DOI:
10.1039/C9RA10106C
(Paper)
RSC Adv., 2020,
10, 7126-7133
Heating-up synthesis of cesium bismuth bromide perovskite nanocrystals with tailored composition, morphology, and optical properties†
Received
3rd December 2019
, Accepted 8th February 2020
First published on 17th February 2020
Abstract
This study represents the heating-up synthesis of lead-free cesium bismuth bromide perovskite nanocrystals (NCs). CsBr and BiBr3 precursors are used to synthesize uniform and phase-pure cesium bismuth bromide NCs, and the reaction is performed via an injection-free, heating-up method in the presence of a solvent mixture with a high boiling point. The size and composition of cesium bismuth bromide NCs are readily controlled by changing the reaction time, temperature, and amount of surfactant added to the reaction mixture. Upon heating, sequential phase evolution occurs, resulting in the formation of kinetically stable BiOBr in the early reaction stages, which transformed into the thermodynamically stable Cs3BiBr6 and Cs3Bi2Br9 with an increase in either the reaction time or the reaction temperature. Furthermore, the absorption and photoluminescence properties of Cs3BiBr6 and Cs3Bi2Br9 NCs are characterized to investigate their composition-dependent optical properties. This work provides the potential to synthesize various types of lead-free perovskite NCs by tailoring the size and compositions.
Introduction
Halide perovskites with an ABX3 (A, B = cation, X = halide) structure have been extensively studied for application to electronic and optoelectronic devices.1–3 Perovskite nanocrystals (NCs) in particular have attracted much attention owing to their unique photoluminescence (PL) properties.4,5 Perovskite NC band gaps are tunable by changing the size, shape, and compositions of the NCs,6,7 which enables the tailoring of the absorption and emission wavelengths of the NCs. Among a variety of perovskite NCs, lead-based perovskite NCs are particularly interesting owing to their outstanding luminescent properties.8,9 Lead-halide perovskite NCs exhibit very high photoluminescence quantum yields (PLQYs), at higher than 90%,10 narrow absorption and emission band widths (FWHM is 12–25 nm) owing to the quantum confinement effect,11,12 and short fluorescence lifetimes.13,14 In addition, the size, shape, and compositions of these NCs can be easily changed by controlling the reaction parameters15–18 and post-synthetic treatments;19–25 this allows for the facile management of spectral conversion. However, despite this outstanding potential, lead-based perovskite NCs have heavy metal composition, which makes it potentially toxic to the environment and human health.26 Therefore, many researchers have made considerable effort to develop lead-free perovskite NCs with superior optical properties.
Various lead-free perovskite NCs using tin,27,28 bismuth,29–32 and antimony,33,34 have been investigated. Among them, Bi-based perovskite NCs exhibit the potential for stability and environment-friendliness. Bismuth is a less-toxic heavy metal, which reduces concern regarding environmental and health issues for Bi-based NCs.35 In addition, the luminescent properties of Bi-based perovskite NCs have been tremendously improved along the development of synthetic methods for the preparation of these NCs.36–38 After Park et al. discovered the synthesis of a A3Bi2X9 (A = methylammonium or cesium) perovskite structure,29 the colloidal synthesis of A3Bi2X9 NCs using the hot-injection method was demonstrated.39 Yang et al. reported on a ligand-assisted re-precipitation method (LARP) for the synthesis of Cs3Bi2Br9 NCs; their method showed a 4.5% PLQY.40 Moreover, Leng et al. synthesized this NC by using ethanol as the antisolvent; the PLQY reached approximately 20% with this method.41 Recently, the synthesis of cesium bismuth bromide with Cs3BiBr6 composition has been reported in the literature.37,42,43 In addition, many studies have been carried out to improve the stability of perovskite; for instance, the core–shell or double perovskite structure (A2BB'X6) has been explored.44 Although various procedures for the preparation of Bi-based perovskite NCs have been developed, synthetic methods to systematically tune the size and shape of Bi-based perovskite NCs and methods to tailor their optical properties have been rarely studied.
Among the various types of colloidal synthetic methods, the heating-up process for the synthesis of inorganic NCs has been widely studied.45–47 The heating-up method is a one-pot and injection-free process designed to synthesize highly uniform NCs and to tailor the sizes and shapes of the NCs.48 In this process, metal precursors and solvents are placed in reaction vessels and the reaction temperatures increases. At the high temperature, the nucleation and growth of NCs is induced to form crystalline NCs. Since such a high temperature injection process is typically not used in synthesis, the heating-up method offers a relatively safe and controllable synthetic process, which is potentially beneficial for mass production. Various types of NCs are synthesized using the heating-up process; for example, metal oxide,49,50 metal chalcogenides,51,52 and lanthanide NCs.53,54 Many literatures note that the size and shape of NCs can be easily controlled by changing reaction parameters such as reaction time55 and temperature,56,57 types of metal precursors,47 and the presence of shape directing reagent58–61 during preparation. Despite the various advantages of the heating-up process, heating-up process to synthesize bismuth perovskite NCs has not been extensively studied thus far. In addition, there are very few papers on controlling the size and shape of perovskite NCs using the heating-up process.
In this paper, we demonstrate a method of injection-free heating-up synthesis of cesium bismuth bromide NCs which allows for the tuning of the size, shape, and composition of perovskite NCs. CsBr and BiBr3 precursors are used to synthesize uniform and phase-pure perovskite NCs. We observed that the phase evolution of Bi-based NCs occurs during the heating-up reaction. At the early reaction stages, BiOBr NCs are formed from BiBr3 precursors. In the presence of CsBr at a higher reaction temperature, BiOBr NCs are transformed into Cs3BiBr6 phase NCs. With further increase in the reaction temperature, the composition of the NCs transform from Cs3BiBr6 to trigonal Cs3Bi2Br9 nanoplates (NPs); in contrast, metallic Bi is formed in the absence of CsBr. We demonstrate that the size and shape of Cs3Bi2Br9 NCs are tuned by changing the amount of oleic acid added into the reaction solution and by modulating the temperature. In addition, we analyze the optical properties of Cs3BiBr6 NCs and Cs3Bi2Br9 NPs to understand the effect of the composition of NCs on their photoluminescence.
Experimental section
Chemicals
Cesium bromide (99.999%, trace metals basis), oleic acid (tech, 90%), 1-octadecene (90%) and oleylamine (tech, 70%) were purchased from Sigma-Aldrich and bismuth bromide (97%) was purchased from Alfa Aesar. All chemicals were used without any further purification.
Heating-up synthesis of Cs3BiBr6 perovskite NCs
1.072 g of CsBr and 0.252 g of BiBr3 were added to a 100 mL three-necked flask containing 20 mL of 1-octadecene (ODE), 1.5 mL of oleylamine (OM), and 1.5 mL of oleic acid (OA). The reaction mixture was heated to 105 °C and the temperature was maintained for an hour under vacuum to remove volatile solvents. After degassing, the reaction mixture was further heated to 160 °C for the synthesis of perovskite NCs. After 60 min of reaction, the solution was cooled to room temperatures. The reaction mixture was diluted with anhydrous toluene and centrifuged at 3000 rpm for 15 s to precipitate unreacted cesium bromide and BiOBr nanoplate byproducts. After precipitates were discarded, the perovskite NCs were collected using centrifugation at 8000 rpm and 3 min.
Heating-up synthesis of Cs3Bi2Br9 perovskite NPs
For the synthesis of large sized NCs, 15 mL of OA, 1.5 mL of OM, and 7.5 mL of ODE were added to the reaction mixtures and the synthesis was performed under the same conditions as the aforementioned experiment.
Synthesis of pure BiOBr NPs
All reactants and processes were the same as those used in the synthesis of cesium bismuth bromide perovskite NCs, except that CsBr was absent. 0.252 g of BiBr3, 20 mL of ODE, 1.5 mL of OM and 1.5 mL of OA were loaded into a 100 mL three-neck flask. The mixture was then heated to 105 °C under vacuum for 1 h. After this, the mixture was heated to 135 °C and this temperature was maintained for 1 h to form BiOBr nanoplates. The nanoparticles were purified with toluene and methanol, and hexane was used as the storage solvent.
Characterization
Transmission electron microscopy (TEM) images were recorded by a JEOL JEM-2100 operating at 200 kV. High-Resolution Transmission Electron Microscopy (HRTEM) images were collected by a JEOL-F200 and JEOL JEM-3010. Energy Dispersive X-ray Spectroscopy (EDS) measurement and mapping were performed using JEOL JEM-ARM200F. Scanning electron microscopy (SEM) images were recorded using Carl Zeiss SIGMA operating at 5 kV. X-ray diffraction (XRD) was conducted using Bruker-AXS New D8-Advance. X-ray photoelectron spectroscopy (XPS) measurements were performed using ThermoFisher Scientific K-alpha. Absorption and transmission spectra were collected with a JASCO V-770 UV-VIS-NIR spectrometer. Photoluminescence (PL) spectra were obtained using EDINBURGH FS5. The PLQY was measured using a fluorimeter equipped with an integrating sphere. Time-resolved photoluminescence decay data were collected using a HORIBA Fluorolog-3 with a single photon-counting photomultiplier tube (PMT) under a 374 nm pulsed laser diode.
Result and discussion
Colloidal cesium bismuth bromide perovskite NCs are prepared via heating-up synthesis using CsBr and BiBr3 precursors. In this experiment, BiBr3 and CsBr are added together into the reaction mixture with an excess of Cs precursors. As CsBr does not completely dissolve in the ODE, OM, and OA solvent mixture, the reaction is conducted between solid-phase CsBr and dissolved BiBr3 in the solution. Fig. 1a–d displays TEM images of the cesium bismuth bromide NCs. The low-magnification image shows the monodisperse cesium bismuth bromide NCs with a narrow size distribution. The size of the cesium bismuth bromide NCs is 14.22 nm ± 1.72 nm, as calculated by statistical size analysis of the TEM images. The cesium bismuth bromide NCs are linearly arrayed into one-dimensional chains, as displayed in Fig. 1a and b. At the same time, the one-dimensional chains are also self-assembled into close-packed structures to form extended two-dimensional superlattice arrays (Fig. 1c and d). HRTEM images show single-crystalline structures of the cesium bismuth bromide NCs (Fig. 1e). Fig. 1f shows the XRD pattern of the cesium bismuth bromide NCs. The XRD pattern is very similar to that of the Cs3BiBr6 composition reported in the recent literature.37,42,43 The STEM-EDS analysis also reveals the composition of the Cs3BiBr6 NCs. Fig. 1g shows the dark-field TEM image of the Cs3BiBr6 NCs, and Fig. 1h–j are the STEM-EDS elemental mapping images, which exhibit a homogeneous elemental distribution of Cs, Bi, and Br in the NCs. The relative ratio of Cs
:
Bi
:
Br atoms is 34.96
:
11.18
:
53.86, which reveals that the composition of the as-synthesized NCs is Cs3BiBr6.
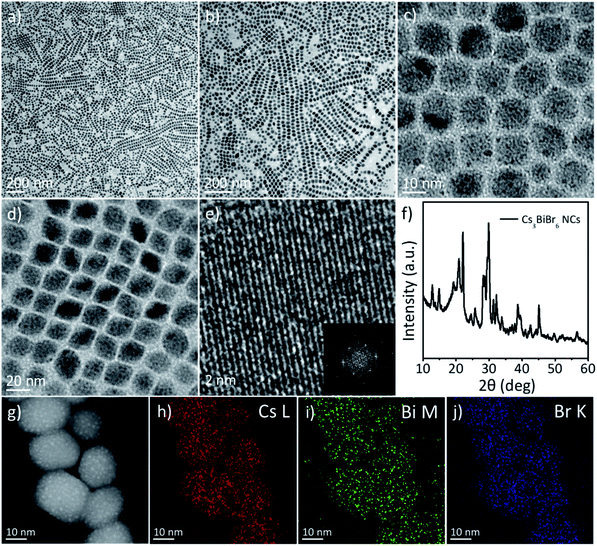 |
| Fig. 1 (a and b) Low-magnification and (c and d) high-magnification TEM images of colloidal Cs3BiBr6 NCs, (e) high-resolution TEM image and FFT image of Cs3BiBr6 NCs, (inset) (f) XRD pattern of Cs3BiBr6 NCs. (g) Dark-field TEM image of Cs3BiBr6 NCs. (h–j) STEM-EDS mapping of Cs3BiBr6 NCs. | |
The synthesis of cesium bismuth bromide with the composition Cs3BiBr6 is readily controlled by increasing the amount of OA added into the reaction mixture. As the amount of OA increases from 1.5 mL to 15 mL, two-dimensional Cs3Bi2Br9 NPs are synthesized. Fig. 2a shows TEM images of the Cs3Bi2Br9 NPs. The size of the Cs3Bi2Br9 NPs is approximately 200–400 nm. HRTEM images show the single-crystalline structure of a Cs3Bi2Br9 nanoplate (Fig. 2d and S2, ESI†). Fast Fourier Transform (FFT) patterns of the HRTEM image exhibit hexagonal symmetry, representing the trigonal structure of the Cs3Bi2Br9 NPs (Fig. 2d, inset). The STEM-EDS elemental mapping image exhibits a homogeneous elemental distribution of Cs, Bi, and Br in the NPs. The XRD patterns also indicate the trigonal diffraction of the Cs3Bi2Br9 NPs (JCPDS no. 44-0714, Fig. 2i). Compositional information is further confirmed by XPS measurements. XPS profiles confirm that the Cs3Bi2Br9 NPs comprise Cs, Bi, and Br (Fig. 2j–l).
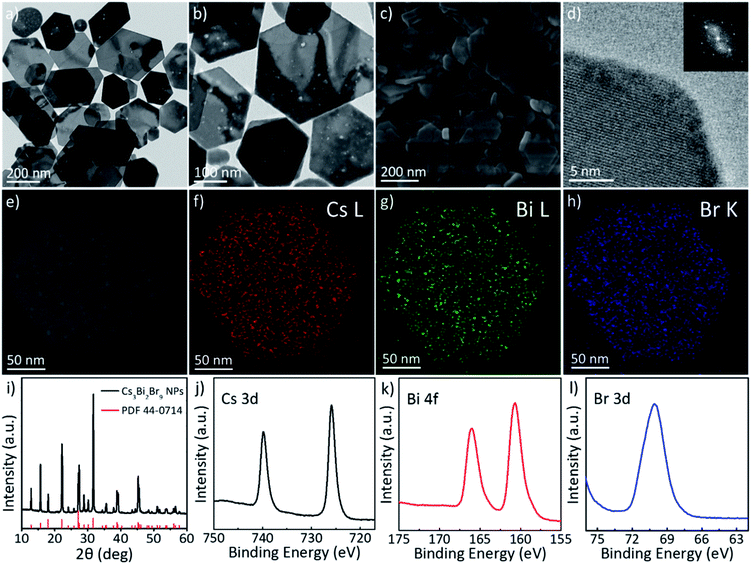 |
| Fig. 2 (a) low-magnification, (b) high-magnification TEM images and (c) SEM image of Cs3Bi2Br9 NPs. (d) HRTEM image of Cs3Bi2Br9 NPs. (e) Dark-field TEM image of Cs3Bi2Br9 NPs. (f–h) EDS mapping of Cs3Bi2Br9 NPs. (i) XRD patterns of trigonal Cs3Bi2Br9 NPs. (j–l) XPS profiles of Cs3Bi2Br9 NPs. | |
During the heating-up process, gradual color change of the reaction solution is observed. When 1.5 mL of OA is used for the reaction, the color of the solution is transparent up to 105 °C. Upon heating, the solution color changes to white at approximately 135 °C and then changes to bright yellow at 160 °C, indicating the formation of Cs3BiBr6 NCs. In order to understand the growth mechanism of perovskite NCs during the heating-up process, we systematically varied the reaction temperature and investigated the crystal structure of the as-synthesized NCs. When the reaction is performed at 135 °C in the presence of 1.5 mL of OA, BiOBr NPs are formed as a final white product. Fig. S3a–c (ESI†) show TEM and HRTEM images of the BiOBr NPs, which exhibit a rectangular nanoplate morphology. The size of the BiOBr NPs is approximately 25.5 nm. Fig. S3d (ESI†) shows XRD pattern of BiOBr NPs. The XRD pattern reveals pure tetragonal BiOBr phases (JCPDS no. 73-0348). The (101) and (102) diffraction planes are relatively broad compared to the other planes, indicating that the top and bottom surfaces of the nanoplates are (001) planes.
Up to a reaction temperature of 160 °C, the BiOBr NPs are predominantly formed as final products and there is no evidence of reaction between the CsBr and BiBr3 precursors. When the reaction temperature is further increased to over 160 °C, CsBr precursors are involved in the reaction to form slightly yellowish Cs3BiBr6 NCs. Cs3BiBr6 NCs are predominantly formed at 160 °C with BiOBr NPs as the residual. When the temperature increases to approximately 170 °C, Cs3Bi2Br9 NPs are synthesized predominantly, as shown in the XRD patterns (Fig S4, ESI†). The Cs3Bi2Br9 NCs are predominantly formed even at a higher reaction temperature, up to 300 °C, without the formation of other phases of Bi-based materials. This reveals that the Cs3Bi2Br9 NCs do not transform into the metallic Bi phase even at higher temperatures. This phase transition signifies that BiOBr is a kinetically stable phase and forms in an early reaction stage. In the presence of CsBr, thermodynamically stable Cs3BiBr6 NCs are initially formed. When sufficient energy is provided by an increase in the reaction temperature, trigonal phase Cs3Bi2Br9 NCs are formed. When the reaction temperature is set above 180 °C while in the absence of CsBr, the reaction solution becomes gray in color, and the XRD pattern indicates that metallic Bi is present, which indicates that the BiOBr phase is reduced to the metallic Bi phase in the absence of CsBr. Even in the presence of CsBr, the Bi phase can appear at a higher temperature and a lower amount of OA in the reaction mixture, which is attributed to the structure transformation from the BiOBr to Bi phases (Fig. S5, ESI†).
To further understand phase evolution from BiOBr NPs to Cs3Bi2Br9 NCs during the reaction, aliquots are taken at different time intervals at a fixed temperature and crystal structure is investigated. Fig. 3 displays XRD patterns of the Bi-based NCs taken 15 min from the time interval at 170 °C. The reaction is performed in presence of 1.5 mL of OA. XRD reveals that BiOBr is predominantly formed during the early stages of the reaction. After 60 minutes of reaction, phase transformation from BiOBr NPs to Cs3Bi2Br9 NCs occurs to form the mixture of BiOBr and Cs3Bi2Br9 NCs. As the reactions are performed at 170 °C, the Cs3BiBr6 phase is not indicated by the XRD patterns. After 90 minutes of reaction, BiOBr NPs are completely converted into Cs3Bi2Br9 NCs. This trend confirms that the kinetically stable BiOBr forms in the early stage of the reaction and is then transformed into Cs3Bi2Br9 NCs, as illustrated in Fig. 4. Since much smaller Cs3BiBr6 NCs are formed from BiOBr NPs during the phase transformation, Cs3BiBr6 NCs are formed while dissolution of BiOBr NPs occurs. We also observed that the amount of OA plays an important role in the phase transition during the reaction. As the amount of OA increases, the Cs3BiBr6 phase is diminished, and Cs3Bi2Br9 forms at a lower reaction temperature. In the presence of 1.5 mL of OA, Cs3Bi2Br9 NCs begin to form at 170 °C. When the amount of OA increases to 15 mL, the reaction solution turns yellow even below 100 °C (Fig. S6, ESI†), which is lower than the formation temperature of BiOBr (Fig. 4). This indicating that the presence of OA facilitates the formation of Cs3Bi2Br9 NPs, which may be attributed to the interaction between OA surfactants and CsBr salts promoting the dissolution of CsBr salts in the non-polar solvent. The ratio of Bi precursors to Cs precursors is an important parameter to control the growth of Cs3Bi2Br9 NCs. As CsBr is not completely soluble in non-polar solvents, excess CsBr is added to facilitate the growth of NCs during the reaction, instead of adding a stoichiometric amount of the CsBr precursors. If Cs and Bi are used at a stoichiometric ratio, the phase transformation of BiOBr to Cs3Bi2Br9 is delayed and a relatively large amount of BiOBr remains as a final product.
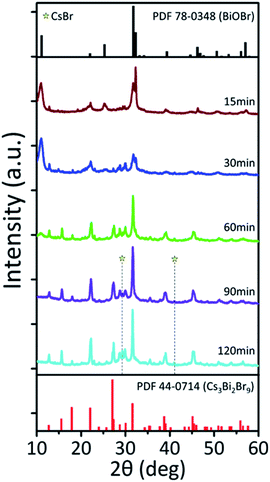 |
| Fig. 3 Reaction time-dependent phase evolution. XRD patterns of Bi-based NCs synthesized at 170 °C with different time intervals. | |
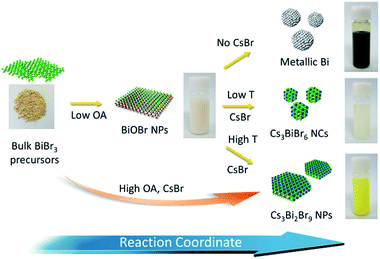 |
| Fig. 4 A schematic representation of the formation of Cs3BiBr6 NCs and Cs3Bi2Br9 NPs from BiBr3 and CsBr precursors. | |
We investigated the composition-dependent optical properties of Cs3BiBr6 NCs and Cs3Bi2Br9 NPs. Fig. 5a displays the absorption spectra of the small Cs3BiBr6 NCs and large Cs3Bi2Br9 perovskite NPs. Band absorption spectra in blue is observed in the UV region. The band gap of large Cs3Bi2Br9 is 2.67 eV (464 nm), which is close to the band gap of bulk Cs3Bi2Br9.31 On the other hand, strong blue-shift of the band gap is observed for smaller Cs3BiBr6 NCs. Compared to Cs3Bi2Br9 NPs, the onset of the absorption band-edge is blue-shifted to 3.05 eV (406 nm), indicating an increased optical band-gap in addition, very narrow first excitonic absorption is observed at 383.5 nm of peak position. We also characterize the PL properties of Cs3BiBr6 NCs and Cs3Bi2Br9 NPs. Fig. 5b and c exhibit the excitation and emission spectra of Cs3BiBr6 NCs and Cs3Bi2Br9 NPs, respectively. While large Cs3Bi2Br9 NPs show weak photoluminescence properties, with an emission maximum of 461 nm, deep blue luminescence is observed in small Cs3BiBr6 NCs, with an emission maximum of 435 nm. The PLQY of Cs3BiBr6 NCs is measured as 6.03%, which is one of the highest values among solvothermally synthesized Cs3BiBr6 NCs without further treatments. Fig. 5d shows the PL decay curves of Cs3BiBr6 NCs and Cs3Bi2Br9 NPs. Time-resolved PL decays are monitored at 440 nm and 461 nm for Cs3BiBr6 NCs and Cs3Bi2Br9 NPs, respectively, under 374 nm of excitation. All decay curves were fitted according to bi-exponential functions, and the fitting results are listed in Table S1.† The PL decay is attributed to sub-bandgap transition, charge carrier recombination processes, and sub-bandgap state emission (trap emission).40,62–64 The PL decay of Cs3BiBr6 NCs is much longer than that of Cs3Bi2Br9 NPs, revealing the higher PLQY of Cs3BiBr6 via radiative recombination (Table S1, ESI†).
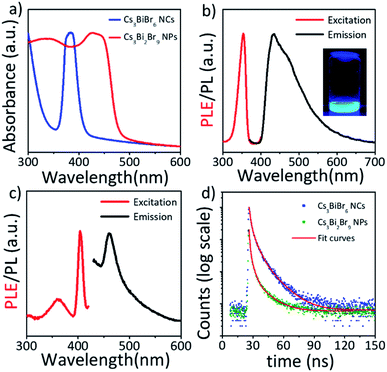 |
| Fig. 5 Optical properties of Cs3BiBr6 NCs and Cs3Bi2Br9 NPs. (a) Absorption spectrum of Cs3BiBr6 NCs (blue) and Cs3Bi2Br9 NPs (red). (b) Excitation and emission spectrum of Cs3BiBr6 NCs. Emission spectra is collected under an excitation wavelength of 360 nm and the excitation spectra is measured under an emission wavelength of 440 nm. (c) Excitation and emission spectra of Cs3Bi2Br9 NPs. Emission spectra is collected under an excitation wavelength of 358 nm and the excitation spectra is measured under an emission wavelength of 461 nm. (d) Time-resolved photoluminescence decay of Cs3BiBr6 NCs (blue) and Cs3Bi2Br9 NPs (red) under 374 nm excitation. | |
Conclusions
In this study, we demonstrate the heating-up synthesis of lead-free cesium bismuth bromide perovskite NCs. The size and composition of perovskite NCs is readily controlled by changing the amount of OA added to the reaction and by adjusting the reaction temperature. In the presence of 1.5 mL of OA, the small Cs3BiBr6 NCs are synthesized with uniform size distribution. When the amount of OA added in the reaction or the reaction temperature increases, large Cs3Bi2Br9 NPs are obtained as a final product. We systematically investigated the growth mechanism of cesium bismuth bromide NCs during the heating up process. In the presence of 1.5 mL of OA, phase evolution from BiOBr to Cs3BiBr6 occurs upon increasing the reaction temperature, as confirmed by XRD and microscopic measurements. Thermodynamically stable Cs3Bi2Br9 NPs are formed in higher temperature reactions, with extended reaction times, and by increasing the amount of OA in the reaction mixture. We investigate the optical properties of Cs3BiBr6 NCs and Cs3Bi2Br9 NPs. The band-gap of small Cs3BiBr6 NCs and large Cs3Bi2Br9 NPs are 3.05 eV and 2.67 eV, respectively. Blue photoluminescence is observed owing to the presence of small Cs3BiBr6 NCs, while large Cs3Bi2Br9 NPs show faint photoluminescence properties. Photoluminescence quantum yield of small Cs3BiBr6 NCs is 6.03%, which is the highest value of the high-temperature solvothermally synthesized Cs3BiBr6 NCs. The heating-up method provides a novel approach for synthesizing perovskite based NCs with readily controlled size, shape, and composition. We believe that our approach provides the potential to synthesize various types of lead-free perovskite NCs.
Conflicts of interest
There are no conflicts to declare.
Acknowledgements
This research was supported by the Creative Materials Discovery Program through the National Research Foundation of Korea (NRF), funded by Ministry of Science and ICT (NRF-2018M3D1A1059001) and by the National Research Foundation of Korea (NRF) grant funded by the Korea government (MSIT) (NRF-2019R1A4A1028700 and NRF-2017R1C1B1005236). It was also supported by the Korea Institute of Energy Technology Evaluation and Planning (KETEP) under a Grant, the Ministry of Trade, Industry & Energy (MOTIE) of the Republic of Korea (No. 20182020109430).
Notes and references
- M. Xiao, F. Huang, W. Huang, Y. Dkhissi, Y. Zhu, J. Etheridge, A. Gray-Weale, U. Bach, Y. B. Cheng and L. Spiccia, Angew. Chem., Int. Ed., 2014, 53, 9898–9903 CrossRef CAS PubMed
. - J. Lin, M. Lai, L. Dou, C. S. Kley, H. Chen, F. Peng, J. Sun, D. Lu, S. A. Hawks and C. Xie, Nat. Mater., 2018, 17, 261 CrossRef CAS PubMed
. - X. Li, Y. Wu, S. Zhang, B. Cai, Y. Gu, J. Song and H. Zeng, Adv. Funct. Mater., 2016, 26, 2435–2445 CrossRef CAS
. - S. T. Ha, X. Liu, Q. Zhang, D. Giovanni, T. C. Sum and Q. Xiong, Adv. Opt. Mater., 2014, 2, 838–844 CrossRef CAS
. - Q. Lin, A. Armin, R. C. R. Nagiri, P. L. Burn and P. Meredith, Nat. Photon., 2015, 9, 106 CrossRef CAS
. - Q. A. Akkerman, S. G. Motti, A. R. Srimath Kandada, E. Mosconi, V. D'Innocenzo, G. Bertoni, S. Marras, B. A. Kamino, L. Miranda and F. De Angelis, J. Am. Chem. Soc., 2016, 138, 1010–1016 CrossRef CAS PubMed
. - V. D'Innocenzo, A. R. Srimath Kandada, M. De Bastiani, M. Gandini and A. Petrozza, J. Am. Chem. Soc., 2014, 136, 17730–17733 CrossRef
. - H.-S. Kim, C.-R. Lee, J.-H. Im, K.-B. Lee, T. Moehl, A. Marchioro, S.-J. Moon, R. Humphry-Baker, J.-H. Yum and J. E. Moser, Sci. Rep., 2012, 2, 591 CrossRef PubMed
. - D. Zhang, S. W. Eaton, Y. Yu, L. Dou and P. Yang, J. Am. Chem. Soc., 2015, 137, 9230–9233 CrossRef CAS PubMed
. - L. Protesescu, S. Yakunin, M. I. Bodnarchuk, F. Krieg, R. Caputo, C. H. Hendon, R. X. Yang, A. Walsh and M. V. Kovalenko, Nano Lett., 2015, 15, 3692–3696 CrossRef CAS
. - J. H. Song and S. Jeong, Nano Convergence, 2017, 4, 21 CrossRef
. - J. Butkus, P. Vashishtha, K. Chen, J. K. Gallaher, S. K. K. Prasad, D. Z. Metin, G. Laufersky, N. Gaston, J. E. Halpert and J. M. Hodgkiss, Chem. Mater., 2017, 29, 3644–3652 CrossRef CAS
. - G. Li, Z.-K. Tan, D. Di, M. L. Lai, L. Jiang, J. H.-W. Lim, R. H. Friend and N. C. Greenham, Nano Lett., 2015, 15, 2640–2644 CrossRef CAS PubMed
. - S. Yakunin, L. Protesescu, F. Krieg, M. I. Bodnarchuk, G. Nedelcu, M. Humer, G. De Luca, M. Fiebig, W. Heiss and M. V. Kovalenko, Nat. Commun., 2015, 6, 8056 CrossRef CAS PubMed
. - Y. Bekenstein, B. A. Koscher, S. W. Eaton, P. Yang and A. P. Alivisatos, J. Am. Chem. Soc., 2015, 137, 16008–16011 CrossRef CAS PubMed
. - W. Liu, Q. Lin, H. Li, K. Wu, I. Robel, J. M. Pietryga and V. I. Klimov, J. Am. Chem. Soc., 2016, 138, 14954–14961 CrossRef CAS
. - S. Sun, D. Yuan, Y. Xu, A. Wang and Z. Deng, ACS Nano, 2016, 10, 3648–3657 CrossRef CAS PubMed
. - H. Liu, Z. Wu, J. Shao, D. Yao, H. Gao, Y. Liu, W. Yu, H. Zhang and B. Yang, ACS Nano, 2017, 11, 2239–2247 CrossRef CAS PubMed
. - S. Bhaumik, S. A. Veldhuis, Y. F. Ng, M. Li, S. K. Muduli, T. C. Sum, B. Damodaran, S. Mhaisalkar and N. Mathews, Chem. Commun., 2016, 52, 7118–7121 RSC
. - M. Imran, P. Ijaz, D. Baranov, L. Goldoni, U. Petralanda, Q. Akkerman, A. L. Abdelhady, M. Prato, P. Bianchini and I. Infante, Nano Lett., 2018, 18, 7822–7831 CrossRef CAS PubMed
. - G. Pan, X. Bai, D. Yang, X. Chen, P. Jing, S. Qu, L. Zhang, D. Zhou, J. Zhu and W. Xu, Nano Lett., 2017, 17, 8005–8011 CrossRef CAS PubMed
. - T. Ahmed, S. Seth and A. Samanta, Chem. Mater., 2018, 30, 3633–3637 CrossRef CAS
. - B. Wang, C. Zhang, S. Huang, Z. Li, L. Kong, L. Jin, J. Wang, K. Wu and L. Li, ACS Appl. Mater. Interfaces, 2018, 10, 23303–23310 CrossRef CAS PubMed
. - D. Gao, B. Qiao, Z. Xu, D. Song, P. Song, Z. Liang, Z. Shen, J. Cao, J. Zhang and S. Zhao, J. Phys. Chem. C, 2017, 121, 20387–20395 CrossRef CAS
. - B. A. Koscher, J. K. Swabeck, N. D. Bronstein and A. P. Alivisatos, J. Am. Chem. Soc., 2017, 139, 6566–6569 CrossRef CAS PubMed
. - A. H. Slavney, R. W. Smaha, I. C. Smith, A. Jaffe, D. Umeyama and H. I. Karunadasa, Inorg. Chem., 2016, 56, 46–55 CrossRef PubMed
. - T. C. Jellicoe, J. M. Richter, H. F. Glass, M. Tabachnyk, R. Brady, S. n. E. Dutton, A. Rao, R. H. Friend, D. Credgington and N. C. Greenham, J. Am. Chem. Soc., 2016, 138, 2941–2944 CrossRef CAS PubMed
. - Z. Tan, J. Li, C. Zhang, Z. Li, Q. Hu, Z. Xiao, T. Kamiya, H. Hosono, G. Niu and E. Lifshitz, Adv. Funct. Mater., 2018, 28, 1801131 CrossRef
. - B. W. Park, B. Philippe, X. Zhang, H. Rensmo, G. Boschloo and E. M. Johansson, Adv. Mater., 2015, 27, 6806–6813 CrossRef CAS PubMed
. - R. Nelson, K. Santra, Y. Wang, A. Hadi, J. Petrich and M. Panthani, Chem. Commun., 2018, 54, 3640–3643 RSC
. - K. K. Bass, L. Estergreen, C. N. Savory, J. Buckeridge, D. O. Scanlon, P. I. Djurovich, S. E. Bradforth, M. E. Thompson and B. C. Melot, Inorg. Chem., 2016, 56, 42–45 CrossRef PubMed
. - M. Gao, C. Zhang, L. Lian, J. Guo, Y. Xia, F. Pan, X. Su, J. Zhang, H. Li and D. Zhang, J. Mater. Chem. C, 2019, 7, 3688–3695 RSC
. - J. Zhang, Y. Yang, H. Deng, U. Farooq, X. Yang, J. Khan, J. Tang and H. Song, ACS Nano, 2017, 11, 9294–9302 CrossRef CAS PubMed
. - B. Pradhan, G. S. Kumar, S. Sain, A. Dalui, U. K. Ghorai, S. K. Pradhan and S. Acharya, Chem. Mater., 2018, 30, 2135–2142 CrossRef CAS
. - M. B. Johansson, H. Zhu and E. M. Johansson, J. Phys. Chem. Lett., 2016, 7, 3467–3471 CrossRef CAS
. - M. Leng, Z. Chen, Y. Yang, Z. Li, K. Zeng, K. Li, G. Niu, Y. He, Q. Zhou and J. Tang, Angew. Chem., Int. Ed., 2016, 55, 15012–15016 CrossRef CAS PubMed
. - Y. Tang, M. Liang, B. Chang, H. Sun, K. Zheng, T. Pullerits and Q. Chi, J. Mater. Chem. C, 2019, 7(11), 3369–3374 RSC
. - Y. Lou, M. Fang, J. Chen and Y. Zhao, Chem. Commun., 2018, 54, 3779–3782 RSC
. - Y. Zhang, J. Yin, M. R. Parida, G. H. Ahmed, J. Pan, O. M. Bakr, J.-L. Brédas and O. F. Mohammed, J. Phys. Chem. Lett., 2017, 8, 3173–3177 CrossRef CAS PubMed
. - B. Yang, J. Chen, F. Hong, X. Mao, K. Zheng, S. Yang, Y. Li, T. Pullerits, W. Deng and K. Han, Angew. Chem., Int. Ed., 2017, 56, 12471–12475 CrossRef CAS PubMed
. - M. Leng, Y. Yang, K. Zeng, Z. Chen, Z. Tan, S. Li, J. Li, B. Xu, D. Li and M. P. Hautzinger, Adv. Funct. Mater., 2018, 28, 1704446 CrossRef
. - H. Yang, T. Cai, E. Liu, K. Hills-Kimball, J. Gao and O. Chen, Nano Res., 2020, 13, 282–291 CrossRef
. - S. E. Creutz, H. Liu, M. E. Kaiser, X. Li and D. R. Gamelin, Chem. Mater., 2019, 31, 4685–4697 CrossRef CAS
. - L. Zhou, Y. F. Xu, B. X. Chen, D. B. Kuang and C. Y. Su, Small, 2018, 14, 1703762 CrossRef PubMed
. - C. B. Murray, S. Sun, W. Gaschler, H. Doyle, T. A. Betley and C. R. Kagan, IBM J. Res. Dev., 2001, 45, 47–56 CAS
. - T. Paik, S.-H. Hong, E. A. Gaulding, H. Caglayan, T. R. Gordon, N. Engheta, C. R. Kagan and C. B. Murray, ACS Nano, 2014, 8, 797–806 CrossRef CAS PubMed
. - J. Park, K. An, Y. Hwang, J.-G. Park, H.-J. Noh, J.-Y. Kim, J.-H. Park, N.-M. Hwang and T. Hyeon, Nat. Mater., 2004, 3, 891 CrossRef CAS PubMed
. - S. G. Kwon and T. Hyeon, Small, 2011, 7, 2685–2702 CrossRef CAS PubMed
. - Y. w. Jun, J. s. Choi and J. Cheon, Angew. Chem., Int. Ed., 2006, 45, 3414–3439 CrossRef CAS PubMed
. - Y. C. Cao, J. Am. Chem. Soc., 2004, 126, 7456–7457 CrossRef CAS PubMed
. - L. Wu, S.-Y. Chen, F.-J. Fan, T.-T. Zhuang, C.-M. Dai and S.-H. Yu, J. Am. Chem. Soc., 2016, 138, 5576–5584 CrossRef CAS PubMed
. - S. E. Creutz, R. Fainblat, Y. Kim, M. C. De Siena and D. R. Gamelin, J. Am. Chem. Soc., 2017, 139, 11814–11824 CrossRef CAS PubMed
. - F. Wang, Y. Han, C. S. Lim, Y. Lu, J. Wang, J. Xu, H. Chen, C. Zhang, M. Hong and X. Liu, Nature, 2010, 463, 1061 CrossRef CAS PubMed
. - F. T. Rabouw, P. T. Prins and D. J. Norris, Nano Lett., 2016, 16, 7254–7260 CrossRef CAS PubMed
. - L. Lian, G. Zhai, F. Cheng, Y. Xia, M. Zheng, J. Ke, M. Gao, H. Liu, D. Zhang and L. Li, CrystEngComm, 2018, 20, 7473–7478 RSC
. - V. F. Puntes, K. M. Krishnan and A. P. Alivisatos, Science, 2001, 291, 2115–2117 CrossRef CAS PubMed
. - H. Huang, A. S. Susha, S. V. Kershaw, T. F. Hung and A. L. Rogach, Adv. Sci., 2015, 2, 1500194 CrossRef PubMed
. - Y. Yang, Y. Jin, H. He, Q. Wang, Y. Tu, H. Lu and Z. Ye, J. Am. Chem. Soc., 2010, 132, 13381–13394 CrossRef CAS PubMed
. - H. Guo, Y. Chen, M. B. Cortie, X. Liu, Q. Xie, X. Wang and D.-L. Peng, J. Phys. Chem. C, 2014, 118, 9801–9808 CrossRef CAS
. - J. Shamsi, Z. Dang, P. Bianchini, C. Canale, F. Di Stasio, R. Brescia, M. Prato and L. Manna, J. Am. Chem. Soc., 2016, 138, 7240–7243 CrossRef CAS PubMed
. - A. Pan, B. He, X. Fan, Z. Liu, J. J. Urban, A. P. Alivisatos, L. He and Y. Liu, ACS Nano, 2016, 10, 7943–7954 CrossRef CAS PubMed
. - K. Wu, G. Liang, Q. Shang, Y. Ren, D. Kong and T. Lian, J. Am. Chem. Soc., 2015, 137, 12792–12795 CrossRef CAS PubMed
. - J. Li, L. Gan, Z. Fang, H. He and Z. Ye, J. Phys. Chem. Lett., 2017, 8, 6002–6008 CrossRef CAS PubMed
. - B. Yang, J. Chen, S. Yang, F. Hong, L. Sun, P. Han, T. Pullerits, W. Deng and K. Han, Angew. Chem., 2018, 130, 5457–5461 CrossRef
.
Footnote |
† Electronic supplementary information (ESI) available. See DOI: 10.1039/c9ra10106c |
|
This journal is © The Royal Society of Chemistry 2020 |
Click here to see how this site uses Cookies. View our privacy policy here.