DOI:
10.1039/C9RA10290F
(Review Article)
RSC Adv., 2020,
10, 10826-10847
Synthesis and application of coumarin fluorescence probes
Received
9th December 2019
, Accepted 15th February 2020
First published on 17th March 2020
Abstract
In recent years, the research on fluorescent probes has developed rapidly. Coumarin fluorescent probes have also been one of the hot topics in recent years. For the synthesis and application of coumarin fluorescent probes, great progress has been made. Coumarin fluorescent probes have become more and more widely used in biochemistry, environmental protection, and disease prevention, and have broad prospects. This review introduces the three main light emitting mechanisms (PET, ICT, FRET) of fluorescent probes, and enumerates some probes based on this light emitting mechanism. In terms of the synthesis of coumarin fluorescent probes, the existing substituents on the core of coumarin compounds were modified. Based on the positions of the modified substituents, some of the fluorescent probes reported in the past ten years are listed. Most of the fluorescent probes are formed by modifying the 3 and 7 position substituents on the mother nucleus, and the 4 and 8 position substituents are relatively less modified. In terms of probe applications, the detection and application of coumarin fluorescent probes for Cu2+, Hg2+, Mg2+, Zn2+, pH, environmental polarity, and active oxygen and sulfide in the past ten years are mainly introduced.
1. Introduction
In contrast to the general instrumental methods, fluorescence analyses have many outstanding features under physiological conditions, such as high sensitivity1,2 or selectivity,2,3 simple manipulations,4,5 low cost,5 rapid response rate,6 real-time detection,7 spatiotemporal resolution8 and facile visualization.9,10 In the past decade, a wide variety of fluorescent probes with different recognition moieties have been designed, which were based on the most versatile fluorophores, including fluorescein,11 coumarin, cyanine,12 boron-dipyrromethene (BODIPY),13 rhodamine, and 1,8-naphthalimide.14–17 They are usually used to detect various analytes. Therefore, the current study on fluorescence probes is a hot topic.
Coumarin, also known as benzopyran-2-one, is widely found in nature. It belongs to the flavonoid class of plant secondary metabolites and has a variety of biological activities, usually associated with low toxicity.18,19 Coumarin is widely distributed in different parts of plants and has the highest concentration in fruits, seeds, roots and leaves.20–22 It was first isolated from Tonka beans in 1820 and the first synthesis was reported in 1882.23 Various coumarin derivatives synthesized by modifying the structure of benzopyran ring show different pharmacological activities and they are widely used in medicine field. Coumarin derivatives can be used as fluorescent probes for metal ions due to their highly variable size, hydrophobicity, and chelation. The use of coumarin as a fluorescent probe began in the 19th century. Coumarin fluorescent probes have been widely used in many fields such as environmental protection and medicine. For example, Jun-Wu Chen et al. synthesized a fluorescent probe 1 (Fig. 1) composing coumarin as a fluorophore and a vinyl ether group as recognition unit to detect the concentration of Hg2+ in water. This probe reacts with Hg2+ to produce a strong fluorescence even the concentration of Hg2+ is lower than 2 ppm (Hg2+ concentration limits for drinking water set by the U.S. Environmental Protection Agency).24 So that, it can be used to monitor water pollutions.25 In medicine, coumarin fluorescent probes can be used for intracellular biological imaging, which can then be used to detect some cancers. To date, medical imaging has made great advances in locating and discriminating tumor lesions.26 Hong Y. Song synthesized the coumarin fluorescent probe 2 (Fig. 2), to label a bioactive small peptide bearing a cysteine sulfhydryl group. The arginine–glycine–aspartic acid (Arg–Gly–Asp; RGD) ligand has a high affinity with the integrin avb3 receptor which is up-regulated on some tumor cell membranes and plays an important role in metastasis.27 This peptide has also been radio-labelled for PET imaging of tumors.28
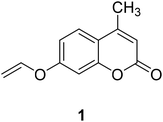 |
| Fig. 1 The structure of compound 1. | |
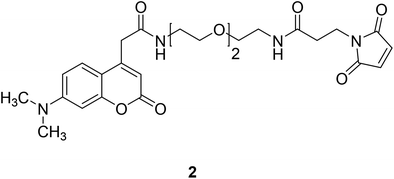 |
| Fig. 2 The structure of compound 2. | |
At present, the luminescence mechanism of most coumarin fluorescent probes can be divided into PET,29 ICT,30,31 FRET.32
2. Luminescence mechanism of coumarin fluorescent probes
2.1 PET mechanism (Fig. 7)
A typical PET system is constructed by attaching the recognition group R (receptor), which contains the electron donor, to a F (fluorophore) through S (spacer) (Fig. 3).33
 |
| Fig. 3 The “fluorophore–spacer–receptor” format of fluorescent PET.33 (Picture from ref. 33). | |
Currently, most PET fluorescent probes have been reported to use fat amino or azacrown ether as the identification group, like structure 334 (Fig. 4) and structure 435 (Fig. 5).
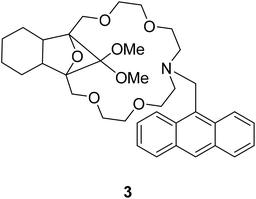 |
| Fig. 4 The structure of compound 3. | |
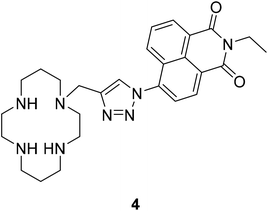 |
| Fig. 5 The structure of compound 4. | |
Most PET fluorescent probes are designed to bind the receptor to the object, inhibiting the light-induced electron transfer and causing the fluorophore to emit intense fluorescence (Fig. 6a). However, when interacting with the transition metal, the electrons can be transferred from the fluorophore to the transition metal or from the transition metal to the fluorophore due to the Redox behavior of the 3d electrons of the transition metal, resulting in fluorescence quenching by no radiation energy transfer (Fig. 6b).36
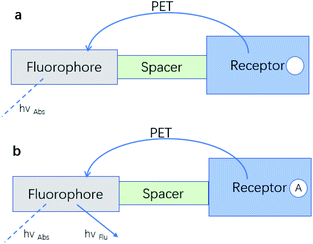 |
| Fig. 6 (a) An electron transfer from the analyte-free receptor to the photo-excited fluorophore creates the “off” state of the sensor. (b) The electron transfer from the analyte-bound receptor is blocked resulting in the “on” state of the sensor.33 (Picture from ref. 33). | |
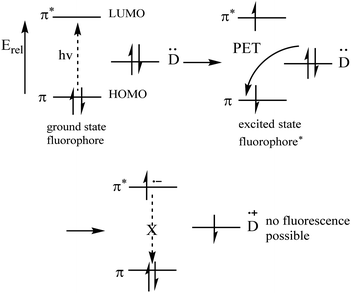 |
| Fig. 7 Molecular orbital energy diagrams37 (Picture from ref. 37). | |
Styliani Voutsadaki et al. synthesized a turn on PET fluorescent sensor 5 (Fig. 8) selective for Hg2+ ions in aqueous in 2010.38 Electrons of this probe are transferred from crown ether to the fluorophore and the fluorescence is attenuated.39 This process is cancelled when it bonds with metal ion, and the fluorescence intensity increases with the increase of metal concentration. This probe is potential to detect and quantify mercury in environmental and biological samples.
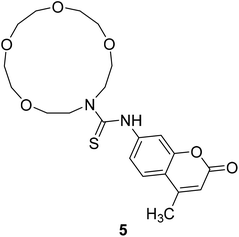 |
| Fig. 8 The structure of compound 5. | |
Yu Xu et al. synthesized compound 6 (Fig. 9) which synthetic mechanism is PET in 2014.40 This probe responds to pH by fluorescence quenching at 460 nm in the fluorescence spectrum, or by the ratio of maximum absorbance at 380 nm to 450 nm in the UV-visible spectrum, showing high sensitivity. In addition, probe 6 can monitor pH changes in real time because its fluorescence intensity is reversible between pH 1 and pH 7. Furthermore, this probe could be an ideal pH indicator for strongly acidic conditions with good biological significance.
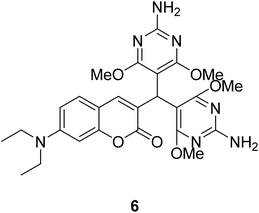 |
| Fig. 9 The structure of compound 6. | |
Long Yi et al. reported a thiol probe 7 (Fig. 10) based on the photoinduced electron transfer (PET) effect in 2009.41 The reaction of compound 7 with mercaptan produces blue-green emission visible to the naked eye. Thus, compound 7 can be used as a quantitative thiol probe for potential biological applications.
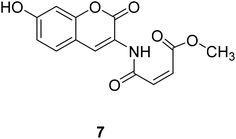 |
| Fig. 10 The structure of compound 7. | |
Kyung-Sik Lee et al. reported a coumarin-based fluorescent probe 8 (Fig. 11) in which the nitrogen's lone pair of electrons of the ring thiazolidine and ortho-hydroxy group form a hydrogen bond, preventing the quenching of PET and showing high selectivity to Hcy and Cys.42
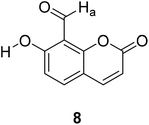 |
| Fig. 11 The structure of compound 8. | |
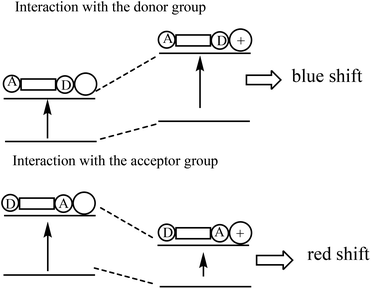 |
| Fig. 12 Intramolecular conjugate charge transfer principle43 (Picture from ref. 43). | |
2.2 ICT mechanism (Fig. 12)
The ICT fluorescent molecular probe is composed of a fluorophore, a strongly attracted electron base and a strongly pushed electron base, conjugated to form a strong push–pull electron system. Under photoexcitation, it will be generated the charge transfer from the electron donor to the electron acceptor. The combination of the identification group of the ICT fluorescence probe with the object affects the push–pull electron effect of the fluorophore, weakens or strengthens the intramolecular charge transfer, and thus leads to changes of the fluorescence spectrum, such as blue shift or red shift.43
Compound 9 (Fig. 13) reacts with H2S, exhibits an increase absorbance at 405 nm. The interaction increased the push–pull character and resulted the large bathochromic shifts in the absorption and the ICT effect.44
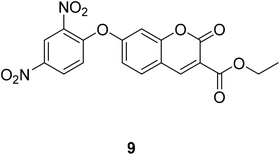 |
| Fig. 13 The structure of compound 9. | |
Jun Li et al. designed and synthesized the plasma probe 10 (Fig. 14) based on the intramolecular charge transfer (ICT) mechanism for the detection of bio thiols in aqueous solution in 2013.45 The probe 10 itself has weak fluorescence, but shows strong fluorescence when combined with R2SH.
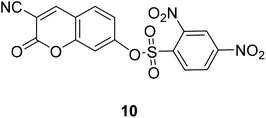 |
| Fig. 14 The structure of compound 10. | |
2.3 FRET mechanism
The fluorescent probe which based on FRET mechanism can join two fluorophores (donor and acceptor) in one molecule.36 Donor (D) collect radiation at the excitation wavelength and transfer this energy to the acceptor (A), which emits it at a longer wavelength. When the emission spectrum of the donor overlaps the absorption spectrum of the acceptor, it may occur non-radiative energy transfer from D to A. When D is excited, we can observe the fluorescence emission of A owing to energy transfer.
A. E. Albers et al. synthesized a fluorescent probe 11 (Fig. 15) for quantitative detection of endogenous H2O2 in 2006.46 The spectral overlap between luciferin absorption and coumarin release of 11 was very small, and the fluorescence energy transfer (FRET) was inhibited, so only blue donor emission was observed. When 11 interacts with H2O2, the spectral overlap increases, and the green fluorescein receptor is increased by FRET emission, excitation at 420 nm produces a bright green-colored fluorescence, so changes in [H2O2] can be detected by measuring the ratio of blue to green fluorescence intensity.
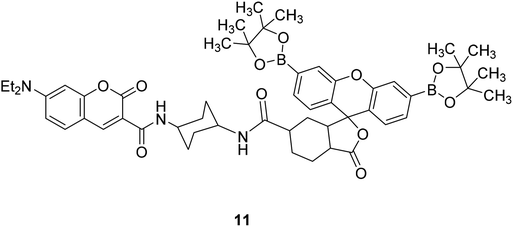 |
| Fig. 15 The structure of compound 11. | |
Changyu Zhang synthesized a coumarin fluorescent probe 12 (Fig. 16) based on the FRET mechanism in 2015 for the detection of H2S with high sensitivity.47
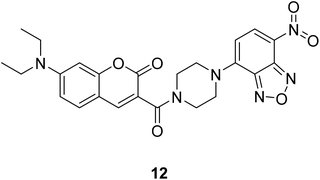 |
| Fig. 16 The structure of compound 12. | |
Fluorescence analysis has many outstanding characteristics under physiological conditions, such as high sensitivity, strong selectivity, simple operation, low cost, fast response speed, real-time detection, high spatiotemporal resolution, and convenient visualization. Currently, more fluorescent probes are reported. The light-emitting mechanism is based on PET, ICT, and FRET mechanisms. These three mechanisms all have certain disadvantages, such as low sensitivity, poor selectivity, and high environmental requirements required for the probe to function. Compared with the other two, the design of the ratio probe using the fluorescence resonance energy transfer (FRET) mechanism has many advantages, which can reduce the fluorescence detection error and self-quenching.
3. Synthesis of coumarin fluorescent probes
The synthesis of coumarin fluorescent probes is a popular topic in recent years. Their synthesis is partly based on classical methodologies such as Pechmann reaction or Knoevenagel condensation, but it also sparked the discovery of completely new pathways.48 The synthesis of coumarin fluorescent probe is based on the existing coumarin for the derivation, by changing the substituents to make the derivative have fluorescence characteristics, thus become a fluorescent probe in some aspects. Based on the classification of substituent position on the parent nucleus of coumarin, this paper introduced the synthesis methods of some fluorescent probes reported in this decade. Of course, it mainly introduced the synthesis of coumarin fluorescent probes mainly based on the three most common fluorescent luminescence mechanisms (PET, ICT and FRET) (Fig. 17).
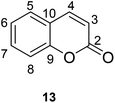 |
| Fig. 17 The chemical structure and numbering scheme of coumarin. | |
3.1 Classification by substituent position
3.1.1 Modification of the 3-position substituent of coumarin. Yu Xu et al. modified the 7-substituent of compound 14 to synthesize compound 6 which can be used to detect ambient pH in 2014 (Fig. 18).40
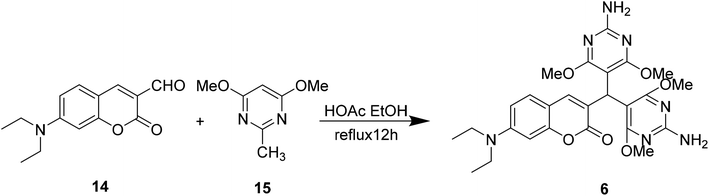 |
| Fig. 18 The synthetic route of compound 6. | |
Xiao-Fan Zhang et al. modified the substituent at the 3-position of compound 16 to develop a coumarin–rhodamine fluorescent probe 18 as a pH probe in 2013 (Fig. 19).49
 |
| Fig. 19 The synthetic route of compound 18. | |
Da En modified the 3-position substituent of compound 19 and designed a fluorescent probe 21 with high sensitivity and selectivity for Fe3+ ions in cells in 2014 (Fig. 20).50
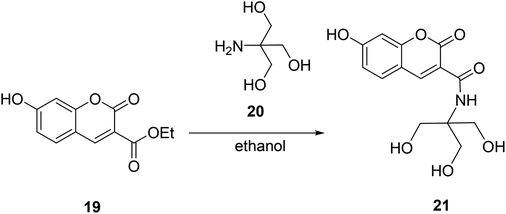 |
| Fig. 20 The synthetic route of compound 21. | |
Ying-Che Chen modified the substituent at position 3 of compound 22 to form a fluorescent probe 24. The fluorescent probe 24 binds to a target protein labeled with a short peptide sequence containing two Cys residues, which causes fluorescence quenching (Fig. 21).51
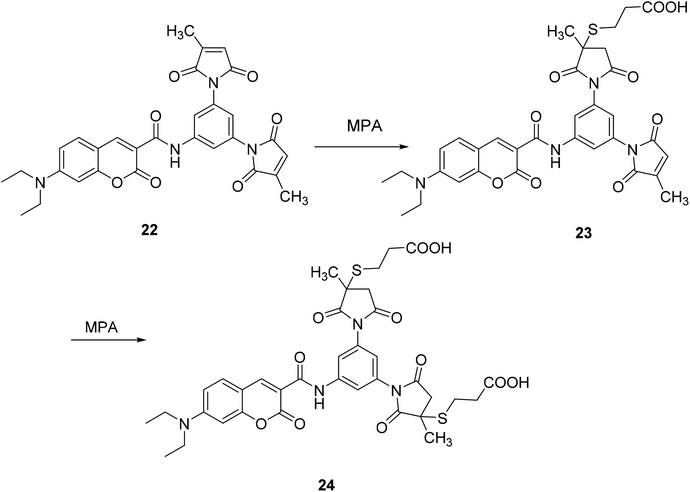 |
| Fig. 21 The synthetic route of compound 24. | |
Qi-Hua You modified the 3-position substituent of the compound 25 and designed a fluorescent probe 27 for detecting copper ions with good selectivity and sensitivity in 2014 (Fig. 22).52
 |
| Fig. 22 The synthetic route of compound 27. | |
3.1.2 Modification of the 4-position substituent of coumarin. Kai-Bo Zheng et al. developed a fluorescent probe 29 in 2014 which contains heavy atomic palladium, and should not display fluorescence, but interacts with CO to induce the release of palladium and restore fluorescence, showing a new emission band at 477 nm. Compound 29 has a higher sensitivity and an 11-fold increase in signal strength compared to current CO probes. The detection limit is 6.53 × 10−7 M (Fig. 23).53
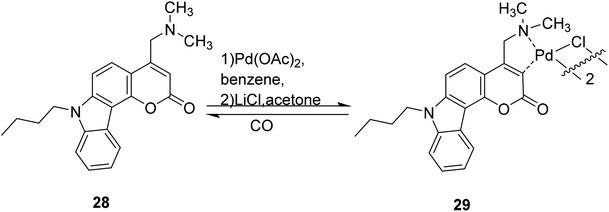 |
| Fig. 23 The synthetic route of compound 29. | |
3.1.3 Modification of the 7-position substituent of coumarin. Styliani Voutsadaki et al. modified the 3-position substituent of compound 31 to synthesized a “turn on” fluorescent sensor 5 selective for Hg2+ ions in aqueous in 2010 (Fig. 24).38
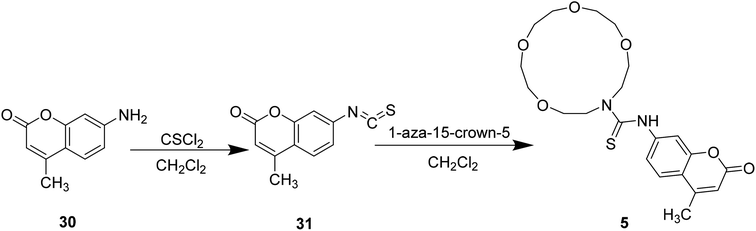 |
| Fig. 24 Synthesis of compound 5. | |
Xiao-Wei Cao et al. designed compound 35 to detect F– by modifying the 7-position substituent of compound 34 in 2011 (Fig. 25).54
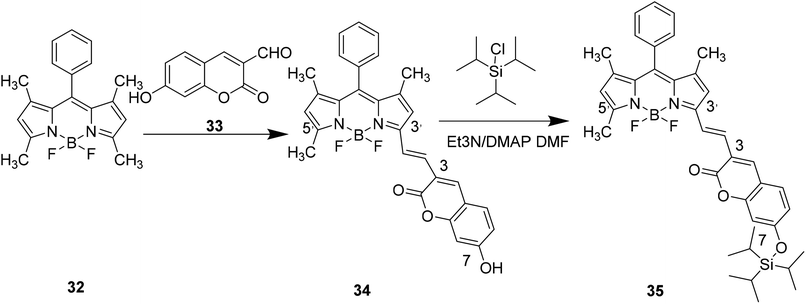 |
| Fig. 25 Synthesis of compound 35. | |
Danbi Jung, in 2014, modified a 7-position substituent on compound 36 to synthesize a fluorescent probe 38, which can be used to monitor thiol levels in cancer cells by fluorescence imaging, which is practical (Fig. 26).55
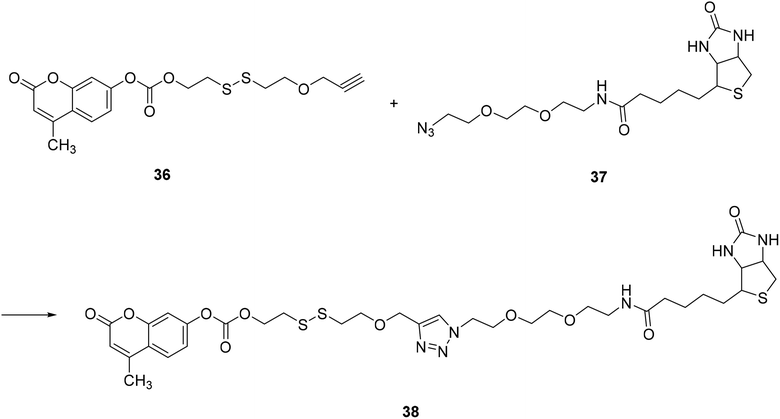 |
| Fig. 26 Synthesis of compound 38. | |
4. Application of coumarin fluorescent probe
Fluorescence sensing probes have many outstanding features that can detect a variety of analytes, such as real-time detection, high selectivity, and high throughput.57 Based on these characteristics, the research on fluorescent probes is also continuously progressing, and the research on coumarin fluorescent probes has also made certain progress. At present, the application range of coumarin fluorescent probes is more extensive. It has been reported that synthetic coumarin fluorescent probes can be used for the detection of some metal ions, the detection of environmental polarity, and the detection of active small molecules related to certain diseases of the human body. Therefore, it has applications in the fields of biochemistry, environmental protection and disease prevention.
4.1 Application in metal ion detection
Metal ion fluorescent probes have important applications in many fields such as environmental protection, biomedicine, chemistry, etc. In terms of environmental protection, heavy metal ion pollution has become increasingly serious in recent years, so it is particularly important to detect the concentration and content of heavy metal ions in the environment effectively, simply and quickly. Therefore, the development of a heavy metal ion fluorescence probe for indicating environmental pollution has great research value. In biomedical, since many metal ions take part in some important biochemical reactions in the body, or participate in the formation of coenzymes,58 as signal molecules to affect normal physiological functions, the form of some metal ions concentration in the body can reflect some chemical reaction process, and as a basis for the disease diagnosis, and indicated the development process of the disease. Based on these important functions, the study of metal ion fluorescence probe is of great value. Coumarin derivatives can be used as fluorescent probes for metal ions because of their highly variable size, hydrophobicity, and chelation.59
4.1.1 Cu2+ fluorescence detection. Copper are trace elements needed in the body, which assist many kinds of metalloenzymes to play a role. In humans, copper is essential to the proper functioning of organs and metabolic processes. It is a constituent of many enzyme systems like oxidases and hydroxylases.58Maity and his group synthesized two fluorescence probes (41 and 42) (Fig. 28) to detect selectively Cu2+ ions in aqueous buffer medium in 2013.59 Selected 480 nm as the excitation wavelength, the ring of rhodamine–thiolactam was partially opened with the addition of Cu2+, the yellow colored probe 41 changed to bright red upon Cu2+, probe 42 showed strong orange fluorescence at 590 nm.
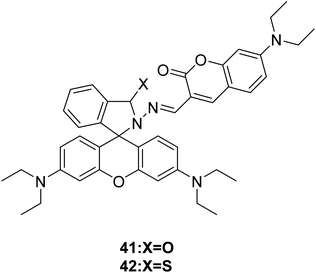 |
| Fig. 28 The structure of compound 41 and 42. | |
Jiun-Ting Yeh et al. synthesized a new coumarin-derived fluorescent probe 43 (Fig. 29) exhibited significant fluorescence quenching in the presence of Cu2+ ions in 2014.60 The maximum fluorescence quenching occurred over a pH range of 5–9. This coumarin-based Cu2+ chemosensor serves as an effective and non-destructive probe for Cu2+ detection in living cells. And it also can be used in the area of environmental protection and food safety.
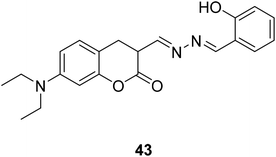 |
| Fig. 29 The structure of compound 43. | |
Hyo Sung Jung et al. developed a novel fluorogenic probe 44 (Fig. 30) bearing the 2-picolyl unit with high selectivity and suitable affinity toward Cu2+ in biological systems in 2009.61 The receptor can monitor Cu2+ ion in aqueous solution with a pH span 4–10. The compound 44 can be used for the fluorescence microscopic imaging and the study on the biological functions of Cu2+. Ahmadreza Bekhradnia et al. synthesized a novel fluorescent chemo sensor: coumarin carboxamide 45 (Fig. 31), through microwave irradiation in 2016.62 The compound can be used as fluorescent probe for Cu2+ with selectivity over other metal ions in aqueous solution and exhibit enhanced fluorescence. Rapid detection of Cu2+ ions in water plays an important role in improving environmental pollution.63
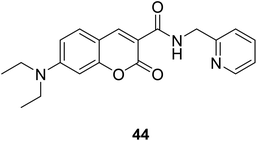 |
| Fig. 30 The structure of compound 44. | |
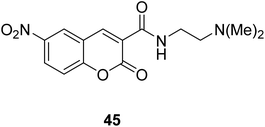 |
| Fig. 31 The structure of compound 45. | |
Qi-Hua You et al. synthesised a coumarin-based fluorescent chemo sensor 46 (Fig. 32) in 2014.52 It can recognize Cu2+ in aqueous acetonitrile solutions with high selectivity and sensitivity. Using the Cu-containing complex 46–Cu2+ as a sensing ensemble, highly selective recognize His/bio thiols. It also can be applied in fluorescence imaging of histidine in hard-to-transfect living cells. Due to its intrinsic paramagnetic properties, Cu2+ can quench the fluorescence of fluorescent metal chelators to make ensemble devices in nonfluorescence off state.64 Then, effectively snatch Cu2+ ions through Cu2+-binding analyte from the ensemble in aqueous solution can switch on the “turn-on” fluorescence of the sensing ensemble.65,66
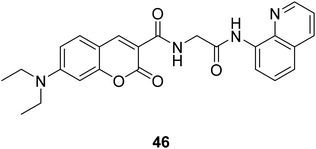 |
| Fig. 32 The structure of compound 46. | |
4.1.2 Hg2+ fluorescence detection. Hg2+ is a kind of heavy metal ion, can cause serious pollutions to the global environment. Hg2+ is a caustic and carcinogenic material with high cellular toxicity.67 It easily change to the highly toxic methyl mercury, passes through biological membranes resulting in the human's neurological system damage, DNA damage, various cognitive and motion disorders,68 and it can cause brain damage and other chronic diseases.69 Therefore, research a method to monitor Hg2+ in many scientific fields, including medicine, environmental and the like, has significant meaning.70 At present, there have been a lot of researches on mercury sensors. It is undeniable that the construction of mercury sensors can contribute to the preparation of other metal sensor. However, it must be admitted that its current application is very limited. Therefore, the breakthrough in the application of mercury sensor in the future is still the direction of efforts.Chen-Jun Wu et al. synthesized a novel probe 1 in 2017 (ref. 25) which treated with Hg2+ in HEPES buffer solution shows remarkable fluorescence enhancement,71–73 we can easily observe strong blue fluorescence by naked eyes. This method is sensitive and selective, so that it reveals the probe 1 could be used as a convenient tool to monitor Hg2+ in neat aqueous solution by fluorescence turn-on response. On the basis of the signal-to-noise ratio of three, the detection limit of this Hg2+ probe is determined as 0.12 μM (24 ppb). It is lower than the majority of reported probes.73–77 (According to the US EPA requirement, the limit of Hg2+ concentration for sake drinking water was set as 2 ppm). That means the probe 1 is sensitive enough to distinguish the Hg2+ for water quality detection.25
Qiu-juan Ma et al. synthesized a rhodamine–coumarin conjugate fluorescent probe 47 (Fig. 33) which with a sulfur-based functional group to detect Hg2+ in 2010.78 In 50% water/ethanol buffered at pH 7.24, the probe 47 binds excess Hg2+ and sensing Hg2+ sensitively and selectively. Furthermore, because of the chelation-induced ring opening of rhodamine spiro lactam, probe 47 also showed a reversible dual chromo- and fluorogenic response toward Hg2+. The development of Hg2+ ion fluorescence probes can be used to detect heavy metal pollution in the environment. It can be used to test the concentration of Hg2+ in both tap and river water samples.
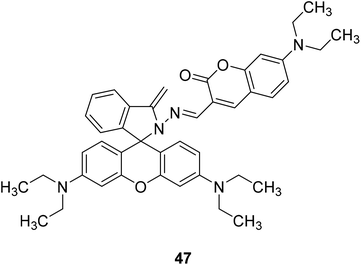 |
| Fig. 33 The structure of compound 47. | |
Wei-Min Xuan and his groups reported a ratio-metric fluorescent probe 48 (Fig. 34) in 2012.79 This probe can be used for living cell imaging and cell permeable. Even the concentration of Hg2+ was low as 2 × 10−8 M (close to the maximum contamination level set by the EPA), we can also observed pronounced fluorescent change.80
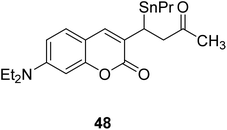 |
| Fig. 34 The structure of compound 48. | |
4.1.3 Mg2+ fluorescence detection. Mg2+ is the most abundant divalent cation within cells and it plays an important physiological role in bone remodelling and skeletal development.81 Magnesium deficiency may be related to the occurrence of many diseases, such as diabetes, osteoporosis, hypertension and coronary heart disease.82,83Vinod Kumar Gupta et al. designed compound 49 (Fig. 35) in 2017 to detect Mg2+ in the presence of alkali and alkaline earth metal ions. It showed a significant fluorescence enhancement towards Mg2+.84 This probe has a low detection limit for Mg2+. This probe is most widely used in Serum magnesium and the magnesium tolerance test. The fluorescence changes from weak blue to bright blue, which can be easily detected by the naked eye.82,84
Debdas Ray et al. designed a coumarin fluorescence sensors 50 (Fig. 36).85 Which was designed to show a significant red fluorescence enhancement response to Mg2+.
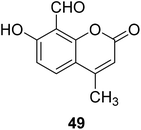 |
| Fig. 35 The structure of compound 49. | |
Hai-Jing Yin and his groups reported two novel 7-substituted coumarin-based two-photon fluorescent probes (51, 52) (Fig. 37) for biological Mg2+ detection in 2015. The probe has higher sensitivity, lower detection limit, and interacts with lower concentration of magnesium ions, showing enhanced fluorescence. The experiment found that these probes are not sensitive in the biologically relevant pH range and have low cytotoxicity, so we can apply them to physiological studies.86,87
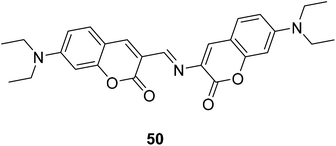 |
| Fig. 36 The structure of compound 50. | |
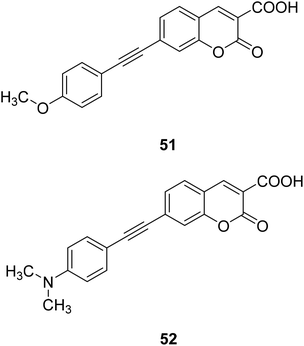 |
| Fig. 37 The structure of compound 51, 52. | |
4.1.4 Zn2+ fluorescence detection. Zn2+ ions are involved in building enzymes and proteins and involved in a variety of biochemical reactions like gene transcription, regulation of metalloenzymes, neural signal transmission, superoxide dismutase, cytochrome oxidase.88–90 Minute amounts of zinc help keep the body healthy, but too much can lead to bad results and disease. So as to keep health, it is really important to design several convenient tools to inspect the concentration of Zn2+.Jing-can Qin et al. synthesized a simple two-photon excitation (TPE) probe 53 (Fig. 38) for Zn2+ in 2016.91 Two-photon excitation (TPE) has the advantages of reducing background fluorescence, increasing tissue penetration, and reducing light damage to biological samples, etc.92–94 The probe selectively binds to zinc ions, showing a significant increase in fluorescence at 500 nm. In addition, due to its low detection limit, the sensor should be able to find potential applications in detecting trace Zn2+ concentrations in biological systems and environments.91
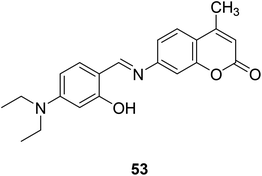 |
| Fig. 38 The structure of compound 53. | |
Shin Mizukami et al. designed a ratio-metric Zn2+ probe (54) (Fig. 39) in 2009.95 The probe 54 could permeate living cell membranes, we introduced it to living RAW264 cells to observe the intracellular Zn2+ concentration via ratio metric fluorescence microscopy. When the probe is bound to zinc ions, the absorption spectrum shifted toward longer wavelengths, and the fluorescence is enhanced.
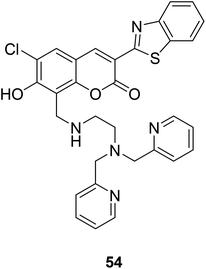 |
| Fig. 39 The structure of compound 54. | |
4.2 Application in PH detection
Intracellular pH plays an important role in drug resistance, cell proliferation, invasion and metastasis, apoptosis, disease and other processes.96 In addition, changes in intracellular pH have been linked to diseases such as some cancer and Alzheimer's disease.
The fluctuation of pH has obvious effect on numerous cellular events, such as cellular metabolism,97 cellular growth,98 signal transduction,99 chemotaxis, apoptosis,100 and autophagy.101 Therefore, monitoring pH changes inside living cells is crucial for exploring cellular functions and understanding physiological and pathological processes in organisms.
Sa-Sa Zhu et al. designed a new linked coumarin–quinoline ratio metric pH probe 55 (Fig. 40) which can be used in cellular imaging studies in 2013.102 When the pH is from 7.4–2.9, the absorption peak of the probe near 425 nm is significantly reduced. At the same time, a new red-shifted absorption band was formed near 516 nm. As the pH decreased, the absorption spectrum gradually red-shifted. The characters of the novel probe include strong fluorescence under acidic conditions, low cytotoxicity and good cell membrane permeability; these features make the probe useful for monitoring pH variations from neutral to acidic conditions in living cells.
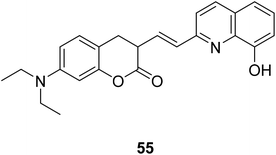 |
| Fig. 40 The structure of compound 55. | |
Yu Xu et al. synthesised a new turn-off fluorescent probe 6 (Fig. 9) with coumarin and imidazole moiety in 2014.40 This probe could be a practical and ideal pH indicator and play a role in extremely acidic environments. When the pH is low (below 3.5), the fluorescence at 460 nm is quenched. A considerable number of microorganisms like Helicobacter pylori and “acidophiles” could survive in harsh acidic environment.103 So that this probe can be used to imaging strong acidity in bacteria. Moreover, in some eukaryotic cells, acidic pH affects organelles along the secretory and endocytic pathways.104,105 Moreover, the fluorescence intensity of probe 6 is reversible between pH 1 and pH 7, which allows it to monitor a system with a shifty pH value and report the real time acidity. The probe is reversible, great selective and quickly responsive. We believe it will be beneficial to study in chemical and biological systems.
Xiao-Fan Zhang developed a coumarin–rhodamine probe 18 (Fig. 41) as a ratio metric pH probe in 2017.49 When the probe interacted with H+, the coumarin release decreased at 477 nm and the rhodamine release increased at 582 nm. The fluorescence intensity ratio responded linearly to minor pH changes in the range of 4.20–6.00. The probe showed high selectivity among different amino acids, metal cations and the ATP. Moreover, it has been successfully applied in fluorescence imaging in HeLa cells and the results indicated that the probe could selectively stain lysosome with low cytotoxicity and excellent photostability. We also applied 18 to monitor intracellular pH variations induced by dexamethasone. Therefore, 18 could act as a practical tool for the detection of pH in weakly acidic conditions and provide essential information in medicinal analysis and real biological systems.
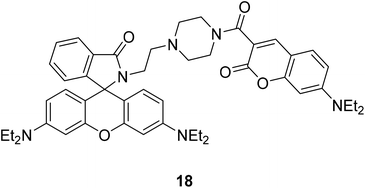 |
| Fig. 41 The structure of compound 18. | |
4.3 Application in the detection of reactive O/S
4.3.1 Fluorescence detection of reactive oxygen. Reactive oxygen species (ROS) are involved in a variety of pathological diseases, played a significant role in keeping body health.106 Hydroxyl radicals, is one of the most important ROS, and it can damage DNA, proteins, or membrane lipids and cause many diseases such as inflammations, embryo teratogenesis, herbicide effects, cell death, and killing of micro-organisms in pathogen-defence reactions.107 Thus, developed new methods for detecting hydroxyl radicals in living cells have great meanings.Lin Yuan et al. synthesized a new probe 56 (Fig. 42) which are really stable against auto-oxidation. The probe 56 as the first probe to achieve ratio metric fluorescent imaging of intracellular hydroxyl radicals. When interacting with hydroxyl radicals, the fluorescence intensity ratio of probe 56 at 495 and 651 nm (I651/I495) increased significantly.108
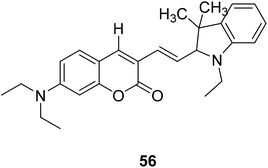 |
| Fig. 42 The structure of compound 56. | |
Ji-Ting Hou et al. firstly designed a ratio-metric fluorescent probe 57 (Fig. 43) for ClO− in 2015, which can sense ClO− quickly and sensitively.109 The probe reacted to ClO− under alkaline conditions, and a new emission appeared at 580 nm. The emission intensity increased with the increase of hypochlorite dose, and the fluorescence intensity decreased at 470 nm. More importantly, 57 is the first mitochondria-targeted ratio-metric fluorescent probe to image exogenous and endogenous ClO−. ClO− produced by mitochondria has been linked to cancer. Therefore, a quantitative detection, especially in situ detection, of basal ClO− in cancer cells is of significant interest.
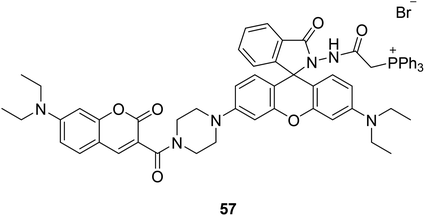 |
| Fig. 43 The structure of compound 57. | |
4.3.2 Fluorescence detection of active sulfur H2S. In vivo, H2S is produced in many organs and tissues, catalysed by enzymes.110 Hydrogen sulphide (H2S), as an endogenous gas compound, is involved in regulating a variety of physiological processes to maintain the health of the body. These physiological processes include regulation of inflammatory parts in the body,111 relaxation of vasodilation, protection of vascular system,112 influence insulin signal transmission,113 intervention of nerve signal transmission,114 anti-oxidation, and inhibit apoptosis of some cells in the body. H2S can also inhibit leukocyte adherence in mesenteric microcirculation during vascular inflammation in rats, suggesting H2S is a potent anti-inflammatory molecule. The concentration of H2S was changed depend on physiological and pathological states.115Zhang Changyu et al. designed a fluorescent probe 58 (Fig. 44) that interacts with H2S in 2015.44 It is reported that the probe has a large switching fluorescence response and can be used for biological imaging of endogenous H2S in living cells. The probe interacted with H2S and showed fluorescence quenching.
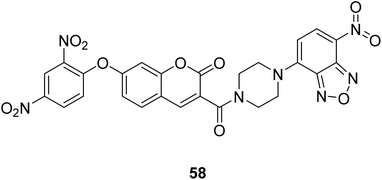 |
| Fig. 44 The structure of compound 58. | |
Bi-feng Chen and his team synthesized a coumarin fluorescent chemical probe 59 (Fig. 45) that detects H2S in fetal bovine serum and degassed PBS buffer, a method with high selectivity and sensitivity in 2013.116 The probe interacts with H2S to increase fluorescence. The probe can achieve in situ display of H2S in normal and AS rat heart tissues, and can also be used to screen agonists or antagonists of H2S synthase and tissue imaging. Therefore, this probe plays an important role in detecting H2S and maintaining body health.
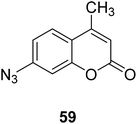 |
| Fig. 45 The structure of compound 59. | |
Ming-Yu Wu et al. synthesized a colorimetric fluorescent probe 60 (Fig. 46) in 2012.117 The probe can reduce nitro compounds to amines in the presence of H2S, so that it could be used to detect H2S. The probe interacts with H2S and generates a large emission displacement. The fluorescence probe provides a good method for the detection of hydrogen sulfide in the process of metabolism due to its fast response, high sensitivity and strong fluorescence ratio.
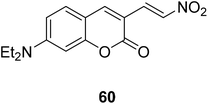 |
| Fig. 46 The structure of compound 60. | |
4.4 Microenvironment polarity detection applications
Marek Cigáň and his groups investigated four new efficient fluorescent “turn-on” probes 61, 62, 63, 64 (Fig. 47) to sensing water in 2016.118 All this coumarin fluorescence probes can response to low-level water content in polar aprotic solvents rapidly and reversibly, and the detection limits are amongst the lowest, even can compete in sensitivity with chemo dosimeters. Owing to that optical sensors for water sensing are flexibility, and it is possible to achieve remote monitoring, so that the probes for water sensing can be used in food processing, chemical reagents pharmaceutical manufacturing, and biomedical or environmental and the like. The specific performance is that when the probe meets with water in the polar aprotic solvents, we can see orange-yellow colour change and simultaneous fluorescence increase.
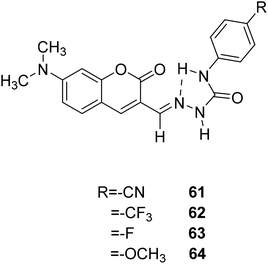 |
| Fig. 47 The structure of compound 61–64. | |
Giovanni Signore et al. synthesized coumarin fluorescence probes (65a–d, 66e–f) (Fig. 48) which can be detected only in the most lipophilic environments of the cell in 2010.119 The probe can be used as remarkable tools for studying subtle biochemical processes in the cellular environment when properly combined with biomolecules. Intracellular protein activity determines cellular behaviour, and the use of fluorescent probes and high-resolution imaging makes it possible to detect protein activity in the cellular environment. However, testing the activity of proteins in their completely natural state is not intrinsically feasible, and this problem can be solved by using a molecule that penetrates cells to selectively bind to a target protein in a certain state. These fluorescent molecules are extremely sensitive to environmental polarity and have good luminance in cells, which can be detected. It can be used as an indicator of environmental polarity change to reflect the biochemical process in cells. For example, coumarin without conjugate cyanide can be used as a fluorescent probe to detect the polarity of the environment. There is no fluorescence in water, but when the polarity of environment decreases, it shows strong fluorescence. Coumarin binds to protein and the same is true.
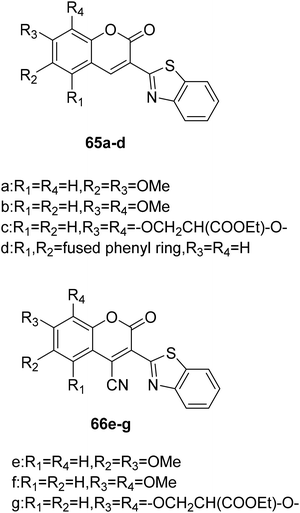 |
| Fig. 48 The structure of compound 65a–d, 66e–g. | |
5. Conclusions
This review has highlighted the various aspects of coumarin fluorescent probes, including their chemical synthesis and application. Coumarin derivatives have good applications as fluorescent probes in many fields, such as detecting metal ions, environmental polarity, some disease-related active small molecules in vivo, etc. Ji Yun Ting et al. found a new coumarin-derived fluorescent probe 43, which binds to Cu2+ ions and shows obvious fluorescence quenching within the pH range of 5–9. It has been applied in living cells and the field of environmental food safety. Coumarin fluorescent probes are widely used in pharmaceutical chemistry. This paper reviews the synthesis of coumarin fluorescent probes based on different mechanisms and their applications in biochemical field. The detection of some active oxygen sulfur compounds by coumarin fluorescent probes provides great help for the diagnosis and treatment of cancer, and the monitoring of some heavy metal ions provides help for environmental protection. In the synthetic method, the fluorescent probes partially synthesized in the past ten years are summarized based on the position of the modified substituents on the coumarin mother nucleus.
Although the synthesis of coumarin fluorescent probes has made great progress, the structural optimization and modification still need to be further studied, and the application of coumarin probes also needs further exploration. At present, fluorescent probes can cause damage to some tumor cells, but there are few studies on their damage to normal cells. In the future, further studies on the damage of coumarin fluorescent probes to normal cells are needed to continuously reduce their harmful damage. The sensitivity of fluorescent probes needs to be improved. NIR fluorescence imaging has the advantages of deep tissue penetration, low background fluorescence interference and minimal light damage of biological samples, which has attracted people's attention. Therefore, further research is needed to improve the fluorescent probe to near-infrared. Now, to design ratiometric probes with the mechanism of fluorescence resonance energy transfer (FRET) system has many advantages. It can reduce fluorescence detection error and self-quenching.120–122 It is still a problem worth studying to fabricate fluorescent chemo-sensors based on the FRET system. It is hoped that the ideas and examples cited in this review article will further stimulate and optimize the full potential of coumarin-based fluorescent probes, improve design selectivity, realize more simple and efficient applications in more fields, and help in the treatment of diseases.
Conflicts of interest
The authors declare that this article content has no conflict of interest.
Acknowledgements
The authors are grateful to the Shandong Provincial Academy of Medical Sciences for the scientific research project (2019–18), and the Shandong Natural Science Foundation [ZR2018LH021].
Notes and references
- X. Q. Li, J. P. Gu, Z. Zhou, L. F. Ma, Y. H. Zheng, Y. P. Tang, J. W. Gao and Q. M. Wang, Chem. Eng. J., 2019, 358, 67–73 CrossRef CAS.
- P. P. Deshmukh, A. Navalkar, S. K. Maji and S. T. Manjare, Sens. Actuators, B, 2018, 281, 8–13 CrossRef.
- X. H. Cheng, H. Z. Jia, T. Long, J. Feng, J. G. Qin and Z. Li, Chem. Commun., 2011, 47(43), 11978–11980 RSC.
- N. T. Wu, Y. P. Tang, M. Zeng, J. W. Gao, X. B. Lu and Y. H. Zheng, J. Lumin., 2018, 202, 502–507 CrossRef CAS.
- D. Lu, Y. P. Tang and Y. H. Zheng, J. Fluoresc., 2018, 28(6), 1269–1273 CrossRef CAS PubMed.
- X. Q. Li, Z. Zhou, C. C. Zhang, Y. H. Zheng, J. W. Gao and Q. M. Wang, Inorg. Chem., 2018, 57(15), 8866–8873 CrossRef CAS PubMed.
- N. Soh, Y. Katayama and M. Maeda, Analyst, 2001, 126(5), 564–566 RSC.
- K. Tanaka, T. Miura, N. Umezawa, Y. Urano, K. Kikuchi, T. Higuchi and T. Nagano, J. Am. Chem. Soc., 2001, 123(11), 2530–2536 CrossRef CAS PubMed.
- R. Su, J. W. Gao, S. R. Deng, R. H. Zhang and Y. H. Zheng, J. Sol-Gel Sci. Technol., 2016, 78(3), 606–612 CrossRef CAS.
- C. F. Yu, Z. Y. Zhang, M. Z. Fu, J. W. Gao and Y. H. Zheng, J. Electron. Mater., 2017, 46(10), 5895–5900 CrossRef CAS.
- S. L. Gui, Y. Y. Huang, F. Hu, Y. L. Jin, G. X. Zhang, L. S. Yan, D. Q. Zhang and R. Zhao, Anal. Chem., 2015, 87(3), 1470–1474 CrossRef CAS PubMed.
- Z. Zhou, C. C. Zhang, Y. H. Zheng and Q. M. Wang, Dyes Pigm., 2018, 150, 151–157 CrossRef CAS.
- B. L. Sui, S. M. Tang, T. H. Liu, B. S. Kim and K. D. Belfield, ACS Appl. Mater. Interfaces, 2014, 6(21), 18408–18412 CrossRef CAS PubMed.
- V. S. Lin, W. Chen, M. Xian and C. J. Chang, Chem. Soc. Rev., 2015, 44(14), 4596–4618 RSC.
- Y. H. Tang, D. Lee, J. L. Wang, G. H. Li, J. H. Yu, W. Y. Lin and J. Yoon, Chem. Soc. Rev., 2015, 44(15), 5003–5015 RSC.
- X. Q. Li, Z. Zhou, Y. P. Tang, C. C. Zhang, Y. H. Zheng, J. W. Gao and Q. M. Wang, Sens. Actuators, B, 2018, 276, 95–100 CrossRef CAS.
- J. P. Gu, X. Q. Li, Z. Zhou, R. S. Liao, J. W. Gao, Y. P. Tang and Q. M. Wang, Chem. Eng. J., 2019, 368, 157–164 CrossRef CAS.
- F. Borges, F. Roleira, N. Milhazes, L. Santana and E. Uriarte, Curr. Med. Chem., 2005, 12(30), 887–916 CrossRef CAS PubMed.
- M. F. M. R. Borges, F. M. F. Roleira, N. J. D. S. P. Milhazes, E. U. Villares and L. S. Penin, Front. Chem., 2009, 4(8), 23–85 Search PubMed.
- G. B. Bubols, D. R. Vianna, A. Medina-Remon, G. VonPoser, R. M. Lamuela-Raventos, V. L. Eifler-Lima and S. C. Garcia, Mini-Rev. Med. Chem., 2013, 13(3), 318–322 CAS.
- S. E. Bariamis, M. Marin, C. M. Athanassopoulos, C. Kontogiorgis, Z. Tsimal, D. Papaioannou, G. Sindona, G. Romeo, K. Avgoustakis and D. Hadjipavlou-Litina, Eur. J. Med. Chem., 2013, 60, 155–169 CrossRef CAS PubMed.
- M. J. Matos, L. Santana, E. Uriarte, G. Delogu, M. Corda, M. B. Fadda, B. Era and A. Fais, Bioorg. Med. Chem. Lett., 2011, 21(11), 3342–3348 CrossRef CAS PubMed.
- H. M. Revankar, S. N. A. Bukhari, G. B. Kumar and H. L. Qin, Bioorg. Chem., 2017, 71, 146–159 CrossRef CAS PubMed.
- Y. Jeong and J. Yoon, Inorg. Chim. Acta, 2012, 381, 2–14 CrossRef CAS.
- C. J. Wu, J. B. Wang, J. J. Shen, C. Bi and H. W. Zhou, Sens. Actuators, B, 2017, 243, 678–683 CrossRef CAS.
- H. Kobayashi, M. Ogawa, R. Alford, P. L. Choyke and Y. Urano, Chem. Rev., 2010, 110(5), 2620–2640 CrossRef CAS PubMed.
- E. Ruoslahti, Annu. Rev. Cell Dev. Biol., 1996, 12, 697–715 CrossRef CAS PubMed.
- H. Y. Song, M. H. Ngai, Z. Y. Song, P. A. MacAry, J. Hobley and M. J. Lear, Org. Biomol. Chem., 2009, 7(17), 3400–3406 RSC.
- I. Wallace, M. Davis, L. Munro, V. J. Catalano, P. J. Cragg, M. T. Huggins and K. J. Wallace, Org. Lett., 2012, 14(11), 2686–2689 CrossRef PubMed.
- Z. R. Grabowski, K. Rotkiewicz and W. Rettig, Chem. Rev., 2003, 103(10), 3899–4032 CrossRef PubMed.
- R. R. Hu, E. Lager, A. Aguilar, J. C. Liu, W. Jacky, H. H.-Y. Sung, L. D. Williama, Y. C. Zhong, K. S. Wong and B. Z. Tang, J. Phys. Chem. C, 2009, 113(36), 15845–15853 CrossRef CAS.
- H. Sahoo, J. Photochem. Photobiol., C, 2011, 12(1), 20–30 CrossRef CAS.
- A. P. Silva, T. S. Moody and G. D. Wright, Analyst, 2009, 134(12), 2385–2393 RSC.
- F. A. Khan, K. Parasuraman and K. K. Sadhu, Chem. Commun., 2009, 134(17), 2399–2401 RSC.
- E. Tamanini, A. Katewa, L. M. Sedger, M. H. Todd and M. Watkinson, Inorg. Chem., 2009, 48(1), 319–324 CrossRef CAS PubMed.
- N. Boens, V. Leen and W. Dehaen, Chem. Soc. Rev., 2012, 41(24), 1130–1172 RSC.
- Y. H. Fu and N. Finney, RSC Adv., 2018, 8(51), 29051–29061 RSC.
- S. Voutsadaki, G. K. Tsikalas, E. Klontzas and G. E. Froudakis, Chem. Commun., 2010, 46(19), 3292–3294 RSC.
- G. Shanker, L. A. Mutkus, S. J. Walker and M. Aschner, Mol. Brain Res., 2002, 106(1–2), 1–11 CrossRef CAS PubMed.
- Y. Xu, Z. Jiang, Y. Xiao, F. Z. Bi, J. Y. Miao and B. X. Zhao, Anal. Chim. Acta, 2014, 820, 146–151 CrossRef CAS PubMed.
- L. Yi, H. Y. Li, L. Sun, L. L. Liu, C. H. Zhang and Z. Xi, Angew. Chem., Int. Ed., 2009, 48(22), 4034–4037 CrossRef CAS PubMed.
- K. S. Lee, T. K. Kim, J. H. Lee, H. J. Kim and J. I. Hong, Chem. Commun., 2008, 14(46), 6173–6175 RSC.
- M. H. Lee, J. S. Kim and J. L. Sessler, Chem. Soc. Rev., 2015, 44(13), 4185–4191 RSC.
- C. Y. Zhang, L. Wei, C. Wei, J. Zhang, R. Wang, Z. Xi and L. Yi, Chem. Commun., 2015, 51(35), 7505–7508 RSC.
- J. Li, C. F. Zhang, Z. Z. Ming, W. C. Yang and G. F. Yang, RSC Adv., 2013, 3(48), 26059–26065 RSC.
- A. E. Albers, V. S. Okreglak and C. J. Chang, J. Am. Chem. Soc., 2006, 128(30), 9640–9641 CrossRef CAS PubMed.
- C. Y. Zhang, L. Wei, C. Wei, J. Zhang, R. Y. Wang, Z. Xi and L. Yi, Chem. Commun., 2015, 51(52), 10510–10513 RSC.
- M. Tasior, D. Kim, S. Singha, M. Krzeszewski, K. H. Ahn and D. T. Gryko, J. Mater. Chem. C, 2015, 3(7), 1421–1446 RSC.
- X. F. Zhang, T. Zhang, S. L. Shen, J. Y. Miao and B. X. Zhao, RSC Adv., 2013, 5(61), 49115–49121 RSC.
- D. En, Y. Guo, B. T. Chen, B. Dong and M. J. Peng, RSC Adv., 2014, 4(1), 248–253 RSC.
- Y. C. Chen, C. M. Clouthier, K. Tsao, M. Strmiskova, H. Lachance and J. W. Keillor, Angew. Chem., Int. Ed., 2014, 53(50), 1–5 Search PubMed.
- Q. H. You, A. W. M. Lee, W. H. Chan, X. M. Zhu and K. C. F. Leung, Chem. Commun., 2014, 50(47), 6207–6210 RSC.
- K. B. Zheng, W. Y. Lin, L. Tan, H. Chen and H. J. Cui, Chem. Sci., 2014, 5(9), 3439–3448 RSC.
- X. W. Cao, W. Y. Lin and Q. X. Yu, J. Org. Chem., 2011, 76(18), 7423–7430 CrossRef CAS PubMed.
- D. Jung, S. Maiti, J. H. Lee, J. H. Lee and J. S. Kim, Chem. Commun., 2014, 50(23), 3044–3047 RSC.
- M. H. Yan, T. R. Li and Z. Y. Yang, Inorg. Chem. Commun., 2011, 14, 463–465 CrossRef CAS.
- X. H. Fang, J. J. Li, J. Perlette, W. H. Tan and K. M. Wang, Anal. Chem., 2000, 72(23), 747A–753A CrossRef CAS PubMed.
- A. Bekhradnia, E. Domehri and M. Khosravi, Spectrochim. Acta, Part A, 2016, 152(5), 18–22 CrossRef CAS PubMed.
- D. Maity, D. Karthigeyan, T. K. Kundu and T. Govindaraju, Sens. Actuators, B, 2013, 176, 831–837 CrossRef CAS.
- J. T. Yeh, W. C. Chen, S. R. Liu and S. P. Wu, New J. Chem., 2014, 38(9), 4434–4439 RSC.
- H. S. Jung, P. S. Kwon, J. W. Lee, J. I. Kim, C. S. Hong, J. W. Kim, S. H. Yan, J. Y. Lee, J. H. Lee, T. Joo and J. S. Kim, J. Am. Chem. Soc., 2009, 131(5), 2008–2012 CrossRef CAS PubMed.
- A. Bekhradnia, E. Domehri and M. Khosravi, Spectrochim. Acta, Part A, 2016, 152, 18–22 CrossRef CAS PubMed.
- W. Y. Lin, L. Yuan, W. Tan, J. B. Feng and L. L. Long, Chem.–Eur. J., 2009, 15, 1030–1035 CrossRef CAS PubMed.
- A. Bekhradnia, E. Domehri and M. Khosravi, Spectrochim. Acta, Part A, 2016, 152, 18–22 CrossRef CAS PubMed.
- X. Chen, S. W. Nam, G. H. Kim, N. Song, Y. Jeong, I. Shin, S. K. Kim, J. Kim, S. Park and J. Yoon, Chem. Commun., 2010, 46(47), 8953–8955 RSC.
- J. T. Hou, K. Li, K. K. Yu, M. Y. Wu and X. Q. Yu, Org. Biomol. Chem., 2013, 11(5), 717–720 RSC.
- J. S. Lee, M. S. Han and C. A. Mirkin, Angew. Chem., Int. Ed., 2007, 46(22), 4093–4096 CrossRef CAS PubMed.
- E. M. Nolan and S. J. Lippard, Chem. Rev., 2008, 108(9), 3443–3480 CrossRef CAS PubMed.
- I. Onyido, A. R. Norris and E. Buncel, Chem. Rev., 2004, 104(12), 5911–5929 CrossRef CAS PubMed.
- X. Q. Chen, X. Z. Tian, I. Shin and J. Yoon, Chem. Soc. Rev., 2011, 40(9), 4783–4804 RSC.
- J. W. Hu, Z. J. Hu, S. Liu, Q. Zhang, H. W. Gao and K. Uvdal, Sens. Actuators, B, 2016, 230, 639–644 CrossRef CAS.
- J. Ding, H. Li, C. Wang, J. Yang, Y. Xie, Q. Peng, Q. Li and Z. Li, ACS Appl. Mater. Interfaces, 2015, 7(21), 11369–11376 CrossRef CAS PubMed.
- Y. Y. Yan, Y. H. Zhang and H. Xu, ChemPlusChem, 2013, 78(7), 628–631 CrossRef CAS PubMed.
- M. Hong, S. Lu, F. Lv and D. Xu, Dyes Pigm., 2016, 127, 94–99 CrossRef CAS.
- S. L. Kao and S. P. Wu, Sens. Actuators, B, 2015, 212, 382–388 CrossRef CAS.
- S. Erdemir, O. Kocyigit and S. Karakurt, Sens. Actuators, B, 2015, 220, 381–388 CrossRef CAS.
- Q. Zou, L. Zou and W. J. Wu, J. Mater. Chem., 2011, 21(38), 14441–14447 RSC.
- Q. J. Ma, X. B. Zhang, X. H. Zhao, Z. Jin, G. J. Mao, G. L. Shen and R. Q. Yu, Anal. Chim. Acta, 2010, 663(1), 85–90 CrossRef CAS PubMed.
- W. M. Xuan, C. Chen, Y. T. Cao, W. H. He, W. Jiang, K. J. Liu and W. Wang, Chem. Commun., 2012, 48(58), 7292–7294 RSC.
- F. J. Huo, Y. Q. Sun, J. Su, Y. T. Yang, C. X. Yin and J. B. Chao, Org. Lett., 2010, 12(21), 4756–4759 CrossRef CAS PubMed.
- R. Bogoroch and L. F. Belanger, Anat. Rec., 1975, 183(3), 437–447 CrossRef CAS PubMed.
- W. Jahnen-Dechent and M. Ketteler, Magnesium, Basics, Clin. Kidney J., 2012, 5(suppl 1), i3–i14 CrossRef CAS PubMed.
- R. Swaminathan, Clin. Biochem. Rev., 2003, 24(2), 47–66 CAS.
- V. K. Gupta, M. Naveen and L. K. Kumawat, Sens. Actuators, B, 2015, 207, 216–223 CrossRef CAS.
- D. Ray and P. K. Bharadwaj, Inorg. Chem., 2008, 47, 2252–2254 CrossRef CAS PubMed.
- H. J. Yin, B. C. Zhang, H. Z. Yu, L. Zhu, Y. Feng, M. Z. Zhu, Q. X. Guo and X. M. Meng, J. Org. Chem., 2015, 80(9), 4306–4312 CrossRef CAS PubMed.
- T. Fujii, Y. Shindo, K. Hotta, D. Citterio, S. Nishiyama, K. Suzuki and K. Oka, J. Am. Chem. Soc., 2014, 136(6), 2374–2381 CrossRef CAS PubMed.
- K. Li and A. J. Tong, Sens. Actuators, B, 2013, 184, 248–253 CrossRef CAS.
- R. McRae, P. Bagchi, S. Sumalekshmy and C. J. Fahrni, Chem. Rev., 2009, 109(10), 4780–4827 CrossRef CAS PubMed.
- L. J. Tang, M. J. Cai, P. Zhou, J. Zhao, K. L. Zhong, S. H. Hou and Y. J. Bian, RSC Adv., 2013, 3(37), 16802–16809 RSC.
- J. C. Qin, L. Fan and Z. Y. Yang, Sens. Actuators, B, 2016, 228, 156–161 CrossRef CAS.
- W. Zhang, P. Li, F. Yang, X. Hu, C. Sun, W. Zhang, D. Chen and B. Tang, J. Am. Chem. Soc., 2013, 135(40), 14956–14959 CrossRef CAS PubMed.
- Q. Q. Wu, Z. F. Xiao, X. J. Du and Q. H. Song, Chem.–Asian J., 2013, 8(11), 2564–2568 CrossRef CAS PubMed.
- L. Li, J. Y. Ge, H. Wu, Q. H. Xu and S. Q. Yao, J. Am. Chem. Soc., 2012, 134(29), 12157–12167 CrossRef CAS PubMed.
- S. Mizukami, S. Okada, S. Kimura and K. Kikuchi, Inorg. Chem., 2009, 48(16), 7630–7638 CrossRef CAS PubMed.
- D. Perez-Sala, D. Collado-Escobar and F. Mollinedo, J. Biol. Chem., 1995, 270(11), 6235–6242 CrossRef CAS PubMed.
- B. C. Dickinson, D. Srikun and C. J. Chang, Curr. Opin. Chem. Biol., 2010, 14(1), 50–56 CrossRef CAS PubMed.
- T. Ueno and T. Nagano, Nat. Methods, 2011, 8(8), 642–645 CrossRef CAS PubMed.
- Z. G. Yang, J. F. Cao, Y. X. He, J. H. Yang, T. Kim, X. J. Peng and J. S. Kim, Chem. Soc. Rev., 2014, 43(13), 4563–4601 RSC.
- B. C. Dickinson and C. J. Chang, J. Am. Chem. Soc., 2008, 130(30), 9638–9639 CrossRef CAS PubMed.
- E. Tomat, E. M. Nolan, J. Jaworski and S. J. Lippard, J. Am. Chem. Soc., 2008, 130(47), 15776–15777 CrossRef CAS PubMed.
- S. S. Zhu, W. Y. Lin and Y. Lin, Dyes Pigm., 2013, 99(2), 465–471 CrossRef CAS.
- H. L. Li, H. Guan, X. R. Duan, J. Hu, G. R. Wang and Q. Wang, Org. Biomol. Chem., 2013, 11(11), 1805–1809 RSC.
- T. A. Krulwich, G. Sachs and E. Padan, Nat. Rev. Microbiol., 2011, 9(5), 330–343 CrossRef CAS PubMed.
- G. Loving and B. Imperiali, J. Am. Chem. Soc., 2008, 130(41), 13630–13638 CrossRef CAS PubMed.
- C. T. Chu, D. J. Levinthal, S. M. Kulich, E. M. Chalovich and D. B. DeFranco, Eur. J. Biochem., 2004, 271, 2060–2066 CrossRef CAS PubMed.
- C. J. Stephanson, A. M. Stephanson and G. P. Flanagan, J. Med. Food, 2003, 6(3), 249–253 CrossRef CAS PubMed.
- L. Yuan, W. Y. Lin and J. Song, Chem. Commun., 2010, 46(42), 7930–7932 RSC.
- J. T. Hou, K. Lin, J. Yang, K. K. Yu, Y. X. Liao, Y. Z. Ran, Y. H. Liu, X. D. Zhou and X. Q. Yu, Chem. Commun., 2015, 51(31), 6781–6784 RSC.
- B. L. Predmore, D. J. Lefer and G. Gojon, Antioxid. Redox Signaling, 2012, 17(1), 119–140 CrossRef CAS PubMed.
- L. Li, M. Bhatia and P. K. Moore, Curr. Opin. Pharmacol., 2006, 6(2), 125–129 CrossRef CAS PubMed.
- G. D. Yang, L. Y. Wu, B. Jiang, W. Yang, J. S. Qi, K. Cao, Q. H. Meng, A. K. Mustafa, W. T. Mu, S. M. Zhang, S. H. Snyder and R. Wang, Science, 2008, 322(5901), 587–590 CrossRef CAS PubMed.
- L. Wu, W. Yang, X. Jia, G. Yang, D. Duridanova, K. Cao and R. Wang, Lab. Invest., 2008, 89(1), 59–67 CrossRef PubMed.
- L. F. Hu, M. Lu, Z. Y. Wu, P. T. Wong and J. S. Bian, Mol. Pharmacol., 2009, 75(1), 27–34 CrossRef CAS PubMed.
- W. H. Li, W. Sun, X. Q. Yu, L. P. Du and M. Y. Li, J. Fluoresc., 2013, 23(1), 181–186 CrossRef CAS PubMed.
- B. F. Chen, W. Li, C. Lv, M. M. Zhao, H. W. Jin, H. F. Jin, J. B. Du, L. R. Zhang and X. J. Tang, Analyst, 2013, 138(3), 946–951 RSC.
- M. Y. Wu, K. Li, J. T. Hou, Z. Huang and X. Q. Yu, Org. Biomol. Chem., 2012, 10(41), 8342–8347 RSC.
- M. Cigáň, J. Gašpar, K. Gáplovská and J. Holeksiova, New J. Chem., 2016, 40(10), 8946–8953 RSC.
- G. Signore, R. Nifosi, L. Albertazzi, B. Storti and R. Bizzarri, J. Am. Chem. Soc., 2010, 132(4), 1276–1288 CrossRef CAS PubMed.
- D. Udhayakumari, S. Naha and S. Velmathi, Anal. Methods, 2017, 9(4), 552–578 RSC.
- N. I. Georgiev, A. M. Asiri, A. H. Qusti, K. A. Alamry and V. B. Bojinov, Dyes Pigm., 2014, 102, 35–45 CrossRef CAS.
- S. L. Casciato, H. M. Liljestrand and J. A. Holcombe, Anal. Chim. Acta., 2014, 813, 77–82 CrossRef CAS PubMed.
|
This journal is © The Royal Society of Chemistry 2020 |
Click here to see how this site uses Cookies. View our privacy policy here.