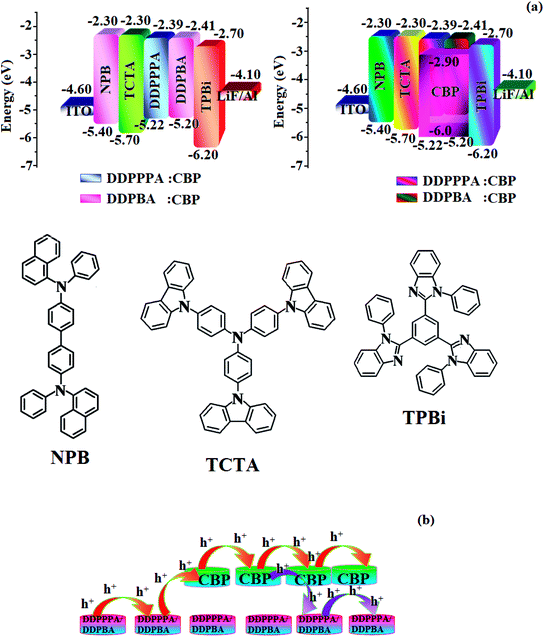 |
| Fig. 5 (a) Schematic representation of energy level diagram of DDPPPA and DDPBA; (b) carrier hopping through both CBP and DDPPPA and DDPBA. | |
The non-doped device with DDPPPA/DDPBA shows maximum ηc, ηp and ηex of 10.5/12 cd A−1, 8.3/9.2 lm W−1 and 5.7/6.0%, respectively (Fig. 4). The maximum exciton-utilizing efficiency (ηs) of the devices based on DDPPPA/DDPBA calculated as 31.4 and 35.3%, respectively, exceeds 25% theoretical limit of spin statistics for conventional fluorescent OLEDs. This can be attributed to the effective utilization of both energies of LE and CT exciton (i.e., hybridized local and charge-transfer). The high ηs is likely to be a result of an exciton channel via potential RISC from upper excited states (Fig. S4†).72 To the best of our knowledge, these efficiencies are highest EL efficiencies with CIEy <0.3 that have been reported73–83 (Table 3). We also observed that the luminance has a good linear relationship with current density shows that the enhanced ηs is not from triplet–triplet annihilation process. Transient PL decays (Fig. 1) reveal single-exponential lifetime of 1.38 ns (DDPPPA) and 1.13 ns (DDPBA). The single-lifetime decay profiles suggest that the LE and CT states are highly hybridized.84
Table 3 Summary of device efficiencies with reported non-doped emitters
Emitter |
Von (V) |
EL (nm) |
ηex (%) |
ηc (cd A−1) |
ηp (lm W−1) |
CIE (x, y) |
Ref. |
DDPPPA |
2.3 |
480 |
5.70 |
10.5 |
8.3 |
(0.15, 0.08) |
This work |
DDPBA |
2.2 |
470 |
6.0 |
12.0 |
9.2 |
(0.15, 0.30) |
This work |
Py-BPA-BPI |
2.15 |
471 |
5.64 |
10.9 |
10.5 |
(0.17, 0.29) |
18 |
TPA3HTPE |
3.7 |
471 |
3.8 |
6.97 |
7.99 |
— |
74 |
DBTO-MeTPE |
4.6 |
481 |
3.01 |
6.17 |
3.82 |
(0.17, 0.28) |
75 |
DPATPE |
3.2 |
488 |
3.3 |
7.5 |
7.9 |
— |
76 |
MeTPA-3pTP |
3.1 |
480 |
3.99 |
7.04 |
8.03 |
(0.17, 0.28) |
77 |
DPF-TPI |
2.7 |
468 |
4.85 |
8.41 |
7.23 |
(0.17, 0.24) |
15 |
TPATPE |
3.6 |
492 |
3.4 |
8.6 |
5.3 |
— |
79 |
TPTPE |
4.2 |
488 |
2.7 |
5.8 |
3.5 |
(0.17, 0.28) |
13 |
TPE-2mTPA |
3.6 |
489 |
— |
4.24 |
2.10 |
(0.14, 0.28) |
81 |
Blu2 |
3.0 |
— |
6.12 |
11.6 |
7.23 |
(0.148, 0.28) |
82 |
PBAPE |
6.3 |
466 |
— |
6.1 |
3.1 |
(0.19, 0.27) |
83 |
To further improve the efficiency and colour purity, we have used DDPPPA and DDPBA as emissive dopant and 4,4′,4′′-tris[3-methylphenyl(phenyl)amino]triphenylamine (m-MTDATA) as host to fabricate OLEDs. In this host-guest system, the emissive materials are diluted in the host matrix, not only device efficiency and colour purity is also improved via efficient Förster energy transfer. Electroluminescent devices based on doped light-emitting layers with ITO/NPB (40 nm)/m-MTDATA:DDPPPA or m-MTDATA:DDPBA (20 nm)/TPBi (25 nm)/LiF (1 nm)/Al (100 nm) have been fabricated. Herein, NPB (N,N′-di-1-naphthyl-N,N′-diphenylbenzidine) and TPBi functioned as electron- and hole-blocking materials, respectively. 4,4′,4′′-tris[3-Methylphenyl(phenyl)amino]triphenylamine (m-MTDATA) was employed as hole-transporting as well as electron-blocking layers and 1,3,5-tris(N-phenylbenzimidazol-2-yl)benzene (TPBi) was employed as electron-injection layers. These devices show white emission due to the mixed blue monomer emission and orange electroplex emission in the m-MTDATA:DDPPPA and or m-MTDATA:DDPBA (Fig. 4). The electroluminescence spectra of the doped devices based on DDPPPA/DDPBA (5% – 448, 536/488, 538; 10% – 448, 535/488, 537; 20% – 448, 537/448, 539) is shown in Fig. 2. The low-energy bands were additionally observed in the electroluminescence spectra of the doped OLEDs as compared to non-doped OLEDs EL spectra. Appearance of the additional band can be explained by the formation of dimeric excited states namely, exciplexes and or electroplexes either in doped light-emitting layer or at interface between layers of OLEDs due to cross-interaction between holes of donor and electrons of acceptor.85 To prove this assumption, PL spectra of the film of hosts and emitters molecular mixture were recorded (Fig. 2). The PL spectra of the mixture m-MTDATA:DDPPPA and m-MTDATA:DDPBA were similar to PL spectra of pure DDPPPA and DDPBA and did not contain bands at wavelength close to low energy band observed in EL spectra of doped OLEDs. The similarity of shapes and positions of the PL spectra of the mixtures to PL spectra of pure emitters (DDPPPA and DDPBA) indicates effective energy transfer from hosts to emitters. However, PL spectra of m-MTDATA:PBD is different as compared with PL spectra of pure m-MTDATA and PBD, respectively. The maximum at 540 nm of PL spectrum of m-MTDATA:PBD film was found to be at similar wavelength as the maximum of additional band in the EL spectra of devices with m-MTDATA as the host. The PL decay curve of m-MTDATA:PBD show double-exponential function (Fig. 2). The shorter and longer lived components attributed to monomeric fluorescence and exciplex emission, respectively. The exciplex emission is due to reverse intersystem crossing (RISC).85 Hence, low-energy emission band in EL spectra of doped OLEDs with m-MTDATA host can be ascribed to the interface exciplex between m-MTDATA and PBD.85 The CIE of m-MTDATA:DDPPPA and m-MTDATA:DDPBA and TAPC:DDPPPA and TAPC:DDPBA based device at voltages from 7 V to 8 V was found to be very close to white point (0.28, 0.26) (Fig. 4) due to the mixed blue monomer emission and orange electroplex emission in the m-MTDATA:DDPPPA and m-MTDATA:DDPBA and TAPC:DDPPPA and TAPC:DDPBA emitting-layer.
Diluting the emitter molecules in the host eliminates the effect of intermolecular close stacking and decreased medium polarity because m-MTDATA is generally used as a nonpolar host material.86,87 The DDPPPA and DDPBA based OLEDs show improved device efficiencies of ηex of 4.72% (5 wt% DDPPPA) and 5.63% (5 wt% DDPBA).88 The doped device m-MTDATA:DDPPPA/m-MTDATA:DDPBA show maximum efficiencies of ηc −7.4/8.23 cd A−1; ηp −5.8/6.13 lm W−1; ηex −4.72/5.63% (5 wt%):ηc −8.36/9.15 cd A−1; ηp −6.32/6.65 lm W−1; ηex −4.86/5.45% (10 wt%):ηc −9.58/10.02 cd A−1; ηp −7.8/8.25 lm W−1; ηex −5.96/6.25% (20 wt%). The doped device based on TAPC host TAPC:DDPPPA/TAPC:DDPBA exhibit maximum efficiencies of ηc −9.60/10.03 cd A−1; ηp −7.81/8.26 lm W−1; ηex −5.96/6.25% (20 wt%).
The theoretically calculated maximum ηEQE is of 5.61/5.20% DDPPPA (neat/20 wt% m-MDATA) and 5.55/5.10% DDPBA (neat/20 wt% m-MDATA) [ηEQE = ηout × ηrec × ηγ × ΦPL,89 ϕPL:DDPPPA (89/96%) and DDPBA (90/98%), ηout – out-coupling efficiency (20%), ηrec – product of charge recombination efficiency (100%), ηγ – radiative exciton-production (25%)] and the experimental ηEQE is of 5.70/5.96% DDPPPA (neat/20 wt%) and 6.01/6.25%, DDPBA (neat/20 wt%) respectively. Similar trend is observed by using TAPC as host (experimental ηEQE:DDPPPA/DDPBA:TAPC – 5.96/6.25 > theoretical ηEQE DDPPPA/DDPBA:TAPC – 5.61/5.56). The experimental ηEQE > theoretical ηEQE because larger triplet excitons are converted to singlet excitons in EL process.90–92 The ηr calculated for DDPPPA (neat/20 wt%) (25–31%/27–33%) and DDPBA (neat/20 wt%) (26–31%/27–34%) indicates γ < 100% because of poor unbalanced carrier transportation in the emissive layer. Enhanced ηIQE 28/29% (m-MTDATA or TAPC:DDPPPA:neat/20 wt%); 30/31% (m-MTDATA or TAPC:DDPBA:neat/20 wt%) and maximum ηs of 31.4/32.5% (m-MTDATA or TAPC:DDPPPA:neat/20 wt%) and 33.3/34.4% (m-MTDATA or TAPC:DDPBA:neat/20 wt%) [ηs = ηout × ηPL × ηres ÷ ηEL] is because of retained CT% in D–π–A compounds. Maximum ηs breaking 25% limit: 6.4% (DDPPPA) and 8.3% (DDPBA) of triplet exciton converted to singlet exciton by RISC and remaining follow non-radiative process leads to high efficiency blue OLEDs. These experimental results reveal that non-doped [DDPPPA and DDPBA] and doped [m-MTDATA:DDPPPA and m-MTDATA:DDPBA] devices are the efficient one. Additional triplet exciton is utilized in the OLEDs because of HLCT of DBTPIN and NDBNPIN as showing the accuracy for our molecular-design-strategy.
4. Conclusion
We have synthesized aromatically substituted phenanthroimidazole at C6 and C9 positions to enhance thermal, photochemical and electroluminescent properties. The intramolecular charge transfer between pyrene moiety at C6/C9 positions and phenanthroimidazole core has been confirmed by experimental and theoretical studies. Photophysical studies reveal that C6 and C9 pyrene substituent induced moderate conjugation relative to C2 modification. The blue device with DDPBA emissive layer show sky blue emission (470 nm, CIE-0.16, 0.10) with maximum efficiencies of ηc −12.0 cd A−1; ηp −9.2 lm W−1; ηex −6.0% at 2.2 V. Both devices show high singlet exciton utilizing efficiency (ηs) of DDPPPA-31.33% and DDPBA-35.29%. The doped device m-MTDATA:DDPPPA/m-MTDATA:DDPBA show maximum efficiencies of ηc −7.4/8.23 cd A−1; ηp −5.8/6.13 lm W−1; ηex −4.72/5.63% (5 wt%):ηc −8.36/9.15 cd A−1; ηp −6.32/6.65 lm W−1; ηex −4.86/5.45% (10 wt%):ηc −9.58/10.02 cd A−1; ηp −7.8/8.25 lm W−1; ηex −5.96/6.25% (20 wt%). The doped device based on TAPC host TAPC:DDPPPA/TAPC:DDPBA exhibit maximum efficiencies of ηc −9.60/10.03 cd A−1; ηp −7.81/8.26 lm W−1; ηex −5.96/6.25% (20 wt%).
Conflicts of interest
There are no conflicts of interest.
Acknowledgements
This research was supported by the DST (Department of Science and Technology – EMR/2014/000094, F. no. SR/S1/1C-73/2010, F. no. SR/S1/1C-07/2007), DRDO (Defence Research and Development Organization – 213/MAT/10-11), CSIR (Council of Scientific and Industrial Research – no. 01/(2707)/13EMR-II), UGC (University Grant Commission – 6-21/2008, F. no. 30-71/2004(SR)) and DST-Nano Mission (SR/NM/NS-1001/2016).
References
- C. H. Chen, M. T. Lee and C. H. Liao, SPIE Newsroom, 2006 Search PubMed.
- Z. Wang, P. Lu, S. Chen, Z. Gao, F. Shen and W. Zhang, J. Mater. Chem., 2011, 21, 5451–5457 RSC.
- Z. Jiang, Z. Liu, C. Yang, C. Zhong, J. Qin, G. Yu and Y. Liu, Adv. Funct. Mater., 2009, 19, 3987–3995 CrossRef.
- H. H. Chou, Y. H. Chen, H. P. Hsu, W. H. Chang, Y. H. Chen and C. H. Cheng, Adv. Mater., 2012, 24, 5867–5871 CrossRef PubMed.
- H. Sasabe and J. Kido, J. Mater. Chem. C, 2013, 1, 1699–1707 RSC.
- X. Ouyang, X. L. Li, L. Ai, D. Mi, Z. Ge and S. J. Su, ACS Appl. Mater. Interfaces, 2015, 7, 7869–7877 CrossRef CAS PubMed.
-
(a) J. Ding, J. Gao, Y. Cheng, Z. Xie, L. Wang, D. Ma, X. Jing and F. Wang, Adv. Funct. Mater., 2006, 16, 575–581 CrossRef CAS;
(b) H. Sasabe, J. Takamatsu, T. Motoyama, S. Watanabe, G. Wagenblast, N. Langer, O. Molt, E. Fuchs, C. Lennartz and J. Kido, Adv. Mater., 2010, 22, 5003–5007 CrossRef CAS PubMed;
(c) M. Zhu and C. Yang, Chem. Soc. Rev., 2013, 42, 4963–4976 RSC;
(d) T. Fleetham, G. J. Li, L. L. Wen and J. Li, Adv. Mater., 2014, 26, 7116–7121 CrossRef PubMed.
-
(a) J. Huang, N. Sun, Y. Dong, R. Tang, P. Lu, P. Cai, Q. Li, D. Ma, J. Qin and Z. Li, Adv. Funct. Mater., 2013, 23, 2329–2337 CrossRef;
(b) C. J. Tonzola, A. P. Kulkarni, A. P. Gifford, W. Kaminsky and S. A. Jenekhe, Adv. Funct. Mater., 2007, 17, 863–874 CrossRef;
(c) C. H. Chien, C. K. Chen, F. M. Hsu, C. F. Shu, P. T. Chou and C. H. Lai, Adv. Funct. Mater., 2009, 19, 560–566 CrossRef.
- M. Zhu and C. Yang, Chem. Soc. Rev., 2013, 42, 4963–4976 RSC.
- A. Richaud, N. Barba-Behrens and F. Méndez, Org. Lett., 2011, 13, 972–975 CrossRef.
- K. Hofmann, Imidazole and its derivatives, Inter Science Publishers, Part I Inc., New York, 1953, ISBN: 0-470-37653-8 Search PubMed.
- W. C. Chen, Y. Yuan, G. F. Wu, H. X. Wei, L. Tang, Q. X. Tong, F. L. Wong and C. S. Lee, Adv. Opt. Mater., 2014, 2, 626–631 CrossRef.
- W. Li, D. Liu, F. Shen, D. Ma, Z. Wang, T. Feng, Y. Xu, B. Yang and Y. Ma, Adv. Funct. Mater., 2012, 22, 2797–2803 CrossRef.
- W. C. Chen, Y. Yuan, S. F. Ni, Z. L. Zhu, J. Zhang, Z. Q. Jiang, L. S. Liao, F. L. Wong and C. S. Lee, ACS Appl. Mater. Interfaces, 2017, 9, 7331–7338 CrossRef.
- Y. Yuan, J. X. Chen, F. Lu, Q. X. Tong, Q. D. Yang, H. W. Mo, T. W. Ng, F. L. Wong, Z. Q. Guo, J. Ye, Z. Chen, X. H. Zhang and C. S. Lee, Chem. Mater., 2013, 25, 4957–4965 CrossRef.
- Y. Zhang, J. H. Wang, G. Han, F. Lu and Q. X. Tong, RSC Adv., 2016, 6, 70800–70809 RSC.
- M. Chen, Y. Yuan, J. Zheng, W. C. Chen, L. J. Shi, Z. Zhu, L. F. Lu, Q. X. Tong, Q. D. Yang, J. Ye, M. Y. Chan and C. S. Lee, Adv. Opt. Mater., 2015, 3, 1215–1219 CrossRef.
- B. Liu, Y. Yuan, D. He, D. Y. Huang, C. Y. Luo, Z. L. Zhu, F. Lu, Q. X. Tong and C. S. Lee, Chem.–Eur. J., 2016, 22, 12130–12137 CrossRef.
- D. He, Y. Yuan, B. Liu, D. Y. Huang, C. Y. Luo, F. Lu, Q. X. Tong and C. S. Lee, Dyes Pigm., 2017, 136, 347–353 CrossRef.
-
(a) S. Jeong and J. I. Hong, Dyes Pigm., 2017, 144, 9–16 CrossRef;
(b) A. Islam, Q. Wang, L. Zhang, T. Lei, L. Hong, R. Yang, Z. Liu, R. Peng, L. S. Liao and Z. Ge, Dyes Pigm., 2017, 142, 499–506 CrossRef.
- Y. Park, J. H. Lee, D. H. Jung, S. H. Liu, Y. H. Lin and L. Y. Chen, J. Mater. Chem., 2010, 20, 5930–5936 RSC.
- Z. X. Wang, H. X. Shao, J. C. Ye, L. Zhang and P. Lu, Adv. Funct. Mater., 2007, 17, 253–263 CrossRef.
- S. Tao, Y. Zhou, C. S. Lee, S. T. Lee, D. Huang and X. Zhang, J. Phys. Chem. C, 2008, 112, 14603–14606 CrossRef.
- S. K. Kim, B. Yang, Y. I. Park, Y. Ma, J. Y. Lee, H. J. Kim and J. Park, Org. Electron., 2009, 10, 822–833 CrossRef.
- W. Li, D. Liu, F. Shen, D. Ma, Z. Wang, T. Feng, Y. Xu, B. Yang and Y. Ma, Adv. Funct. Mater., 2012, 22, 2797–2803 CrossRef CAS.
- L. Yao, S. Zhang, R. Wang, W. Li, F. Shen, B. Yang and Y. Ma, Angew. Chem., Int. Ed., 2014, 53, 2119–2123 CrossRef CAS.
- S. K. Kim, B. Yang, Y. Ma, J. H. Lee and J.-W. Park, J. Mater. Chem., 2008, 18, 3376–3384 RSC.
- H. Liu, Q. Bai, L. Yao, H. Zhang, H. Xu, S. Zhang, W. Li, Y. Gao, J. Li, P. Lu, H. Wang, B. Yang and Y. Mac, Chem. Sci., 2015, 6, 3797–3804 RSC.
- H. Wang, L. Xie, Q. Peng, L. Meng, Y. Wang, Y. Yi and P. Wang, Adv. Mater., 2014, 26, 5198–5204 CrossRef CAS.
- Z. Wu, J. Luo, N. Sun, L. Zhu, H. Sun, L. Yu, D. Yang, X. Qiao, J. Chen, C. Yang and D. Ma, Adv. Funct. Mater., 2016, 26, 3306–3313 CrossRef CAS.
- W. Li, Y. Pan, L. Yao, H. Liu, S. Zhang, C. Wang, F. Shen, P. Lu, B. Yang and Y. Ma, Adv. Opt. Mater., 2014, 2, 892–901 CrossRef CAS.
- Q. Zhang, B. Li, S. Huang, H. Nomura, H. Tanaka and C. Adachi, Nat. Photonics, 2014, 8, 326–332 CrossRef CAS.
- S. Y. Lee, T. Yasuda, H. Nomura and C. Adachi, Appl. Phys. Lett., 2012, 101, 093306 CrossRef.
- H. Tanaka, K. Shizu, H. Miyazaki and C. Adachi, Chem. Commun., 2012, 48, 11392–11394 RSC.
- T. Nakagawa, S.-Y. Ku, K.-T. Wong and C. Adachi, Chem. Commun., 2012, 48, 9580–9582 RSC.
- W. J. Li, Y. Y. Pan, R. Xiao, Q. M. Peng, S. T. Zhang, D. G. Ma, F. Li, F. Z. Shen, Y. H. Wang, B. Yang and Y. G. Ma, Adv. Funct. Mater., 2014, 24, 1609–1614 CrossRef.
- S. Tang, W. J. Li, F. Z. Shen, D. D. Liu, B. Yang and Y. G. Ma, J. Mater. Chem., 2012, 22, 4401–4408 RSC.
- S. T. Zhang, W. J. Li, L. Yao, Y. Y. Pan, B. Yang and Y. G. Ma, Chem. Commun., 2013, 49, 11302–11304 RSC.
- L. Yao, S. T. Zhang, R. Wang, W. J. Li, F. Z. Shen, B. Yang and Y. G. Ma, Angew. Chem., Int. Ed., 2014, 53, 2119–2123 CrossRef CAS.
- Y. Y. Pan, W. J. Li, S. T. Zhang, L. Yao, C. Gu, H. Xu, B. Yang and Y. G. Ma, Adv. Opt. Mater., 2014, 2, 510–515 CrossRef CAS.
- W. J. Li, Y. Y. Pan, L. Yao, H. C. Liu, S. T. Zhang, C. Wang, F. Z. Shen, B. Yang and G. A. Ma, Adv. Opt. Mater., 2014, 2, 892–901 CrossRef CAS.
- D. Chaudhuri, E. Sigmund, A. Meyer, L. Rcck, P. Klemm, S. Lautenschlager, A. Schmid, S. R. Yost, T. Van, S. Bange, S. Hcger and J. M. Lupton, Angew. Chem., 2013, 52, 13449–13452 CrossRef CAS.
- J. R. Sheats, H. Antoniadis, M. Hueschen, W. Leonard, J. Miller, R. Moon and D. Roitman, Science, 1996, 273, 884–888 CrossRef CAS.
- T. Forster, Discuss. Faraday Soc., 1959, 27, 7–17 RSC.
- S. Zhang, L. Yao, Q. Peng, W. Li, Y. Pan, R. Xiao, Y. Gao, C. Gu, Z. Wang, P. Lu, F. Li, S. Su, B. Yang and Y. Ma, Adv. Funct. Mater., 2015, 25, 1755–1762 CrossRef CAS.
-
(a) M. J. Frisch, G. W. Trucks, H. B. Schlegel, G. E. Scuseria, M. A. Robb, J. R. Cheeseman, G. Scalmani, V. Barone, B. Mennucci, G. A. Petersson, H. Nakatsuji, M. Caricato, X. Li, H. P. Hratchian, A. F. Izmaylov, J. Bloino, G. Zheng, J. L. Sonnenberg, M. Hada, M. Ehara, K. Toyota, R. Fukuda, J. Hasegawa, M. Ishida, T. Nakajima, Y. Honda, O. Kitao, H. Nakai, T. Vreven, J. A. Montgomery, J. E. Peralta, F. Ogliaro, M. Bearpark, J. J. Heyd, E. Brothers, K. N. Kudin, V. N. Staroverov, R. Kobayashi, J. Normand, K. Raghavachari, A. Rendell, J. C. Burant, S. S. Iyengar, J. Tomasi, M. Cossi, N. Rega, J. M. Millam, M. Klene, J. E. Knox, J. B. Cross, V. Bakken, C. Adamo, J. Jaramillo, R. Gomperts, R. E. Stratmann, O. Yazyev, A. J. Austin, R. Cammi, C. Pomelli, J. W. Ochterski, R. L. Martin, K. Morokuma, V. G. Zakrzewski, G. A. Voth, P. Salvador, J. J. Dannenberg, S. Dapprich, A. D. Daniels, O. Farkas, J. B. Foresman, J. V. Ortiz, J. Cioslowski and D. J. Fox, Revision A.02, Gaussian, Inc., Wallingford, CT, 2009 Search PubMed;
(b) T. Lu and F. Chen, J. Comput. Chem., 2012, 33, 580–592 CrossRef CAS.
- S. Zhang, L. Yao, Q. Peng, W. Li, Y. Pan, R. Xiao, Y. Gao, C. Gu, Z. Wang, P. Lu, F. Li, S. Su, B. Yang and Y. Ma, Adv. Funct. Mater., 2015, 25, 1755–1762 CrossRef CAS.
- M. A. Baldo, S. Lamansky, P. E. Burrows, M. E. Thompson and S. R. Forrest, Appl. Phys. Lett., 1999, 60, 14422–14428 CAS.
- Y. Tao, Q. Wang, C. Yang, Q. Wang, Z. Zhang and T. Zou, Angew. Chem., 2008, 47, 8104–8107 CrossRef CAS.
- W. C. Chen, Y. Yuan, G. F. Wu, H. X. Wei, J. Ye, M. Chen, F. Lu, Q. X. Tong, F. L. Wong and C. S. Lee, Org. Electron., 2015, 17, 159–166 CrossRef CAS.
- R. L. Martin, J. Chem. Phys., 2003, 118, 4775–4777 CrossRef CAS.
- S. Tretiak and S. Mukamel, Chem. Rev., 2002, 102, 3171–3212 CrossRef CAS.
-
(a) C. J. Kuo, T. Y. Li, C. C. Lien, C. H. Liu, F. I. Wu and M. J. Huang, J. Mater. Chem., 2009, 19, 1865–1871 RSC;
(b) Z. M. Wang, P. Lu, S. M. Chen, Z. Gao, F. Z. Shen, W. S. Zhang, Y. X. Xu, H. S. Kwok and Y. G. Ma, J. Mater. Chem., 2011, 21, 5451–5456 RSC;
(c) W. J. Li, D. D. Liu, F. Z. Shen, D. G. Ma, Z. M. Wang, T. Feng, Y. X. Xu, B. Yang and Y. G. Ma, Adv. Funct. Mater., 2012, 22, 2797–2803 CrossRef CAS;
(d) K. Wang, F. C. Zhao, C. G. Wang, S. Y. Chen, D. Chen, H. Y. Zhang, Y. Liu, D. G. Ma and Y. Wang, Adv. Funct. Mater., 2013, 23, 2672–2680 CrossRef CAS;
(e) W. C. Chen, Y. Yuan, G. F. Wu, H. X. Wei, L. Tang, Q. X. Tong, F. L. Wong and C. S. Lee, Adv. Opt. Mater., 2014, 2, 626–631 CrossRef CAS;
(f) W. C. Chen, Q. X. Tong and C. S. Lee, J. Mater. Chem. C, 2015, 3, 10957–10963 RSC;
(g) C. Li, S. Wang, W. Chen, J. Wei, G. Yang, K. Ye, Y. Liu and Y. Wang, Chem. Commun., 2015, 51, 10632–10635 RSC;
(h) W. C. Chen, Y. Yuan, G. F. Wu, H. X. Wei, J. Ye, M. Chen, Q. X. Lu, F. Tong, F. L. Wong and C. S. Lee, Org. Electron., 2015, 17, 159–166 CrossRef;
(i) L. Bin, J. W. Zhao, C. Y. Luo, L. Feng, S. L. Tao and Q. X. Tong, J. Mater. Chem. C, 2016, 4, 2003–2010 Search PubMed.
-
(a) J. Ye, Z. Chen, M. K. Fung, C. Zheng, X. Ou, X. Zhang, Y. Yuan and C. S. Lee, Chem. Mater., 2013, 25, 2630–2637 CrossRef;
(b) Y. C. Li, Z. H. Wang, X. L. Li, G. Z. Xie, D. C. Chen, Y. F. Wang, C. C. Lo, A. Lien, J. B. Peng, Y. Cao and S. J. Su, Chem. Mater., 2015, 27, 1100–1109 CrossRef;
(c) R. Hu, E. Lager, A. A. Aguilar, J. Z. Liu, J. W. Y. Lam, H. H. Y. Sung, I. D. Williams, Y. C. Zhong, K. S. Wong, E. Pena-Cabrera and B. Z. Tang, J. Phys. Chem. A, 2009, 113, 15845–15853 CAS.
-
(a) Y. Zhang, S. L. Lai, Q. X. Tong, M. F. Lo, T. W. Ng, M. Y. Chan, Z. C. Wen, J. He, K. S. Jeff, X. L. Tang, W. M. Liu, C. C. Ko, P. F. Wang and C. S. Lee, Chem. Mater., 2012, 24, 61–70 CrossRef;
(b) C. Chen, Y. Yuan, Y. Xiong, A. L. Rogach, Q. X. Tong and C.-S. Lee, ACS Appl. Mater. Interfaces, 2017, 9, 26268–26278 CrossRef PubMed.
- S. Zhuang, R. Shangguan, H. Huang, G. Tua, L. Wang and X. Zhu, Dyes Pigm., 2014, 101, 93–102 CrossRef CAS.
- Z. Ma, E. Wang, M. E. Jarvid, P. Henriksson, O. Inganas, F. Zhang and M. R. Andersson, J. Mater. Chem., 2012, 22, 2306–2314 RSC.
-
(a) W. J. Li, D. D. Liu, F. Z. Shen, D. G. Ma, Z. M. Wang, T. Feng, Y. X. Xu, B. Yang and Y. G. Ma, Adv. Funct. Mater., 2012, 22, 2797–2803 CrossRef;
(b) G. B. Bodedla, K. R. Justin Thomas, M.-S. Fan and K.-C. Ho, J. Org. Chem., 2016, 81, 640–653 CrossRef.
- X. Chen, G. Zhang, H. Luo, Y. Li, Z. Liu and D. Zhang, J. Mater. Chem. C, 2014, 2, 2869–2876 RSC.
-
(a) G. M. Li, D. X. Zhu, T. Peng, Y. Liu, Y. Wang and M. R. Bryce, Adv. Funct. Mater., 2014, 24, 7420–7426 CrossRef;
(b) Y. T. Tao, Q. Wang, C. L. Yang, C. Zhong, K. Zhang, J. G. Qin and D. G. Ma, Adv. Funct. Mater., 2010, 20, 304–311 CrossRef;
(c) H. Liu, P. Chen, D. H. Hu, X. Y. Tang, Y. Y. Pan, H. H. Zhang, W. Q. Zhang, X. Han, Q. Bai, P. Lu and Y. G. Ma, Chem.–Eur. J., 2014, 86, 7286–7292 Search PubMed;
(d) D. H. Hu, F. Z. Shen, H. Liu, P. Lu, Y. Lv, D. D. Liu and Y. G. Ma, Chem. Commun., 2012, 48, 3015–3017 RSC.
- M. R. Zhu and C. L. Yang, Chem. Soc. Rev., 2013, 42, 4963–4976 RSC.
- H. H. Chou, Y. H. Chen, H. P. Hsu, W. H. Chang, Y. H. Chen and C. H. Cheng, Adv. Mater., 2012, 24, 5867–5871 CrossRef.
- Q. Zhang, J. Li, K. Shizu, S. Huang, S. Hirata, H. Miyazaki and C. Adachi, J. Am. Chem. Soc., 2012, 134, 14706–14709 CrossRef.
-
(a) M. Segal, M. Singh, K. Rivoir, S. Difley, T. V. Voorhis and M. A. Baldo, Nat. Mater., 2007, 6, 374–378 CrossRef;
(b) W. Barford, Phys. Rev. B: Condens. Matter Mater. Phys., 2004, 70, 205204–205212 CrossRef.
- W. Jiang, L. Duan, J. Qiao, G. Dong, L. Wang and Y. Qiu, Org. Lett., 2011, 13, 3146–3149 CrossRef.
- Y. Tao, Q. Wang, C. Yang, C. Zhong, K. Zhang, J. Ma and D. Qin, Adv. Funct. Mater., 2010, 20, 304–311 CrossRef.
- Y. Liu, L. S. Cui, M. F. Xu, X. B. Shi, X. B. Zhou, Z. K. Wang, Z. Q. Jiang and L. S. Liao, J. Mater. Chem. C, 2014, 2, 2488–2495 RSC.
- Y. H. Lou, M. F. Xu, L. Zhang, Z. K. Wang, S. Naka, H. Okada and L. S. Liao, Org. Electron., 2013, 14, 2698–2704 CrossRef.
- Z. Wang, Y. Lou, S. Naka and H. Okada, Appl. Phys. Lett., 2011, 98, 063302–063306 CrossRef.
- R. R. Hu, E. Lager, A. L. Aguilar-Aguilar, J. Z. Liu, J. W. Y. Lam, H. H. Y. Sung, I. D. Williams, Y. C. Zhong, K. S. Wong, E. Pena-Cabrera and B. Z. Tang, J. Phys. Chem. A, 2009, 113, 15845–15853 Search PubMed.
-
(a) Y. P. Li, F. Li, H. Y. Zhang, Z. Q. Xie, W. J. Xie, H. Xu, B. Li, F. Z. Shen, L. Ye, M. Hanif, D. G. Ma and Y. G. Ma, Chem. Commun., 2007, 231–233 RSC;
(b) Y. P. Li, F. Z. Shen, H. Wang, F. He, Z. Q. Xie, H. Y. Zhang, Z. M. Wang, L. L. Liu, F. Li, M. Hanif, L. Ye and L. Ma, Chem. Mater., 2008, 20, 7312–7318 CrossRef.
-
(a) S. T. Zhang, W. J. Li, L. Yao, Y. Y. Pan, F. Z. Shen, R. Xiao, B. Yang and Y. G. Ma, Chem. Commun., 2013, 49, 11302–11304 RSC;
(b) C. Cao, W. C. Chen, J. X. Chen, L. Yang, X. Z. Wang, H. Yang, B. Huang, Z. L. Zhu, Q. X. Tong and C. S. Lee, ACS Appl. Mater. Interfaces, 2019, 11, 11691–11698 CrossRef;
(c) H. C. Liu, Q. Bai, L. Yao, H. Y. Zhang, H. Xu, S. T. Zhang, W. J. Li, Y. Gao, J. Y. Li, P. Lu, H. Y. Wang, B. Yang and Y. G. Ma, Chem. Sci., 2015, 6, 4623–4635 RSC;
(d) X. Y. Tang, Q. Bai, Q. M. Peng, Y. Gao, J. Y. Li, Y. L. Liu, L. Yao, P. Lu, B. Yang and Y. G. Ma, Chem. Mater., 2015, 27, 7050–7057 CrossRef CAS;
(e) Q. Zhang, D. Tsang, H. Kuwabara, Y. Hatae, B. Li, T. Takahashi, S. Y. Lee, T. Yasuda and C. Adachi, Adv. Mater., 2015, 27, 2096–2100 CrossRef CAS.
- B. Liu, Y. Yuan, D. He, D. Y. Huang, C. Y. Luo, Z. L. Zhu, F. Lu, Q. X. Tong and C. S. Lee, Chem.–Eur. J., 2016, 22, 12130–12137 CrossRef CAS.
- G. Chen, W. Li, T. Zhou, Q. Peng, D. Zhai, H. Li, W. Z. Yuan, Y. Zhang and B. Z. Tang, Adv. Mater., 2015, 27, 4496–4501 CrossRef CAS.
- L. Zhan, S. Wang, L. X. Ding, Z. Li and H. Wang, J. Mater. Chem. C, 2015, 3, 19711–19717 RSC.
- C. Y. K. Chan, J. W. Y. Lam, Z. Zhao, S. Chen, P. Lu, H. H. Y. Sung, H. S. Kwok, Y. Ma, I. D. Williams and B. Z. Tang, J. Mater. Chem. C, 2014, 2, 4320–4327 RSC.
- J. Huang, N. Sun, J. Yang, R. Tang, Q. Li, D. Ma and Z. Li, Adv. Funct. Mater., 2014, 24, 7645–7654 CrossRef CAS.
-
(a) C. Cao, W. C. Chen, S. Tian, J. X. Chen, Z. Y. Wang, X. H. Zheng, C. W. Ding, J. H. Li, J. J. Zhu, Z. L. Zhu, Q. X. Tong and C. S. Lee, Mater. Chem. Front., 2019, 3, 1071–1079 RSC;
(b) X. H. Zheng, J. W. Zhao, T. T. Huang, X. Chen, C. Cao, G. X. Yang, Z. H. Lin, Q. X. Tong, S. L. Tao and D. Liu, ChemElectroChem, 2019, 6, 5810–5818 CrossRef CAS.
- Y. Liu, S. Chen, J. W. Y. Lam, P. Lu, R. T. K. Kwok, F. Mahtab, H. S. Kwok and B. Z. Tang, Chem. Mater., 2011, 23, 2536–2544 CrossRef CAS.
- C. Y. K. Chan, Z. Zhao, J. W. Y. Lam, J. Liu, S. Chen, P. Lu, F. Mahtab, X. Chen, H. H. Y. Sung, H. S. Kwok, Y. Ma, I. D. Williams, K. S. Wong and B. Z. Tang, Adv. Funct. Mater., 2012, 22, 378–389 CrossRef CAS.
- J. Huang, R. Tang, T. Zhang, Q. Li, G. Yu, S. Xie, Y. Liu, S. Ye, J. Qin and Z. Li, Chem.–Eur. J., 2014, 20, 5317–5326 CrossRef CAS.
- C. G. Zhen, Y. F. Dai, W. J. Zeng, Z. Z. K. Chen and J. Kieffer, Adv. Funct. Mater., 2011, 21, 699–707 CrossRef CAS.
- S. K. Kim, Y. Park, I. Kang and J. Park, J. Mater. Chem., 2007, 17, 4670–4678 RSC.
- H. Uoyama, K. Goushi, K. Shizu, H. Nomura and C. Adachi, Nature, 2012, 492, 234–238 CrossRef CAS.
-
(a) R. Butkute, R. Lygaitis, V. Mimaite, D. Gudeika, D. Volyniuk, G. Sini and J. V. Grazulevicius, Dyes Pigm., 2017, 146, 425–437 CrossRef CAS;
(b) T. Zhang, B. Chu, W. Li, Z. Su, Q. M. Peng, B. Zhao, Y. Luo, F. Jin, X. Yan, Y. Gao, H. Wu, F. Zhang, D. Fan and J. Wang, ACS Appl. Mater. Interfaces, 2014, 6, 11907–11921 CrossRef CAS PubMed;
(c) D. Chen, Z. Wang, D. Wang, Y. C. Wu, C. Lo, A. Lien, Y. Cao and S. J. Su, Org. Electron., 2015, 25, 79–84 CrossRef CAS.
- G. Xie, D. Chen, X. Li, X. Cai, Y. Li, D. Chen, K. Liu, Q. Zhang, Y. Cao and S. J. Su, ACS Appl. Mater. Interfaces, 2016, 8, 27920–27930 CrossRef CAS.
- J. H. Jou, Y. L. Chen, J. R. Tseng, R. Z. Wu, J. J. Shyue, K. R. Justin Thomas, N. Kapoor, C. T. Chen, Y. P. Lin, P. H. Wang, H. W. Hung, J. Y. Li and S. P. Chen, J. Mater. Chem., 2012, 22, 15500–15506 RSC.
- W. C. Chen, Y. Yuan, G. F. Wu, H. X. Wei, J. Ye, M. Chen, F. Lu, Q. X. Tong, F. L. Wong and C. S. Lee, Org. Electron., 2015, 17, 159–166 CrossRef CAS.
- S. H. Cho, J. R. Oh, H. K. Park, H. K. Kim, Y. H. Lee, J. G. Lee and Y. R. Do, Opt. Express, 2010, 18, 1099–1104 CrossRef CAS.
- M. Zhu and C. Yang, Chem. Soc. Rev., 2013, 42, 4963–4976 RSC.
- W. C. Chen, Q. X. Tong and C. S. Lee, Sci. Adv. Mater., 2015, 7, 2193–2205 CrossRef CAS.
- Y. Xu, X. Liang, X. Zhou, P. Yuan, J. Zhou, C. Wang, B. Li, D. Hu, X. Qiao, X. Jiang, L. Liu, S. J. Su, D. Ma and Y. Ma, Adv. Mater., 2019, 31, 1807388–1807396 CrossRef PubMed.
Footnote |
† Electronic supplementary information (ESI) available. See DOI: 10.1039/d0ra00658k |
|
This journal is © The Royal Society of Chemistry 2020 |