DOI:
10.1039/D0RA00459F
(Paper)
RSC Adv., 2020,
10, 11219-11224
The influence of an ultra-high resistivity Ta underlayer on perpendicular magnetic anisotropy in Ta/Pt/Co/Pt heterostructures
Received
16th January 2020
, Accepted 3rd March 2020
First published on 17th March 2020
Abstract
Thin films with perpendicular magnetic anisotropy (PMA) play an essential role in the development of technologies due to their excellent thermal stability and potential application in devices with high density, high stability, and low energy consumption. Many studies have focused on the relationship between the resistivity of heavy metals and the PMA of the neighbouring magnetic metals in magnetic multi-layered films. However, reports on the effects of heavy metals non-adjacent to the magnetic metals on the PMA are rare. Herein, we demonstrate the influence of the heavy metal Ta underlayer non-adjacent to the magnetic Co layer on the PMA and thermal stability in the Ta/Pt/Co/Pt heterostructures. A type of amorphous Ta film having an ultra-high resistivity (ρmax = 3.9 × 105 μΩ cm) was optimized by DC sputtering at a high sputtering Ar pressure, low sputtering power, and large target-to-substrate distance. The value of resistivity is three orders of magnitude higher than that of the β-Ta underlayer. We found that this special Ta underlayer can effectively improve the PMA and thermal stability of the magnetic Co layer based on the anomalous Hall and planar Hall effect measurements. The maximum magnetic anisotropic field reaches 1.1 T at a low temperature. It is very likely that the ultra-high resistivity leads to the increase in the additional electron scattering in the Ta/Pt interface, while the latter results in the enhancement of the PMA and thermal stability in the structure. These results reveal the inherent relationship between the resistivity of the heavy metal underlayer and PMA, and provide a novel approach to improve the PMA and thermal stability of heavy metal/magnetic metal multi-layered films.
Introduction
Thin films with perpendicular magnetic anisotropy (PMA) play an essential role in the development of technologies in the field of modern magnetics. Due to their excellent thermal stability and potential applications in ultra-high-density devices, they have garnered strong research interests.1–5 PMA is a notable behavior that causes the magnetic moment of a magnetic material to be aligned perpendicular to the film plane, whereas the shape of the thin film usually makes the magnetic moment parallel to the film plane. Currently, PMA materials are widely applied in memory and logic devices.6–9 Since the magnetic moment being perpendicular to the film plane is beneficial to the formation of smaller storage cells, the magnetic storage density can be increased. The PMA films can effectively eliminate the restrictions on the area and shape imposed by the vortex rotation in the magnetic layer. Moreover, the competitions between PMA and other magnetic mechanisms (such as Dzyaloshinskii–Moriya interaction) can produce rich and novel chiral spin textures, such as magnetic skyrmions.10 Therefore, the realization and modulation of PMA in ferromagnetic materials is of great significance.
Recently, current-induced magnetization switching has been realized in PMA heavy metal/magnetic metal multi-layered films, which has significantly reduced the critical switching current density and the power consumption of devices.11–18 In general, heavy metal layers with high resistivity, which are directly adjacent to the magnetic metal layer, are utilized to improve the PMA, spin–orbit torque (SOT) and critical switching current density of multi-layers. For example, in tungsten/magnetic metal films, the high resistivity of β-W can significantly increase the effective magnetic anisotropic field (Han), thus leading to a decrease in the critical switching current density and a significant increase in the spin Hall angle.19–24 The same results were also reported for high resistivity β-Ta based multilayered film structures.25–28 Moreover, the insertion of a non-magnetic layer between the heavy metal/magnetic metal layers will also affect the spin–orbit coupling (SOC) of the film interface, and an appropriate insertion layer can effectively improve the PMA and reduce the spin Hall current density.29–32 However, there are few studies on the influence of the inserted heavy metal layer non-adjacent to the magnetic metals on PMA and SOT in the heavy metal/magnetic metal multi-layered films. In this study, the influence of the Ta underlayer inserted between the substrate and heavy metal/magnetic metal multi-layers on the PMA and thermal stability was investigated. A type of ultra-high resistivity Ta film was obtained via the optimization of the experimental conditions, and its maximum resistivity reaches 3.9 × 105 μΩ cm, which is three orders of magnitude higher than that of β-Ta. The anomalous and planar Hall effect measurements show that the Ta underlayer having ultra-high resistivity effectively improved the Han and thermal stability of Ta/Pt/Co/Pt multi-layered films. The highest Han is 1.1 T at a low temperature, which is far larger than that of the β-Ta underlayer samples. This study provides a novel approach to improve the PMA and thermal stability of the heavy metal/magnetic metal multi-layered films.
Experimental method
Pt (5 nm)/Co (0.9 nm)/Pt (2 nm) (labeled as Pt/Co/Pt) and Ta (3 nm)/Pt (5 nm)/Co (0.9 nm)/Pt (2 nm) (labeled as Ta/Pt/Co/Pt) multi-layered films were prepared by DC sputtering on thermally oxidized Si substrates at room temperature. The base pressure of the sputtering system was 5 × 10−9 torr and the sputtering target-to-substrate distance was 25 cm. The Pt layers were grown at Ar pressure of 4 mTorr and sputtering power of 15 W; the Co layers were grown at Ar pressure of 20 mTorr and sputtering power of 80 W. A series of Ta films with 20 nm thicknesses were prepared by changing the sputtering Ar pressure (4–20 mTorr) and sputtering power (8–20 W). A standard four-point probe was used to test the resistivity of Ta films. It was found that the Ta films fabricated at a high power and low sputtering Ar pressure have relatively small resistivity in the range of (2.3–3.6) × 102 μΩ cm, corresponding to the β-Ta phase.28,29 Moreover, the Ta films prepared at a low power and high sputtering Ar pressure have ultra-high resistivity, and the maximum value reaches 3.9 × 105 μΩ cm. In order to clear the influence of resistivity on the PMA, two types of Pt/Co/Pt multi-layered films with a 3 nm Ta underlayer were investigated. The β-Ta and ultra-high resistivity Ta layers were prepared under the conditions: sputtering Ar pressure of 4 mTorr and sputtering power of 20 W; sputtering Ar pressure of 20 mTorr and sputtering power of 8 W, respectively. The two types of Ta/Pt/Co/Pt multi-layered films were labeled as Ta/Pt/Co/Pt-4 and Ta/Pt/Co/Pt-20 according to Ta layer's sputtering Ar pressure. A superconducting quantum interference device (SQUID) magnetometer was used to measure the in-plane and out-of-plane magnetic properties of the films. X-ray diffraction (XRD) was used to characterize the structure of the multi-layered films, and atomic force microscopy (AFM) was used to characterize the surface morphologies of the Ta films. Using standard photolithography and Ar ion etching, the samples were patterned into Hall bars with dimensions of 10 × 100 μm2 for Hall voltage measurements. A physical property measurement system (PPMS) was used for the transport measurements at temperatures ranging from 10 K to 300 K. The Ar in the Ta film was investigated via X-ray photoelectron spectroscopy (XPS) using an Al Kα X-ray source. The Ta/Pt/Co/Pt-20 sample was annealed in a vacuum furnace at two different temperatures of 260 °C and 380 °C for 1 hour.22
Results and discussion
Fig. 1 shows the in-plane and out-of-plane magnetic hysteresis loops of the Pt/Co/Pt, Ta/Pt/Co/Pt-4, and Ta/Pt/Co/Pt-20 samples measured at room temperature. As can be seen that all the three samples exhibit PMA, which is related to the interfacial symmetry break and strong interface SOC of the heavy metals.33,34 The insertion of the Ta underlayer not only relaxes the lattice mismatch between the substrate and the heavy metal Pt but also effectively improves the squareness ratio and coercivity of the out-of-plane hysteresis loops, as shown in the insets of Fig. 1. Fig. 1(c) shows that the squareness ratio of the Ta/Pt/Co/Pt-20 sample was close to 1, and the coercivity reaches 440 Oe. These values are obviously larger than that of the Pt/Co/Pt and Ta/Pt/Co/Pt-4 samples [see the insets of Fig. 1(a) and (b)]. The value of Han can be roughly estimated using the difference between the out-of-plane and in-plane saturation fields.35,36 In the case of Ta/Pt/Co/Pt-20, the value of Han = ∼2700 Oe is clearly higher than that of the Pt/Co/Pt (Han = ∼1100 Oe) and Ta/Pt/Co/Pt-4 (Han = ∼1500 Oe) samples (the quantitative analysis of Han will be carried out in the next content). Thus, the PMA and thermal stability of the Pt/Co/Pt can be enhanced by inserting the ultra-high resistivity Ta underlayer. Here, two points should be noted: first, the thermal stability can be represented using the equation: Δ = E/kBT,33,37 where E = MSHanV/2 is the energy barrier between the two magnetization states, MS is the saturation magnetization, Han is the anisotropy field, V is the volume of the ferromagnetic Co layer, kB is the Boltzmann constant, and T is the temperature. In our experiments, the hysteresis loops of three types of multi-layered films at the same Co thickness (0.9 nm) were measured at same temperature; and the values of MS are approximately equal. Thus, Δ ∝ Han, indicating that the sample of Ta/Pt/Co/Pt-20 with larger Han has higher thermal stability. Second, in order to prove the thermal stability of the Ta/Pt/Co/Pt-20 film, annealing was carried out at two different temperatures of 260 °C and 380 °C for 1 hour in a vacuum furnace at a base pressure of 2.0 × 10−6 torr.38 The out-of-plane hysteresis loops are shown in Fig. 1(d). The square loops indicate that strong PMA and high thermal stability of the Ta/Pt/Co/Pt-20 film can be maintained, even after annealing at 380 °C. This means that the thermal stability of the Ta/Pt/Co/Pt-20 film is far greater than that reported in previous publications.39,40
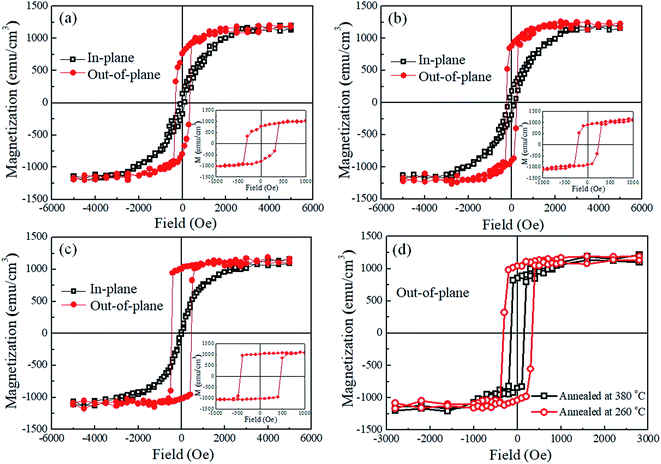 |
| Fig. 1 Hysteresis loops of multi-layered films measured at room temperature: (a) Pt/Co/Pt, (b) Ta/Pt/Co/Pt-4, and (c) Ta/Pt/Co/Pt-20; the insets are the enlarged views of the out-of-plane hysteresis loops. (d) Out-of-plane hysteresis loops of Ta/Pt/Co/Pt-20 annealed at 260 °C and 380 °C, measured at room temperature. | |
The following analysis will focus on the differences between Ta/Pt/Co/Pt-4 and Ta/Pt/Co/Pt-20 from two aspects: (i) the resistivity of the 20 nm Ta sample prepared at a sputtering Ar pressure of 4 mTorr and a sputtering power of 20 W is 2.3 × 102 μΩ cm, which belongs to β-Ta; however, the 20 nm Ta sample fabricated at a sputtering Ar pressure of 20 mTorr and a sputtering power of 8 W displays an ultra-high resistivity (ρ = 3.9 × 105 μΩ cm), exhibiting three orders of higher magnitude than that of the β-Ta. This ultra-high resistivity of the Ta film could be related to the experimental conditions, such as low sputtering power, high sputtering Ar pressure, and ultra-large target-to-substrate distance.41–43 (ii) Fig. 2(a) and (b) present the XRD patterns of the Ta/Pt/Co/Pt samples. (002), (202) and (410) diffraction peaks corresponding to β-Ta were observed for the Ta/Pt/Co/Pt-4 sample [see Fig. 2(a)], while no Ta diffraction peak was observed for the Ta/Pt/Co/Pt-20 sample [see Fig. 2(b)], indicating that Ta is an amorphous structure in the Ta/Pt/Co/Pt-20 sample. These results are consistent with the resistivity data discussed above. In our experiment, the ultra-high resistivity of the Ta film is probably attributed to the amorphous structure and entrapment of Ar ions in the Ta film, resulting from a low deposition rate and high sputtering Ar pressure. For metal films grown by a magnetron sputtering technology, low sputtering power, high sputtering Ar pressure, and large target-to-substrate distance can effectively reduce the deposition rate of metals.42,43 The growth rate of the ultra-high resistance Ta is ∼0.0025 nm s−1, which is far less than that of the β-Ta film (∼0.0115 nm s−1). This low growth rate as well as high Ar pressure result in an amorphous structure of Ta, characterized by XRD pattern, as shown in Fig. 2(b). Also, the high-resolution transmission electron microscopy and fast Fourier transform (FFT) exhibited that the Ta layer is an amorphous state in our previous publication.18 On the other hand, the entrapment of Ar ions in the sputtering process is mainly due to the incorporation of Ar ions.42 Owing to the low sputtering power and high sputtering Ar pressure, the mean free path of the molecules is so small that the low-energy Ar ions will undergo numerous collisions with gas molecules in the sputtering chamber and lose energy with a large target-to-substrate distance, causing some Ar atoms doped into the Ta film.42,44 In addition, the Ar element in the ultra-high resistivity Ta film was detected via XPS, as shown in the inset of Fig. 2(b). The charge-shifted spectrum was corrected using the maximum of the adventitious C 1s signal at 284.8 eV. A distinct binding energy peak of Ar 2p at 241.3 eV indicates the formation of the Ar impurity in the Ta film. One note should be made about the surface roughness of Ta films. From Fig. 2(c) and (d), one can see that the β-Ta and ultra-high resistivity Ta films have smooth surfaces with a roughness of 0.236 nm and 0.269 nm, respectively. Thus, both can be used as underlayers to grow Pt/Co/Pt multi-layered films.
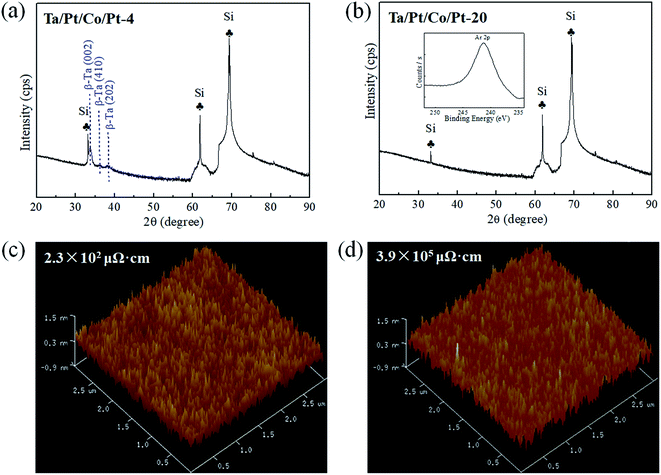 |
| Fig. 2 The XRD patterns for Ta/Pt/Co/Pt-4 (a) and Ta/Pt/Co/Pt-20 (b) multi-layered films. AFM images for the β-Ta film with ρ = 2.3 × 102 μΩ cm (c) and the ultra-high resistivity Ta film with ρ = 3.9 × 105 μΩ cm (d). The inset of (b) shows the Ar 2p core-level XPS spectrum of the Ta/Pt/Co/Pt-20 film. | |
Fig. 3 shows the anomalous Hall curves of the Ta/Pt/Co/Pt-4 and Ta/Pt/Co/Pt-20 films with different currents under a perpendicular magnetic field, using the configuration shown in the inset of Fig. 3(a). It can be seen that both Hall loops exhibit high squareness and the values of the anomalous Hall resistance (RH) remain constant with the change in current, suggesting that the anomalous Hall effect in the Ta/Pt/Co/Pt samples is not very sensitive to heating. The coercivity of the Ta/Pt/Co/Pt-20 multi-layered film reaches 210 Oe, which is significantly higher than that of Ta/Pt/Co/Pt-4 (about 96 Oe), indicating that the multi-layered film with the ultra-high resistivity Ta underlayer possesses stronger PMA and better thermal stability. This result is consistent with the coercivity variation trend of the magnetic hysteresis loops in Fig. 1(b) and (c). In case of the Ta/Pt/Co/Pt-20 film, the presence of the ultra-high resistivity Ta underlayer improves the electron additional scattering at the amorphous Ta/Pt interface, thereby effectively enhancing the PMA and thermal stability of the film.45 Meanwhile, the ultra-high resistivity reduces the partial current of the Ta layer in the multi-layered film and increases the partial current in the magnetic metal Co layer.
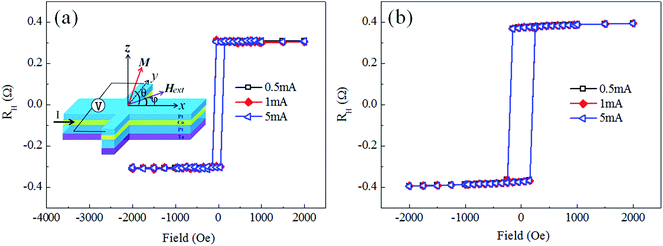 |
| Fig. 3 The anomalous Hall curves of (a) Ta/Pt/Co/Pt-4 and (b) Ta/Pt/Co/Pt-20 samples. The inset shows the configuration of anomalous Hall and plane Hall measurements on the multi-layered Hall bar structure. The magnetic field (Hext) and the current (I, along the x axis) are indicated; and φ is the angle between Hext and the x axis; θ is the angle between the magnetization vector M and the x axis. | |
To further analyze the influence of ultra-high resistivity on the PMA of the films, the planar Hall effect of the Ta/Pt/Co/Pt-4 and Ta/Pt/Co/Pt-20 films was measured and the value of Han was calculated. As shown in the inset of Fig. 3(a), in our measurements an in-plane external magnetic field (Hext) was applied within the x–z plane at a small angle of 7° with respect to the x axis. Fig. 4 shows the planar Hall curves of Ta/Pt/Co/Pt-4 and Ta/Pt/Co/Pt-20 at different temperatures with the small current of 0.1 mA. The magnetic moment of the magnetic metal Co gradually rotates back and forth between the out-of-plane and in-plane directions when Hext is swept along the x axis between ±1.5 T. The planar Hall resistance (RPH) approaches a fixed value at high fields, suggesting that the direction of the magnetic moment is nearly parallel to the film plane. The changing trend of RPH with temperature is similar to that reported by Hao et al. in the Ta/CoFeB/MgO structure.46 Han at different temperatures can be calculated according to the following equation when a current of nearly zero is applied to the Hall bar:18,46
|
Hext = Han sin(θ)cos(θ)/sin(θ − φ)
| (1) |
where
θ is the angle between the sample film plane and the magnetic moment, and
φ is the angle between the external magnetic field and film plane, as shown in the inset of
Fig. 3(a). According to
Fig. 4,
Hext can be plotted as a function of sin(
θ)cos(
θ)/sin(
θ −
β). A complete linear relationship is observed at different temperatures, as expected from
eqn (1). The values of
Han can be obtained from the slopes of the fitted line.
11,46 Fig. 5 shows the values of
Han of the two samples with temperatures. It can be seen that
Han increases with the decrease in temperature, but the values of the Ta/Pt/Co/Pt-20 sample remain larger than that of the Ta/Pt/Co/Pt-4 sample over the entire temperature range.
Han of the Ta/Pt/Co/Pt-20 sample reaches 1.1 T at 10 K. The estimated value of
Han of the Ta/Pt/Co/Pt-20 at 50 K is 9083 Oe, which is nearly twice as high as that of the Ta/Pt/Co/Pt-4 sample (
Han = ∼4620 Oe). These results confirm that the insertion of the ultra-high resistivity Ta underlayer greatly improves the PMA and thermal stability of the Pt/Co/Pt film. It is worth noting that in the Ta/Pt/Co/Pt multi-layered structure the upper and lower layers adjacent to the magnetic metal Co are both Pt. When current is applied, the spin direction of the spin-polarized current injected from the upper and lower heavy metal Pt layers are opposite due to same signs of the spin Hall angle in the two Pt layers; then, the torque would almost cancel each other out. Thus, the magnetization switching was not observed in our experiment.
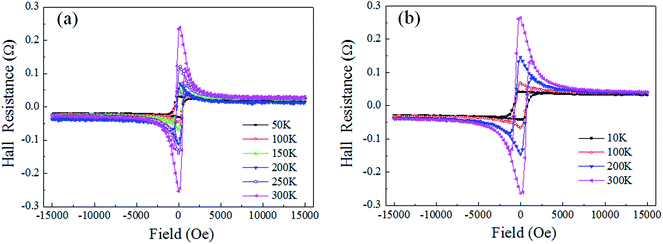 |
| Fig. 4 The plane Hall curves of Ta/Pt/Co/Pt-4 (a) and Ta/Pt/Co/Pt-20 (b) samples. | |
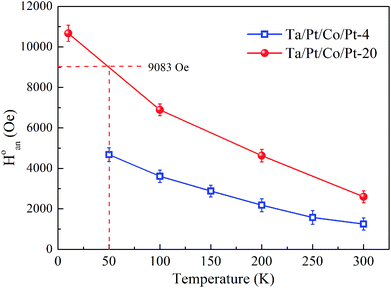 |
| Fig. 5 The values of Han of the Ta/Pt/Co/Pt-4 and Ta/Pt/Co/Pt-20 samples at different temperatures ranging from 10 K to 300 K. | |
Conclusions
In conclusion, the influence of an ultra-high resistivity Ta underlayer on PMA and thermal stability in Ta/Pt/Co/Pt heterostructures were investigated. An optimized amorphous Ta film was obtained at a high sputtering Ar pressure, low sputtering power, and large target-to-substrate distance, exhibiting an ultra-high resistivity (ρ = 3.9 × 105 μΩ cm) of three orders of magnitude higher than that of β-Ta. The Ta underlayer having the ultra-high resistivity increases the additional electron scattering in the Ta/Pt interface, thereby effectively improving the PMA and thermal stability of the magnetic layer. The Han reaches a maximum of 1.1 T at 10 K. These results reveal the inherent relationship between the high resistance heavy metal underlayer and PMA, and provide a new approach to improve the PMA and SOT for heavy metal/magnetic metal multi-layered film structures.
Conflicts of interest
There are no conflicts to declare.
Acknowledgements
This work was supported by the National Natural Science Foundation of China (No. 51571136, 61434002, and 51871137).
References
- I. M. Miron, K. Garello, G. Gaudin, P. J. Zermatten, M. V. Costache, S. Auffret, S. Bandiera, B. Rodmacq, A. Schuhl and P. Gambardella, Nature, 2011, 476, 189–193 CrossRef CAS PubMed.
- S. Ikeda, K. Miura, H. Yamamoto, K. Mizunuma, H. D. Gan, M. Endo, S. Kanai, J. Hayakawa, F. Matsukura and H. Ohno, Nat. Mater., 2010, 9, 721–724 CrossRef CAS PubMed.
- W.-G. Wang, M. Li, S. Hageman and C. L. Chien, Nat. Mater., 2011, 11, 64–68 CrossRef PubMed.
- S. H. Yang, K. S. Ryu and S. Parkin, Nat. Nanotechnol., 2015, 10, 221–226 CrossRef CAS PubMed.
- M. Tang, W. Li, Y. Ren, Z. Zhang, S. Lou and Q. Y. Jin, RSC Adv., 2017, 7, 5315 RSC.
- S. Mangin, D. Ravelosona, J. A. Katine, M. J. Carey, B. D. Terris and E. E. Fullerton, Nat. Mater., 2006, 5, 210–215 CrossRef CAS.
- H. Meng and J.-P. Wang, Appl. Phys. Lett., 2006, 88, 172506 CrossRef.
- S. Yakata, H. Kubota, Y. Suzuki, K. Yakushiji, A. Fukushima, S. Yuasa and K. Ando, J. Appl. Phys., 2009, 105, 07D131 CrossRef.
- L. J. Zhu, S. H. Nie, K. K. Meng, D. Pan, J. H. Zhao and H. Z. Zheng, Adv. Mater., 2012, 24, 4547–4551 CrossRef CAS PubMed.
- N. Nagaosa and Y. Tokura, Nat. Nanotechnol., 2013, 8, 899–911 CrossRef CAS PubMed.
- L. Q. Liu, C.-F. Pai, Y. Li, H. W. Tseng, D. C. Ralph and R. A. Buhrman, Science, 2012, 336, 555–558 CrossRef CAS PubMed.
- M. Tang, R. Ramaswamy, H. Yang, H. Yang, W. Fan, Z. Shi, S. Zhou and X. Qiu, Appl. Phys. Lett., 2018, 113, 222406 CrossRef.
- X. Qiu, Z. Shi, W. Fan, S. Zhou and H. Yang, Adv. Mater., 2018, 30, 1705699 CrossRef PubMed.
- S. Chen, J. Yu, Q. Xie, X. Zhang, W. Lin, L. Liu, J. Zhou, X. Shu, R. Guo, Z. Zhang and J. Chen, ACS Appl. Mater. Interfaces, 2019, 11, 30446–30452 CrossRef CAS PubMed.
- L. Q. Liu, O. J. Lee, T. J. Gudmundsen, D. C. Ralph and R. A. Buhrman, Phys. Rev. Lett., 2012, 109, 096602 CrossRef PubMed.
- P. Li, T. Liu, H. C. Chang, A. Kalitsov, W. Zhang, G. Csaba, W. Li, D. Richardson, A. DeMann, G. Rimal, H. Dey, J. S. Jiang, W. Porod, S. B. Field, J. K. Tang, M. C. Marconi, A. Hoffmann, O. Mryasov and M. Z. Wu, Nat. Commun., 2016, 7, 12688 CrossRef CAS PubMed.
- G. Y. Shi, C. H. Wan, Y. S. Chang, F. Li, X. J. Zhou, P. X. Zhang, J. W. Cai, X. F. Han, F. Pan and C. Song, Phys. Rev. B, 2017, 95, 104435 CrossRef.
- R. Wang, Z. Y. Xiao, H. H. Liu, Z. Y. Quan, X. Zhang, M. M. Wang, M. Z. Wu and X. H. Xu, Appl. Phys. Lett., 2019, 114, 042404 CrossRef.
- C. Zhang, S. Fukami, K. Watanabe, A. Ohkawara, S. DuttaGupta, H. Sato, F. Matsukura and H. Ohno, Appl. Phys. Lett., 2016, 109, 192405 CrossRef.
- W. Skowroński, M. Cecot, J. Kanak, S. Ziętek, T. Stobiecki, L. Yao, S. van Dijken, T. Nozaki, K. Yakushiji and S. Yuasa, Appl. Phys. Lett., 2016, 109, 062407 CrossRef.
- C.-F. Pai, L. Q. Liu, Y. Li, H. W. Tseng, D. C. Ralph and R. A. Buhrman, Appl. Phys. Lett., 2012, 101, 122404 CrossRef.
- Q. Hao, W. Z. Chen and G. Xiao, Appl. Phys. Lett., 2015, 106, 182403 CrossRef.
- G. W. Kim, A. S. Samardak, Y. J. Kim, I. H. Cha, A. V. Ognev, A. V. Sadovnikov, S. A. Nikitov and Y. K. Kim, Phys. Rev. Appl., 2018, 9, 064005 CrossRef CAS.
- Y. Takeuchi, C. L. Zhang, A. Okada, H. Sato, S. Fukami and H. Ohno, Appl. Phys. Lett., 2018, 112, 192408 CrossRef.
- M. Cecot, Ł. Karwacki, W. Skowroński, J. Kanak, J. Wrona, A. Żywczak, L. Yao, S. van Dijken, J. Barnaś and T. Stobiecki, Sci. Rep., 2017, 7, 968 CrossRef PubMed.
- G. Allen, S. Manipatruni, D. E. Nikonov, M. Doczy and I. A. Young, Phys. Rev. B: Condens. Matter Mater. Phys., 2015, 91, 144412 CrossRef.
- J. Kim, J. Sinha, S. Mitani, M. Hayashi, S. Takahashi, S. Maekawa, M. Yamanouchi and H. Ohno, Phys. Rev. B: Condens. Matter Mater. Phys., 2014, 89, 174424 CrossRef.
- X. P. Qiu, P. Deorani, K. Narayanapillai, K. S. Lee, K. J. Lee, H. W. Lee and H. Yang, Sci. Rep., 2014, 4, 4491 CrossRef PubMed.
- D. Li, B. S. Cui, T. Wang, J. J. Yun, X. B. Guo, K. Wu, Y. L. Zuo, J. B. Wang, D. Z. Yang and L. Xi, Appl. Phys. Lett., 2017, 110, 132407 CrossRef.
- B. S. Cui, S. W. Chen, D. Li, J. J. Yun, X. B. Guo, K. Wu, X. Zhang, Y. P. Wang, Y. L. Zuo, M. Z. Gao and L. Xi, Appl. Phys. Express, 2018, 11, 013001 CrossRef.
- H. L. Ju, P. P. Xiang, W. Wang and B. H. Li, Acta Phys. Sin., 2015, 64, 197501 Search PubMed.
- S. P. Bommanaboyena and M. Meinert, Appl. Phys. Lett., 2017, 111, 042407 CrossRef.
- S. Ikeda, K. Miura, H. Yamamoto, K. Mizunuma, H. D. Gan, M. Endo, S. Kanai, J. Hayakawa, F. Matsukura and H. Ohno, Nat. Mater., 2010, 9, 721–724 CrossRef CAS PubMed.
- H. Sato, M. Yamanouchi, S. Ikeda, S. Fukami, F. Matsukura and H. Ohno, Appl. Phys. Lett., 2012, 101, 022414 CrossRef.
- B. Cui, C. Song, G. A. Gehring, F. Li, G. Wang, C. Chen, J. Peng, H. Mao, F. Zeng and F. Pan, Adv. Funct. Mater., 2015, 25, 864–870 CrossRef CAS.
- Z. Y. Xiao, F. Zhang, M. A. Farrukh, R. Wang, G. W. Zhou, Z. Y. Quan and X. H. Xu, J. Mater. Sci., 2019, 54, 9017–9024 CrossRef CAS.
- O. J. Lee, L. Q. Liu, C. F. Pai, Y. Li, H. W. Tseng, P. G. Gowtham, J. P. Park, D. C. Ralph and R. A. Buhrman, Phys. Rev. B: Condens. Matter Mater. Phys., 2014, 89, 024418 CrossRef.
- S. Chen, J. Zhou, W. Lin, J. Yu, R. Guo, F. Poh, D. Shum and J. S. Chen, J. Phys. D: Appl. Phys., 2018, 51, 055006 CrossRef.
- P. Sethi, S. Krishnia, S. H. Li and W. S. Lew, J. Magn. Magn. Mater., 2017, 426, 497 CrossRef CAS.
- M. Furuta, Y. Liu, H. Sepehri-Amin, K. Hono and J. G. Zhu, J. Appl. Phys., 2017, 122, 113901 CrossRef.
- M. Grosser and U. Schmid, Thin Solid Films, 2009, 517, 4493–4496 CrossRef CAS.
- W. W. Y. Lee, J. Appl. Phys., 1971, 42, 4366 CrossRef CAS.
- E. Krikorian and R. J. Sneed, J. Appl. Phys., 1966, 37, 3674 CrossRef CAS.
- E. V. Kornelsen, Can. J. Phys., 1964, 42, 364–381 CrossRef CAS.
- N. Ryzhanova, A. Vedyayev, A. Pertsova and B. Dieny, Phys. Rev. B: Condens. Matter Mater. Phys., 2009, 80, 024410 CrossRef.
- Q. Hao and G. Xiao, Phys. Rev. B: Condens. Matter Mater. Phys., 2015, 91, 224413 CrossRef.
|
This journal is © The Royal Society of Chemistry 2020 |
Click here to see how this site uses Cookies. View our privacy policy here.