DOI:
10.1039/C9RA10794K
(Paper)
RSC Adv., 2020,
10, 7170-7180
Characterization of biocompatible pig skin collagen and application of collagen-based films for enzyme immobilization
Received
22nd December 2019
, Accepted 6th February 2020
First published on 18th February 2020
Abstract
Based on the excellent biocompatibility of collagen, collagen was extracted from pig skin by acid-enzymatic method. The films were prepared by the self-aggregation behavior of collagen, and the catalase was immobilized by adsorption, cross-linking and embedding. The experiment investigated the effects of glutaraldehyde on the mechanical properties, external sensory properties, and denaturation temperature of the films. The results showed that self-aggregating material could maintain the triple helix structure of pig skin collagen. The self-aggregation treatment and cross-linking treatment can improve the mechanical properties to 53 MPa, while the glutaraldehyde cross-linking agent can increase the denaturation temperature of the pig skin collagen self-aggregating membrane by 20.35% to 84.48 °C. This means that its application to immobilized catalase has better stability. The comparison shows that the catalase immobilized by the adsorption method has strong activity and high operational stability, and the cross-linking agent glutaraldehyde and the initial enzyme concentration have a significant effect on the immobilization, and the activity can reach 175 U g−1. After 16 uses of the film, the catalase was completely inactivated. This study provides a reference for the preparation of a catalase sensor that can be used to detect hydrogen peroxide in food by a catalase sensor.
Introduction
A ubiquitous enzyme called catalase, is involved in oxidative stress protection. The hydrogen peroxide is usually produced by a superoxide dismutase in the mitochondria. The substrate of this process is the anion superoxide which is extremely harmful. Catalase contains a heme cofactor in active site and it converts hydrogen peroxide to harmless oxygen and water.1 Catalase has found a place in every field of the biological sciences, because of many unique features. Catalase is one of the enzymes which has the highest turnover number with an isoelectric point of 5.4 and a molecular weight of 240 kDa.2 Enzymes have a high degree of selectivity, act under mild conditions and produce a reduced number of undesirable by-products compared to chemical catalysts. Therefore, enzymes have been used to generate high value chemicals from a low-cost start.3 Catalase has several applications in food, medicine, bioremediation, and textile industries.4 Moreover, the cosmetic industry uses it in the skin formulations as an antioxidant or together with hydrogen peroxide in facial masks to increase cellular oxygenation in the epidermis. Catalase, used for removing hydrogen peroxide from milk in the food industry, is one of an essential commercial catalysts.5 But, catalase is typical of some enzymes, and exhibits a shorter period of use, poor operational stability and reusability. Therefore, its potential application has been limited.6 With immobilized enzymes, improved stability, reuse, continuous operation, better control of reactions, high yield and high quality has been achieved and it has been expected to bring more favorable economic costs.7 Research shows that the immobilized catalase showed higher activity concerning the free catalase in the lower temperature and acidic region and immobilization can enhance the enzyme activity in the initial pH region.8 Thus, immobilization, of late, has been receiving considerable attention as a widely used strategy to promote enzymes in the bulk scale.
There are three main methods for enzyme immobilization, including cross-linking, adsorption, and embedding, but none is generally valid for a specific enzyme.9 Different immobilization methods indicate the different interaction between enzyme and the carrier material.10 The activity of immobilized catalase is affected by the immobilization method. The activity of catalase is closely related to the subunits. Preventing the dissociation of the subunits and achieving the characteristics of the enzyme are the important requirements for immobilizing catalase.11 However, common methods such as cross-linking, adsorption, and embedding cause different activities of immobilized enzymes due to different mechanisms of action.12 Therefore, studying the effects of different methods on immobilized peroxidase and optimizing the relevant parameters of different methods of immobilized peroxidase are important for collagen immobilized catalase.13 There are few studies on collagen immobilized catalase.
Besides immobilization techniques, another affecting factor which is of vital importance for enzymes immobilization is the support material.10 Catalase has been fixed on various carriers and widely used in industrial fields. Synthetic polymers, natural macromolecule materials and inorganic compounds are used as carrier materials in many immobilization processes.2 In many studies, catalase was immobilized on substrates such as poly(2-hydroxyethyl methacrylate-glycidyl methacrylate) cryogel,2 graded macroporous/mesoporous silica sphere.14 Studies have shown that immobilized system exhibits high activity recovery, and possesses considerable reusability. However, the immobilization process hinders the contact of enzyme substrates, thus reducing the catalytic efficiency of the enzyme.
In recent years, collagen is expanding its use in food, cosmetics, and biomaterials due to its wide range of sources and high cell compatibility.15 Collagen is a main constituent of the extracellular matrix and therefore the most abundant protein in the human body.16 The most common types of collagen are types I and III, which are comprising approximately 90% of total collagen.17 Collagen molecules have excellent properties such as biodegradability, weak antigenicity, good biocompatibility, and they have unique self-assembled fibril formation characteristics.18 In addition, type I collagen molecules with a triple helix structure of 1.5 nm in diameter and 300 nm in length can self-assemble through hydrophobic, electrostatic and hydrogen bond interactions to form well-organized fibers under physiological conditions. These collagen fibrils with regions of loosely packed collagen and areas of densely packed collagen contain a periodic cross-striated structure (D-periodicity).19 Type I collagen is a rich protein in animal tissues. It is a heterotrimeric trimer of alpha chain and interweaves into a triple helix structure. Self-assembly is its inherent characteristic. Through this characteristic, free collagen can spontaneously form fibrils similar to those in vivo in vitro, making it possible to manufacture different products. This is the reason why type I collagen used widely in biological materials and tissue engineering.20 Therefore, the use of collagen self-aggregation behavior to prepare biological materials for immobilized enzymes has broad development prospects. Type I collagen was most prevalent and found in connective tissues such as skin, bone, and cornea of the eyes.21
Collagen fiber is a rich biopolymer natural material used as a support matrix for immobilized catalase. Collagen has unique biocompatibility to enzymes that cannot be replaced by other substrates, which is unmatched by other inorganic metabolisms. It is important that collagen can adjust its conformation when it comes into contact with the substrate. Therefore, the use of collagen immobilized enzyme can effectively maintain the enzyme activity, and can adjust its conformation to adapt to the geometric size of the enzyme, and achieve high efficiency of the immobilized enzyme.22 The scholar immobilized catalase on zirconium(IV)-modified collagen fibers by adsorption. Immobilized catalase catalyzes the decomposition of hydrogen peroxide with enhanced temperature and pH stability, as well as better reusability and storage stability.23 Li et al. added hemoglobin and catalase to the collagen film modified on the pyrolytic graphite electrode. The results show that the heme protein in the collagen film maintains its nearly natural conformation, and the heme protein-collagen film has good electrocatalytic properties. It fully shows that collagen has excellent biological compatibility, and the catalase-bound film has good stability. Collagen films have great potential for the preparation of novel biosensors or bioreactors based on direct electrochemistry of proteins.24
Usually, collagen has been extracted from mammals, cattle and pig skin, for food, cosmetic.25,26 Affected by religion, disease, and thrifty society, collagen extraction research has gradually turned to by-products of other animals. There are many studies on extracting collagen from gutted silver carp,27 ovine bones,26 scales of tilapia,28 etc. to improve waste utilization. But pig skin collagen is still the main source of commercial collagen.29 It is estimated that 2 billion tons of waste are generated from fisheries and meat (bovine, pig, and poultry) residues worldwide each year, of which the fluoride produced by meat can reach 82.5%.30 The problem of meat waste is more urgent and more widespread than fish. Recycling animal residues at the molecular level and applying them to medicine, cosmetics, etc. to achieve high-value transformation may be a feasible and more profitable option. In the international frame, China emerges as the main producer of collagen. It can be seen that the extraction of collagen from a large amount of waste such as pigs and cattle has broad development prospects.31 Immobilization of extracted pig skin collagen catalase can effectively improve the high value utilization of pig skin tibia.
In this study, unlike collagen or enzymatic extraction of collagen, we extracted collagen from collagen-rich pig skin by acid-enzyme binding. Based on the self-aggregation behavior of collagen, an immobilized hydrogen peroxide membrane is prepared by adsorption, embedding and cross-linking, thereby improving catalase stability and the number of repeated uses. The best method for selecting the best pig skin collagen immobilized catalase is to provide a reference for its application to immobilized catalase. We tested the mechanical properties, external sensory properties, scanning electron microscopy (SEM) and Fourier transform infrared spectroscopy (FT-IR) characteristics of pig skin collagen self-aggregating membrane materials, and studied different immobilization methods for immobilized peroxidation. The effects of hydrogenase activity and operational stability. In addition, the parameters of the optimal immobilization method such as enzyme concentration and glutaraldehyde concentration were optimized, which provided theoretical basis and data reference for the application of collagen materials.
Materials and methods
Materials
The raw pig skin we used was purchased from the local market in Ya'an City, Sichuan Province, China. The pig skin was cleaned, removed hair, scraped fat, and add sodium dodecylbenzene sulfonate (SDBS, 0.75%) for degreasing in an ultrasonic cleaner (pig skin
:
SDBS = 1
:
2.5, 25 °C, 120 W) after chopping and soaked by NaCl (1%, 6 h) to remove other protein.
We used sodium chloride (NaCl), acetic acid, sodium dihydrogen phosphate, sodium dodecylbenzene sulfonate (SDBS) glutaraldehyde, dibasic sodium phosphate from Chengdu Kelong Reagent Co., China. All other chemicals and solvents were of reagent grade or higher purity and were purchased from Yan'an Wanke Reagent Co., China, unless otherwise indicated. The pepsin (biological reagent, 99%, initial enzyme activity 10
000 U g−1) and catalase (biological reagent, 99%, initial enzyme activity 2000–5000 U mg−1, from bovine liver) we used came from Shandong Fengtai Biotechnology Co., Ltd., and Shanghai Meiruil Chemical Technology Co., Ltd., China, respectively.
Extraction of collagen from pig skin
According to Liu et al.,32 the extraction of collagen mainly consists of three steps. First, the pig skin is enzymatically acidified with acetic acid and pepsin, followed by salting out, and finally purification. The collagen was extracted from pigskin by stirring at 4 °C for 18 h using pepsin in acetic acid (pH = 2.2, 0.5 mol L−1) as a substrate for enzymatic hydrolysis. The slurry is filtered and the supernatant is removed. Add NaCl to the above supernatant and stir until it dissolves, salting out at 4 °C for 8–12 h. After centrifugation (10
000 rpm, 10 min), the precipitates were taken out. Finally, the precipitate was dissolved in acetic acid (0.5 mol L−1, pH 2.2). The solution was dialyzed with acetic acid (0.1 mol L−1, 48 h) and ultrapure water (24 h), then lyophilized to obtain the target product.
Preparation of self-aggregating collagen film and testing
The lyophilized collagen is used as a solute, and the 0.5 mol L−1 acetic acid solution is used as a solution, and the 1% collagen solution is prepared by fully dissolving at 4 °C. The solution was placed in a dialysis bag and dialyzed for 24 h at 4 °C in phosphate buffer (PBS) contained 150 mmol L−1 NaCl at pH 7.0. Second, the sample in the dialysis bag was self-aggregated at 30 °C for 10 h to obtain a film-forming solution after self-aggregation. Finally, 10 mL of the film forming solution was cast onto a polytetrafluoroethylene plate and dried at 30 °C to form a film. After film formation, 10 mL of glutaraldehyde (3%) solution was added dropwise to the membrane for fixed cross-linking. After air drying for 1 h, it was washed with distilled water, and after drying, a self-aggregating film of pig skin collagen was obtained. Before testing all the samples, put them in a constant temperature and humidity chamber (25 °C, RH 50%, LS-TH-80Z, Dongguan Laisi Test Equipment Co., Ltd., Dongguan, China) to balance for 24 h.
Thickness. The thickness of the film was measured by hand-held micrometers (ACE-H1012, Dongguan city Express Measuring Instrument Co., Ltd., Dongguan, China) with a sensitivity of 0.0001 mm at 5 random positions for each sample.
Film sensitivity index. In the absence of external interference, the external sensory properties of pig skin collagen self-aggregating films were roughly evaluated, including the color, odor, transparency, solubility in water and removal of the film from the Teflon plate.33
Transmittance. Transmittance through the films (10 mm × 30 mm) was recorded between 190 and 1100 nm on an ultraviolet-visible spectrophotometer (α1860, Shanghai Puyuan Instrument Co., Ltd., Ltd. Shanghai, China). The formula for calculating the transmittance is as follows. Five replications were done for each treatment.34
Transmittance (%) = 102−A |
where A is the absorbance value at 500 nm.
Moisture absorption. The films, dried at 40 °C for three days in a vacuum oven, were placed inside an environmental chamber maintained at 75% relative humidity (RH) and 25 °C, to obtain water sorption kinetics. Samples were taken out of the chamber at regular time intervals and weighed with a precision of ±0.01 g. To ensure the repeatability of the results, four specimens for each sample were tested. The equilibrium moisture content of the films was calculated as follows:35
Moisture absorption = (m2 − m1)/m1 × 100% |
where m1 is the initial (dry) weight of the sample (g), m2 is the mass at which the sample absorbs moisture to reach equilibrium, after about 6 hours (g).
Mechanical properties. The tensile strength (TS) and elongation (E) at the break of films were tested by a texture analyzer (TX-700, Lai Lejin Supply Chain (Shenzhen) Co., Ltd., Shenzhen, China). Carefully cut each sample into the appropriate size (10 × 80 mm), install it, clamp it between the stretching fixtures, and stretch it at a speed of 5 mm s−1, the stretching distance is 50 mm and the triggering force is 5 N. Record the maximum tensile force and extension distance of each film at break, and test each sample eight times. The TS was calculated as follows:36
where TS is tensile strength (kPa); Fm is the maximum tension of film at break (N); S is cross-sectional area of the film (mm2). The E was counted by the following equation:
where E is elongation at break (%); ΔL is extension distance of each film at break (mm); L is fixture distance (mm).
Fourier transform infrared spectroscopy (FT-IR) and differential scanning calorimeter (DSC)
Instruments (FT-IR 6600, Jiangsu Tianrui Instruments Co., Ltd., Jiangsu, China) are used to record FTIR of thin films, equipped with attenuated total reflection accessories. All samples were dried to constant weight in a constant temperature and humidity oven (25 °C, RH 50%), and a flat, non-destructive, uniform film was placed on the annex. The parameters of the infrared spectrometer are set as follows: the scanning range is 4000–650 cm−1, and the resolution is 4 cm−1. After background correction, all spectra were baseline corrected, and then spectra were obtained.37 Thermal analysis a differential scanning calorimeter (DSC) was performed to examine the thermal properties of films, using a piece of equipment (Q200, American TA Instruments, American). The samples were conditioned in hermetic aluminum pans, with a sample weight around 1–2 mg. The test was implemented at a heating rate of 5 °C min−1 from 20 to 100 °C, in inner atmosphere of N2.38
Pig skin collagen self-aggregating film material immobilized catalase and its performance test
Immobilization of catalase by self-aggregation of pork skin collagen film was realized by three methods: adsorption, cross-linking and embedding. (I) Adsorption method: the prepared, dry and undamaged collagen self-aggregating film material was placed in 50 mL enzyme solution (catalase enzyme powder dissolved in PBS solution, the same as below, 0.01 mg mL−1) to adsorb and immobilize catalase for 24 h at 4 °C. After ventilation and drying, the collagen film material immobilized by adsorption method was obtained. (II) Cross-linking method: 10 mL of the catalase enzyme solution was added dropwise to the dried collagen self-aggregating film, which was cast from a self-aggregating collagen solution onto a Teflon plate and dried in a 30 °C incubator (DHG-9162, Shanghai Yiheng Technology Co., Ltd., Shanghai, China) to form a film. After natural drying, the film material immobilized catalase by cross-linking method is prepared by glutaraldehyde fixed cross-linking (3%), ventilated, dried, and then dried. (III) Embedding method: the film sample was prepared by mixing pig skin collagen self-aggregating membrane solution with catalase solution (0.1 mg mL−1). The film was cast onto Teflon board and dried at room temperature to obtain the film. After glutaraldehyde crosslinking (3%), ventilation and drying, distilled water washing and drying again, the immobilized CAT film material was obtained by embedding method. All prepared samples were stored in a refrigerator (BCD-258WDPM, Qingdao Haier Co., Ltd., Qingdao, China) at 4 °C for use.
The catalase activity. The activity of immobilized catalase was determined by spectrophotometry. The catalase activity was calculated by the rate of change of absorbance of hydrogen peroxide solution (0.05 mol L−1) at 240 nm for 1 min, during which the activity followed the first-order reaction kinetics. A catalase active unit is defined as the decomposition of 1 μmol hydrogen peroxide per minute at 25 °C and pH 7.0.8 Prepare 0.02, 0.03, 0.04, 0.05, 0.06 mol L−1 hydrogen peroxide solution accurately by PBS, the absorbance at 240 nm was tested respectively, choose the absorbance value as the ordinate, the solution concentration as the abscissa, and absorbance standard and the solution concentration curve were plotted. The assay was terminated when the relative activity of the immobilized enzyme was close to 10% or its activity was 0.
Catalase activity (U g−1) = V × (C0 − C1) × 103/(t × m) |
where V is the volume of hydrogen peroxide (mL), t is the reaction time (min), m is the mass of the immobilized carrier (g), C0 is the initial concentration of hydrogen peroxide (mol L−1), C1 is the concentration of hydrogen peroxide after reaction (mol L−1).
Immobilized catalase operational stability. At room temperature, the collagen film immobilized enzyme with 3 g was added to 30 mL PBS solution (0.05 mol L−1 H2O2). After 3 minutes of reaction, the immobilized collagen film was removed. After washing with PBS, repeat the test under the same conditions, and the immobilized enzyme activity after each repeated experiment was measured to judge the operational stability of the immobilized enzyme.5
SEM. Surface morphologies of collagen film and collagen/catalase films were observed using field emission scanning electron microscopy (SU8010, Hitachi High-Technology Corp., Tokyo, Japan) with an accelerating voltage of 2 kV. The samples were sputtered with gold on their surfaces in a vacuum before testing.39
Statistical analysis
All assays were conducted on at least three replicates and the results were recorded as mean ± standard deviation (SD). IBM Statistics SPSS 22 statistical software was used for the analysis of variance (ANOVA) of the data. Duncan's test was utilized to determine the differences in the mean values (p < 0.05).
Results and discussion
Influence of collagen appearance quality of the pig skin film
Table 1 shows the influence of glutaraldehyde on the sensory performance of pig skin collagen self-aggregating film materials. With the change in glutaraldehyde content from 5% to 25%, the film materials thickness continued to increase, while the moisture absorption and transmittance changed minimally. As the glutaraldehyde content increased, the transmittance of the composite film decreased slightly. When the glutaraldehyde content was 25%, the moisture absorption and transmittance were the maximum at 13.83% and 44.46%. All collagen film materials were easily removed from the board, no odor, and solubility was zero. All collagen film materials exhibited yellow, and the lightest color is cross-linked by 5% glutaraldehyde. Tian et al. prepared collagen hydrogels and they noticed that all the hydrogels composed of collagen fibrils were milky and the cross-linked collagen hydrogels showed yellow observed by the naked eye.40 This result could be owing to the polymeric glutaraldehyde which took shape from the self-polymerization of glutaraldehyde molecules. This also implies that the light transmittance rate of the composite film will decrease. Table 1 shows the thickness of films. Film thickness had not change obviously. The thickness of the film increased from 0.0326 mm to 0.0426 mm with the increase in glutaraldehyde. Therefore, the increase in thickness may be related to the crosslinking reaction. At the same time, they also think that thickness is related to temperature. The intermolecular distance of the collagen molecules was tiny under low-temperature circumstance. Therefore, it benefited the interaction between collagen molecules. Collagen's helix structure was loosened gradually with the temperature increased to 35 °C, but there was no chain cracking. The thickness of the film and the distance between the molecules may increase because of those changes.41 The moisture absorption of collagen films was found to be in the range of 13.83–18.98% (Table 1). According to the cross-linked films, the moisture absorption was low in 25% (13.8%). In the current study, the decline water solubility and swelling of collagen–glutaraldehyde films might be caused by the following reason: a high degree of chemical crosslinking occurred between the free aldehyde group (CHO) of glutaraldehyde and the amine group of collagen. Therefore, the hydrophobicity of collagen film become stronger.34 Elango et al.42 also reported that the collagen–sorbitol films' water solubility varied from 6.3% to 67%. The pure collagen films show the highest water solubility among those films. Our collagen film has a water solubility of 0, and its water solubility is superior to collagen–sorbitol films and pure collagen films. In general, collagen and collagen based composites are hydrophilic fibril matrix. And to reduce the water solubility of collagen, the chemical modification of collagen by its cross-linking with glutaraldehyde is common, which has been used for a long time.43 It is generally considered that the interaction between aldehyde groups of glutaraldehyde and e-amino groups of lysine or hydroxylysine of collagen is the major reaction of glutaraldehyde with collagen, and it caused the constitution of a Schiff base type compound. The procedure of linking polymer chains by covalent or non-covalent bonds and forming three-dimensional networks is called as cross-linking. It is these intermolecular crosslinks that confer the insolubility typical of collagenous tissues.43 Tian et al. reported that a closer-knit spatial network structure can be formed by the cross-linked structure catalyzed by glutaraldehyde, which can decrease the intermolecular space. Thus, the diffusion of water molecules and the solubility of films reduced.43
Table 1 The effect of glutaraldehyde on the quality of porcine skin filma
GTA |
Thickness (mm) |
Transmittance (%) |
Moisture absorption (%) |
Remove |
Colour and transparency |
Odor |
Solubility in water |
The different letters (a–d) indicate significant differences between samples (p < 0.05) in the same column, and the values are mean ± SD, the same below. |
5% |
0.0326 ± 0.0029c |
51.68 ± 3.02a |
18.90 ± 0.02a |
Easily |
Light yellow translucent |
No |
No |
10% |
0.0372 ± 0.0080c |
48.75 ± 2.20b |
17.62 ± 0.03b |
Easily |
Yellow translucent |
No |
No |
15% |
0.0392 ± 0.0077c |
45.60 ± 1.75c |
18.81 ± 0.04a |
Easily |
Yellow translucent |
No |
No |
20% |
0.0415 ± 0.0051c |
45.30 ± 2.32c |
16.87 ± 0.03c |
Easily |
Yellow translucent |
No |
No |
25% |
0.0426 ± 0.0092c |
44.46 ± 3.33d |
13.66 ± 0.01d |
Easily |
Yellow translucent |
No |
No |
Effect of glutaraldehyde on TS and E of the pig skin collagen re-assembled fiber film
As we all know, the mechanical properties of films are described as TS and E. The film can be more extensible with higher value of E and more rigid consistently with higher value of TS.41 Collagen has good film-forming ability and extra strength and stability through self-polymerization, so it has been widespread used for various applications. Ideally, the difficulty of preventing collagen molecules from sliding past each other under stress can be solved by the introduction of additional crosslinks, and it also enhances the collagen fibers' mechanical strength.20 Great majority of the studies have been performed with glutaraldehyde as the crosslinker with collagen films the better mechanical properties and lower water solubility of it.42 Fig. 1 shows the data of TS and E. With the change in glutaraldehyde content from 5% to 25%, the film of TS and E continued to increase, while the film of TS and E changed minimally when the glutaraldehyde is greater in 25%. The TS of the shark catfish skin collagen films ranged from 3.5 to 6.4 MPa. It was lower than the pig skin collagen films (>40 MPa). The TS of pig skin collagen is 6 times that of shark collagen.43 The research report of Chen et al. Showed that glutaraldehyde cross-linking significantly improved the mechanical properties of collagen film. Compared with untreated collagen film (TS = 2.3 MPa, E = 52.5%), their TS was increased by more than three times, TS was about 6.5%, but the E of the collagen was significantly reduced. Its TS is lower than pig skin collagen film after glutaraldehyde cross-linking, but its (17%) E is much higher than pig skin collagen film (2%).44 The above observations indicated that glutaraldehyde forms a stable structure in the collagen network by covalent bonds.45 The introduction of crosslinking bonds hindered the intermolecular slippage with the addition of glutaraldehyde, resulting in the decrease of the deformation.46 Cheng et al. reported that the mechanical strength and E of the film are increased by the isopeptide bonds formed by crosslinking, and the mechanical strength of gelatin film was concerning the degree of crosslinking of glutaraldehyde.39 According to the result, we can see that the nature of the cross-linking agent and degree of cross-linking can effect the mechanical properties of collagen films. Collagen self-assembly starts with three amino acid peptides strands of polyproline type II helical structure were used. Then they entangled with each other to form triple helixes, which could be packaged in quasi-hexagonal and interlaced ways to form collagen with nanofibers. Subsequently, collagen fibers and networks are formed by linear and transverse self-assembly. Both crosslinked networks and collagen fiber networks can effectively improve the mechanical properties of collagen-based materials.47,48 This phenomenon can be observed in the research of He et al., but the results show that the mechanical properties of collagen in grass carp scales are lower than those of pig skin collagen film. Different collagen sources, different collagen content and different structures lead to different results.12,32 Studies by Liu et al. show that the degree of collagen aggregation of fish skin, fish scales, and cattle Achilles tendon are different, which are 28.00% ± 0.65%, 27.33% ± 0.41%, and 56.74% ± 1.38%. Obviously, the degree of collagen aggregation of bovine Achilles tendon is much higher than that of collagen extracted from fish, which may be due to the difference between their aggregation state and microstructure. In the study by Tang et al., the TS of tilapia skin, grass carp skin, and silver carp skin were 51.24 ± 2.81 MPa, 35.85 ± 5.68 MPa, and 40.17 ± 4.73 MPa, respectively, and E were 36.71 ± 4.94%, 44.47 ± 6.00%, and 25.90 ± 5.38%, it can be seen that the strength of collagen in tilapia skin is the best, which is better than that of grass carp skin, fish skin, and cross-linked pig skin collagen. This may be due to the plasticizer.23,32 They added 20% glycerol as a plasticizer when preparing the collagen film, which greatly improved the mechanical properties of the collagen film. The ductility of pig skin collagen in the study was significantly poor, which may be due to the reduction of the ductility of pig skin collagen after cross-linking.49 Expansion of the application of collagen film should also give due consideration to the addition of plasticizers.
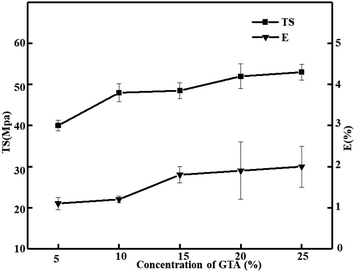 |
| Fig. 1 Effect of glutaraldehyde on TS and E of the pig skin collagen re-assembled fiber film. | |
Effect of glutaraldehyde on DSC of collagen self-assembled fibrous film
The denaturation temperature of pig skin collagen self-aggregating film material changes with the concentration of glutaraldehyde as shown in Table 2. When the highest denaturation is 20% glutaraldehyde, the denaturation temperature of collagen self-aggregating film material is 84.48 °C. Glutaraldehyde can significantly increase the thermal transition temperature of self-assembled film materials compared to collagen films that have not been cross-linked with glutaraldehyde. The improvement in thermal stability upon cross-linking was explained by the denaturation temperature shifting, which was higher with increasing the glutaraldehyde. It is well known that collagen structure and denaturation fibril-forming collagen molecules which is comprised of three similar or identical protein-chains, has a left-handed twist, that are wound to form a supercoiled right-handed triple helical structure.48 Non-covalent interactions (hydrogen bonding, hydrophobic and electrostatic interactions) combining together form the reconstituted collagen fibrils. They are so unstable that the collagen molecules can slide relaxingly and the fibril can easily be dissociated by variations in ionic strength, pH, temperature or collagenase.40 As we all known, thermal stability of col-based scaffolds depends on the density of crosslinking. Marzec et al.41 proposed that the glutaraldehyde influenced collagen structure stabilization is manifested by a shift of both peaks towards higher temperatures, which results in a higher thermo-stability of the collagen–glutaraldehyde scaffolds. Crosslinking by glutaraldehyde tends to abatement the hydrogen-bonded network. Conversely, it can declines energy required to break the bonds formed by the absorbed water with polar regions on the col surface. It same as reported by Tian et al. They think that the transformation from natural triple helix into random coil structure of collagen is reflected by the thermal denaturation temperature, which is due to cross-linking bonds within collagen and hydrogen bonds. A close reason for the improvement in thermal stability was that the alignment of the collagen fibers tended to be dense due to the introduction of cross-linking bonds, and the arrangement between the collagen fibrils was very compact.50 So, the increase of the intermolecular crosslinks among the triple helix structures of collagen had not only limited the degrees of freedom of its α-chain, but also prevented unraveling of the collagen microfibrillar arrangement caused by heating.18 Tian et al. also proposed that the thermal stability of collagen triple helix conformation strongly depends on the inter- and intra-molecular hydrogen bonds and the degree of crosslinking among the chains confirming that crosslinked collagen possessed better thermal stability than the native one.51 The denaturation temperature of uncrosslinked pigskin collagen is 67.75 °C, which is slightly higher than that of grass carp scale collagen prepared and extracted by He et al.12 The denaturing temperature of bovine collagen is 42 °C, the denaturing temperature of shark skin collagen is 37.8 °C, and the temperature of acid-soluble collagen of eel is 39 °C.8,52 Collagens from different sources have different numbers of protein molecules, and the interactions between or within proteins are related to the aggregation of collagen molecules, resulting in different heat resistance and viscosity. The different moisture content of the film, the degree of cross-linking, and the degree of self-aggregation all affect the collagen protein denaturation temperature.12,49,53
Table 2 Denaturation temperature of the pig skin collagen re-assembled fiber filma
GTA |
Denaturation temperature (°C) |
Different letters (a–f) indicate significant differences in the same column among denaturation temperature (p < 0.05) due to glutaraldehyde. |
0% |
67.75 ± 0.79f |
5% |
76.22 ± 0.83e |
10% |
79.16 ± 0.56c |
15% |
78.38 ± 0.61d |
20% |
84.48 ± 0.95a |
25% |
81.54 ± 0.33b |
FTIR of pig skin collagen re-assembled fiber film
As revealed in Fig. 2, the amide A and B peak, respectively corresponding to the stretching vibration of N–H and O–H, appeared at 3300 and 2929 cm−1.36 Collagen's main characteristic bands were amide I, II and III bands. The absorption band at 1630–1660 cm−1 for amide I band was originated from C
O stretching vibration and amide II band at 1550 cm−1 was usually responsible for the combination of N–H bending and C–N stretching vibrations; what's more, 1240 cm−1 was attributed to amide III band for C–N stretching and N–H in-plane bending from amide linkages.48,51 The second derivative of the amide II region showed that the native pig collagen and the prominent peaks at 1550 cm−1, indicative of helical structure.43 It has been used to describe collagen structure. With increasing glutaraldehyde from 5% to 25%, all of the bands of crosslinked collagen nearly unchanged. This indicates that structure of collagen didn't effect by the glutaraldehyde cross-linking and the collagen structure extracted from pig skin remains intact. Furthermore, one of the ways to forecast the preservation of integrity of collagen triple helical structure is the absorption ratio of 1240 cm−1 (amide III band) to 1455 cm−1 (AIII/A1455).51 The value of AIII/A1455 was 1.00, indicating triple helical structure is present, which indicated that the triple helix structure was destructed by glutaraldehyde.
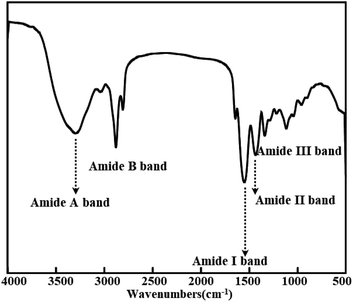 |
| Fig. 2 FT-IR of pig skin collagen re-assembled fiber film. | |
Immobilized catalase by the pig skin collagen re-assembled fiber film
Operational stability and enzyme activity of immobilized catalase with three methods. Fig. 3 shows the enzymatic activity and operational stability of the immobilized catalase using three methods. At the same time, we provide Table 3 for easy reading of relevant data. Immobilized enzymes in industrial applications have many advantages, such as lower quantity of required enzyme, reusability concerning free form, and easier recovery from reaction medium.4 Inanan reported that using 2-hydroxyethyl methacrylate as a synthetic material and chitosan as a natural material to immobilize catalase to synthesize synthetic-natural copolymer at nano-scale, show that the storage stability of the enzyme has be enhanced by immobilization.8 Fig. 3 shows the operational stability and enzyme activity of catalase immobilized by cross-linking, embedding and adsorption. After cross-linking, immobilized catalase activity reached 39 U g−1. At the end of 5th cycle, a decrease of 50% at the original activity was measured. At the end of 8th cycle, the catalase lost its all activity. In physical adsorption, hydrophobic interactions, van der Waals forces, hydrogen bonds and ionic bonds made the enzymes immobilized on the support surface.54 Immobilized catalase activity reached 175 U g−1 by adsorption. A decline of 50% at the original activity was measured at the end of 9th cycle. At the end of 15th cycle, the immobilized catalase obtained 0% of its original activity. Immobilized catalase activity reached 73 U g−1 by embedding. A decline of 50% at the original activity was measured at the end of 2nd cycle. At the end of 5th cycle, the catalase lost it all activity. This immobilization approach is different due to using assembling the carrier in the existence of the soluble biocatalyst in place of prefabricated support materials. In the existence of the enzyme, materials are required to assemble into supramolecular structures such as gels.55 The comparison shows that different immobilization methods have different fixed amounts and operational stability, and the adsorption method is superior to the cross-linking method and the embedding method.
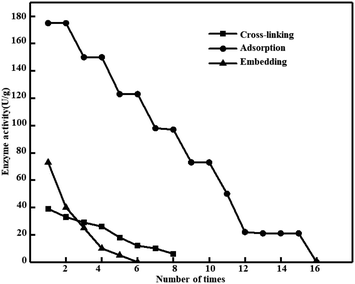 |
| Fig. 3 Operational stability and enzyme activity of immobilized catalase with three methods. (Catalase = 0.1 mg mL−1, glutaraldehyde = 3%, and pH = 7.) | |
Table 3 Operational stability and enzyme activity of catalase immobilized using three methodsa
Methods |
Enzyme activity after use |
Available times |
1 times |
5 times |
10 times |
15 times |
Different letters (a and b) indicate significant differences in the same column among available times (p < 0.05) due to the methods. “—” indicates that the relative activity is less than 10% or the activity is 0, and there is no data state after the experiment is terminated, the same below. (Catalase = 0.1 mg mL−1, glutaraldehyde = 3%, and pH = 7.) |
Embed |
39 ± 1.23 |
18 ± 2.00 |
— |
— |
8 ± 1b |
Adsorption |
175 ± 1.50 |
123 ± 2.00 |
73 ± 2.13 |
21 ± 1.00 |
16 ± 1a |
Cross-linking |
73 ± 1.90 |
5 ± 0.50 |
— |
— |
6 ± 2b |
Adsorption is one of the immobilization methods, which involves reversible interactions between enzyme and carrier (or sorbent). The reversible interaction is formed by weak forces such as van der Waals forces, hydrophobic, electrostatic, and hydrogen bonding interactions. Some obvious advantages had this method have, such as cheapness, comfort, speed and reversibility, and no change to the carrier or enzyme.4 It is speculated that glutaraldehyde is cross-linking inactivation to catalase, and the adsorption method remains high because the catalase is not directly exposed to glutaraldehyde. And the adsorption method is easier and faster to immobilize. Another reason is that the fibrillar structure of collagen became closer-knit by cross-linking and this steric hindrance blocks the immobilization of catalase.32 Inanan et al.4 also reported that it could be due to either conformational changes. Immobilization or lower accessibility of substrate to immobilized catalase caused this situation. Similarly, Song et al. also observed the compatibility of collagen with enzymes. They extract collagen fibers from cowhide by acid method, first chelate collagen fibers with Zr(IV), and then immobilize catalase by adsorption. The immobilized catalase has increased operational stability and can be reused 70 times.22 Compared with the pig skin collagen adsorption immobilized catalase used in this experiment, the pig skin collagen immobilized catalase can only be used 16 times. The reason for this difference may be related to the amount of enzyme added and the initial activity of catalase, and the source of collagen and the purity of extraction may also result in different adsorption amounts and operational stability. Most importantly, collagen has increased its stability and affinity for catalase due to chelation and Zr(IV) reaction. The immobilization of catalase by different materials should be deepened.
Influence of glutaraldehyde concentration on immobilized catalase activity in grass carp scale collagen re-assembled fiber film with adsorption method. Since the adsorption method is the best among the three immobilized catalase enzymes, Fig. 4 presents the impact of glutaraldehyde on the immobilized catalase by adsorption. Increasing the glutaraldehyde concentration, the catalase initial activity increases and, after getting an optimum value, the activity decreases, but the activity is not much different. The catalase shows the maximum activity of 170 U g−1 at 20%, and the catalase shows the minimum activity of 152 U g−1 at 5%. Formation of Schiff base compounds due to glutaraldehyde and ε-amino groups of lysine or hydroxylysine of collagen.43 Tian et al. also reported that the more glutaraldehyde caused the higher the cross-linking degree. In theory, the amino groups should react with the aldehyde groups totally when the [CHO]/[NH2] ratio was 1, however, the cross-linking degree was only 46.79%.43 The reason might be that some hydroxylysine within collagen fibrils or amino groups of lysine were unable to approaching aldehyde groups. And the collagen and glutaraldehyde molecules cannot be uniformly mixed in a short time if the concentration is too high, an uneven reaction will happen. The fibrillar structure of collagen became closer-knit by cross-linking and this steric hindrance blocks the immobilization of catalase.43 Therefore, in the late stage, the concentration of glutaraldehyde has no significant effect on the reaction.
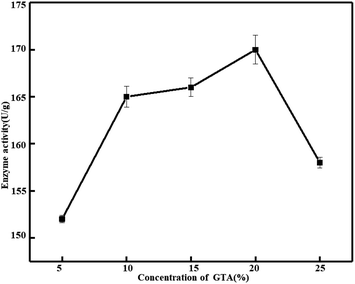 |
| Fig. 4 Effect of concentration of glutaraldehyde on the activity of immobilized catalase. (Catalase = 0.1 mg mL−1, T = 4 °C, and pH = 7.) | |
Influence of enzyme concentration on immobilized catalase activity and operational stability with adsorption method. When the glutaraldehyde is 20%, the enzyme activity is the strongest, then the strength of glutaraldehyde is fixed at 20%, and the impact of initial enzyme concentration on the enzymatic entrapment of catalase is investigated. The impact of the initial catalase concentration on catalase immobilization was determined with various catalase solutions in the range of 0.01–0.1 mg mL−1 and results are showed in Fig. 5. The amount of immobilized catalase was increased with increasing original catalase concentration reached 0.05 mg mL−1 and arrived at a stable value. The maximum catalase amount immobilized in pig skin collagen re-assembled fiber film was determined as 174 U g−1. At the 14th cycle, the catalase lost it all activity (Table 4). As we can see, too much catalase in the structure did not increase in the amount of catalase, thus, at a short-term, more catalase was immobilized onto collagen film materials. Meanwhile, more catalase to attack the collagen film materials because more catalase in the structure, but the large catalase molecules cause a steric hindrance to each other.2 And the fibrillar structure of collagen became closer-knit via cross-linking which hindrance blocks the immobilization of catalase.43 The catalase extracted from grass carp scale is immobilized with catalase, and its immobilized enzyme activity can reach up to 2596 U g−1. After 22 uses, the enzyme activity remains above 50% and can be reused more than 45 times. The maximum activity of collagen immobilized enzyme extracted from pig skin is only 174 U g−1, and all activities are lost after 17 uses. The reason for this difference is that the content of collagen is different, the structure is different, and the ability of collagen to self-aggregate is different. The pig skin collagen uses the adsorption method, which has steric hindrance, resulting in a decrease in enzyme activity, and the immobilized catalase only adsorbs on the surface, resulting in poor durability. The embedding method used for fish scale collagen can embed catalase to the greatest extent and protect catalase well, increasing durability.12,32
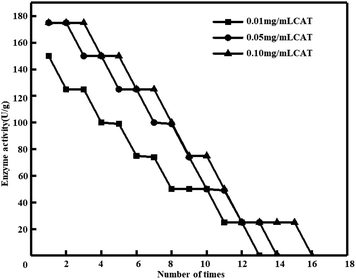 |
| Fig. 5 Influence of enzyme concentration on immobilized catalase activity and operational stability in pig skin collagen re-assembled fiber film. (Glutaraldehyde = 20%, T = 4 °C, and pH = 7.) | |
Table 4 Influence of enzyme concentration on immobilized catalase activity and operational stability in pig skin collagen re-assembled fiber film
CAT |
Enzyme activity after use |
Available times |
1 times |
5 times |
10 times |
15 times |
No significant differences in the same column among available times due to catalase. (Glutaraldehyde = 20%, T = 4 °C, and pH = 7.) |
0.01% |
150 ± 2.00 |
99 ± 1.50 |
50 ± 0.28 |
— |
13 ± 1a |
0.05% |
175 ± 2.13 |
125 ± 2.05 |
50 ± 0.89 |
— |
14 ± 1a |
0.10% |
175 ± 1.07 |
150 ± 2.55 |
75 ± 1.00 |
25 ± 2.10 |
16 ± 1a |
SEM of collagen film collagen and re-assembled fiber film immobilized catalase
SEM micrographs were further used to characterize the cross-section microstructure of films. Fig. 6a is the microstructure of collagen extracted from pig skin after self-polymerization, and Fig. 6b is the collagen of pig skin without self-polymerization. It can be seen that the pig skin collagen is a dense film structure before self-polymerization. The collagen aggregates had a rough and coarse fibrous internal structure because of the collagen fibrils. The difference in the structure before and after aggregation suggests that collagen self-polymerization can significantly alter its ultrastructure. This is because collagen is a typical amphiphilic molecule with a large number of hydrophilic amino acids. As the neutral salt is added to the collagen hydration layer, the charged residue and the hydrophobic group are exposed, resulting in rapid aggregation due to electrostatic forces and hydrophobic interactions.56 Collagen fibrils are supramolecular structures formed by collagen molecules in the body by lateral collection and axial aggregation. The supramolecular structure of collagen fibrils confers biological properties such as good toughness and muscular tensile strength of collagen. It also determines the significant application value of collagen in biology and materials to some extent. Self-polymerization in vitro can improve the mechanical strength, enzyme degradation resistance and thermal stability of collagen biomaterials.45 Fig. 6c is a collagen self-aggregating film treated by 20% glutaraldehyde cross-linking. It indicates that the treatment of cross-linking has a particular destructive effect on the fibrils of collagen. As glutaraldehyde increased, the collagen fibril components in different directions were linked via the cross-linking bonds. As a result, the long-distance collagen fibrils could be linked and almost all the fibrils huddled together.43 Glutaraldehyde is a linear 5-carbon di-aldehyde and is among the most potent known crosslinking agents. It reacts with the primary amine groups of (hydroxyl)-lysine residues in collagen to form intermediate Schiff's bases, which then convert to stable and complex crosslinks. Crosslinking reduces the anisotropy of fibrils and increases the mechanical properties of the film.57 Fig. 6d is an SEM image of the adsorption of catalase from the aggregated film of pig skin collagen. A small amount of enzyme is adsorbed on the film (see red circle for details). This indicates that collagen self-polymerization and immobilization of peroxidase is feasible, but the immobilization rate is low, and it is necessary to deepen the study.
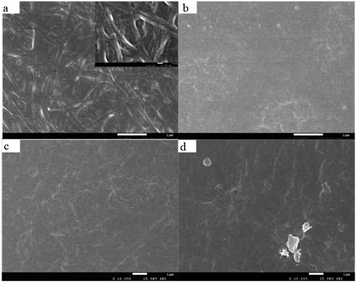 |
| Fig. 6 SEM images of mammalian collagens (×20 000). (a) Pig skin collagen assembled in vitro, (b) pig skin collagen film. Scanning electron micrographs of collagen film (×10 000), (c) 20% glutaraldehyde pig skin collagen self-assembled fiber film, (d) 20% glutaraldehyde pig skin collagen re-assembled fiber film immobilized catalase with adsorption method. | |
Conclusions
In this paper, we extracted collagen from pig skin by acid enzymatic method. Collagen film material was prepared by self-assembled behavior of collagen. The elongation at break and tensile strength of the collagen film material increased with increasing glutaraldehyde. FTIR shows that the triple helix structure of collagen remains intact during self-polymerization and glutaraldehyde cross-linking. DSC data show that neutral salt-induced self-polymerization and glutaraldehyde cross-linking could obviously improve the thermal stability of collagen. The catalase was immobilized by collagen self-aggregating film material, and the three immobilization methods were compared with the immobilized enzyme activity and the number of repeated uses. Among them, the immobilized enzyme activity of adsorption method is the highest, and the operation stability is excellent. While the enzyme reaches 0.1 mg mL−1, the immobilized enzyme activity of pig skin self-polymerization film can reach 175 U g−1, and there is no enzyme activity after 16 times. It is higher than the cross-linking and intercalation method, and both the amount of the enzyme and the concentration of glutaraldehyde affect the activity and operational stability of the immobilized enzyme. The SEM results show that the self-polymerization treatment changes the ultrastructure of collagen and forms the internal structure of the fiber, while self-polymerization can improve the mechanical properties of the collagen film and expand the application range of collagen. Microphotographs of collagen self-aggregating film adsorption immobilized catalase show that catalase was successfully immobilized into the film. This study can provide a reference for immobilized catalase, and it has broad application prospects in catalase sensors.
Conflicts of interest
There are no conflicts to declare.
Acknowledgements
This work was supported by National Natural Science Foundation of China (51703147), National Fund of China Scholarship Council (201806915013), Sichuan Science and Technology Program (2018RZ0034), and Natural Science Fund of Education Department of Sichuan Province (16ZB0044 and 035Z1373).
Notes and references
- C. Bianchi, A. Y. Kostygov, N. Kraeva, K. Zahonova, E. Horakova, R. Sobotka, J. Lukes and V. Yurchenko, Mol. Biochem. Parasitol., 2019, 232, 111199 CrossRef CAS PubMed.
- K. Erol, B. K. Cebeci, K. Kose and D. A. Kose, Int. J. Biol. Macromol., 2019, 123, 738–743 CrossRef CAS PubMed.
- X. Shen, M. Yang, C. Cui and H. Cao, Colloids Surf., A, 2019, 568, 411–418 CrossRef CAS.
- T. Inanan, N. Tuzmen and F. Karipcin, Int. J. Biol. Macromol., 2018, 114, 812–820 CrossRef CAS PubMed.
- N. Misra, N. K. Goel, S. A. Shelkar, L. Varshney and V. Kumar, J. Mol. Catal. B: Enzym., 2016, 133, S172–S178 CrossRef.
- E. Czechowska, K. Ranoszek-Soliwoda, E. Tomaszewska, A. Pudlarz, G. Celichowski, D. Gralak-Zwolenik, J. Szemraj and J. Grobelny, Colloids Surf., B, 2018, 171, 707–714 CrossRef CAS PubMed.
- T. Inanan, React. Funct. Polym., 2019, 135, 94–102 CrossRef CAS.
- E. Basak and T. Aydemir, Artif. Cells, Nanomed., Biotechnol., 2013, 41, 269–275 CrossRef CAS PubMed.
- A. G. Grigoras, Biochem. Eng. J., 2017, 117, 1–20 CrossRef CAS.
- D. Liu, J. Chen and Y. Shi, TrAC, Trends Anal. Chem., 2018, 102, 332–342 CrossRef CAS.
- K. Prakash, S. Prajapati, A. Ahmad, S. K. Jain and V. Bhakuni, Protein Sci., 2002, 11, 46–57 CrossRef CAS PubMed.
- L. He, W. Lan, L. Cen, S. Chen, S. Liu, Y. Liu, X. Ao and Y. Yang, Mater. Sci. Eng., C, 2019, 105, 110024 CrossRef CAS PubMed.
- R. Fernandez-Lafuente, Enzyme Microb. Technol., 2009, 45, 405–418 CrossRef CAS.
- J. Liu, Q. Wang, X. R. Fan, X. J. Sun and P. H. Huang, Appl. Biochem. Biotechnol., 2013, 169, 2212–2222 CrossRef CAS PubMed.
- T. Ikoma, H. Kobayashi, J. Tanaka, D. Walsh and S. Mann, Int. J. Biol. Macromol., 2003, 32, 199–204 CrossRef CAS PubMed.
- D. Dippold, A. Cai, M. Hardt, A. R. Boccaccini, R. E. Horch, J. P. Beier and D. W. Schubert, Mater. Sci. Eng., C, 2019, 95, 217–225 CrossRef CAS PubMed.
- Y. Morikiri, E. Matsuta and H. Inoue, Biochem. Biophys. Res. Commun., 2018, 505, 1168–1173 CrossRef CAS PubMed.
- L. Gu, T. Shan, Y. X. Ma, F. R. Tay and L. Niu, Trends Biotechnol., 2018, 1718, 1–28 Search PubMed.
- L. Shen, H. Bu, H. Yang, W. Liu and G. Li, Int. J. Biol. Macromol., 2018, 115, 635–642 CrossRef CAS PubMed.
- M. Yan and X. Wang, Spectrochim. Acta, Part A, 2018, 203, 342–347 CrossRef CAS PubMed.
- J. Li, M. Wang, Y. Qiao, Y. Tian, J. Liu, S. Qin and W. Wu, Process Biochem., 2018, 74, 156–163 CrossRef CAS.
- N. Song, S. Chen, X. Huang, X. Liao and B. Shi, Process Biochem., 2011, 46, 2187–2193 CrossRef CAS.
- Y. Liu, D. Ma, Y. Wang and W. Qin, Int. J. Biol. Macromol., 2018, 106, 516–522 CrossRef CAS PubMed.
- M. Li, P. He, Y. Zhang and N. Hu, Biochim. Biophys. Acta, Proteins Proteomics, 2005, 1749, 43–51 CrossRef CAS PubMed.
- G. S. Simoes, E. T. Silveira, S. R. Oliveira, E. Poleze, J. R. Allison, E. I. Ida and M. Shimokomaki, Meat Sci., 2014, 96, 1460–1468 CrossRef CAS PubMed.
- L. L. Gao, Z. Y. Wang, Z. Li, C. X. Zhang and D. Q. Zhang, J. Integr. Agric., 2018, 17, 704–711 CrossRef CAS.
- M. Abdollahi, M. Rezaei, A. Jafarpour and I. Undeland, Food Chem., 2018, 242, 568–578 CrossRef CAS PubMed.
- L. Wang, Q. Liang, T. Chen, Z. Wang, J. Xu and H. Ma, Food Hydrocolloids, 2014, 38, 104–109 CrossRef CAS.
- R. Ahmed, M. Haq and B. S. Chun, Int. J. Biol. Macromol., 2019, 135, 668–676 CrossRef CAS PubMed.
- J. Gustavsson, C. Cederberg, U. Sonesson, R. Van Otterdijk and A. Meybeck, Global Food Losses and Food Waste: extent, Causes and Prevention, FAO, Rome, 2011 Search PubMed.
- V. Ferraro, M. Anton and V. Santé-Lhoutellier, Trends Food Sci. Technol., 2016, 51, 65–75 CrossRef CAS.
- S. Liu, Y. Li and L. Li, Carbohydr. Polym., 2017, 160, 62–70 CrossRef CAS PubMed.
- M. Mujtaba, A. M. Salaberria, M. A. Andres and M. Kaya, Int. J. Biol. Macromol., 2017, 104, 944–952 CrossRef CAS PubMed.
- N. Noshirvani, B. Ghanbarzadeh, C. Gardrat, M. R. Rezaei, M. Hashemi, C. C. Le and V. Coma, Food Hydrocolloids, 2017, 70, 36–45 CrossRef CAS.
- M. Pereda, A. Dufresne, M. I. Aranguren and N. E. Marcovich, Carbohydr. Polym., 2014, 101, 1018–1026 CrossRef CAS PubMed.
- L. Sun, B. Li, D. Yao, W. Song and H. Hou, J. Mech. Behav. Biomed. Mater., 2018, 80, 51–58 CrossRef CAS PubMed.
- K. Li, J. Zhu, G. Guan and H. Wu, Int. J. Biol. Macromol., 2019, 122, 485–492 CrossRef CAS PubMed.
- P. Cazon, M. Vazquez and G. Velazquez, Int. J. Biol. Macromol., 2018, 117, 235–246 CrossRef CAS PubMed.
- S. Cheng, W. Wang, Y. Li, G. Gao, K. Zhang, J. Zhou and Z. Wu, Food Chem., 2019, 271, 527–535 CrossRef CAS PubMed.
- Z. Tian, W. Liu and G. Li, Polym. Degrad. Stab., 2016, 130, 264–270 CrossRef CAS.
- E. Marzec and K. Pietrucha, Colloids Surf., B, 2018, 162, 345–350 CrossRef CAS PubMed.
- J. Elango, Y. Bu, B. Bin, J. Geevaretnam, J. S. Robinson and W. Wu, Food Biosci., 2017, 17, 42–51 CrossRef CAS.
- Z. Tian, L. Duan, L. Wu, L. Shen and G. Li, Mater. Sci. Eng., C, 2016, 63, 10–17 CrossRef CAS PubMed.
- X. Chen, L. Zhou, H. Xu, M. Yamamoto, M. Shinoda, I. Tada, S. Minami, K. Urayama and H. Yamane, Int. J. Biol. Macromol., 2019, 135, 959–968 CrossRef CAS PubMed.
- J. Zhang, X. Tu, W. Wang, J. Nan, B. Wei, C. Xu, L. He, Y. Xu, S. Li and H. Wang, Int. J. Biol. Macromol., 2019, 128, 885–892 CrossRef CAS PubMed.
- M. Zou, H. Yang, H. Wang, H. Wang, J. Zhang, B. Wei, H. Zhang and D. Xie, Int. J. Biol. Macromol., 2016, 92, 1175–1182 CrossRef CAS PubMed.
- M. Schroepfer and M. Meyer, Int. J. Biol. Macromol., 2017, 103, 120–128 CrossRef CAS PubMed.
- K. H. Sizeland, K. A. Hofman, I. C. Hallett, D. E. Martin, J. Potgieter, N. M. Kirby, A. Hawley, S. T. Mudie, T. M. Ryan, R. G. Haverkamp and M. H. Cumming, Materialia, 2018, 3, 90–96 CrossRef.
- L. Tang, S. Chen, W. Su, W. Weng, K. Osako and M. Tanaka, Process Biochem., 2015, 50, 148–155 CrossRef CAS.
- Z. Tian, K. Wu, W. Liu, L. Shen and G. Li, Spectrochim. Acta, Part A, 2015, 140, 356–363 CrossRef CAS PubMed.
- C. Ding, M. Zhang, K. Wu and G. Li, Polymer, 2014, 55, 5751–5759 CrossRef CAS.
- M. J. Fabra, A. López-Rubio and J. M. Lagaron, Food Hydrocolloids, 2016, 55, 11–18 CrossRef CAS.
- M. C. P. Gonçalves, T. G. Kieckbusch, R. F. Perna, J. T. Fujimoto, S. A. V. Morales and J. P. Romanelli, Process Biochem., 2019, 76, 95–110 CrossRef.
- F. Rehm, S. Chen and B. Rehm, Molecules, 2016, 21, 1370 CrossRef PubMed.
- F. Yasar Mahlicli, Y. Şen, M. Mutlu and S. Alsoy Altinkaya, J. Membr. Sci., 2015, 479, 175–189 CrossRef CAS.
- M. Ahlers, N. Stein, L. Broch and I. Brand, J. Electroanal. Chem., 2013, 706, 140–148 CrossRef CAS.
- P. L. Chandran, D. C. Paik and J. W. Holmes, Connect. Tissue Res., 2012, 53, 285–297 CrossRef CAS PubMed.
Footnote |
† These authors contributed equally to the work. |
|
This journal is © The Royal Society of Chemistry 2020 |
Click here to see how this site uses Cookies. View our privacy policy here.