DOI:
10.1039/C9RA10084A
(Review Article)
RSC Adv., 2020,
10, 7026-7047
Recent advances in the use of walnut (Juglans regia L.) shell as a valuable plant-based bio-sorbent for the removal of hazardous materials
Received
2nd December 2019
, Accepted 3rd February 2020
First published on 17th February 2020
Abstract
The effective use of agricultural by-products is definitely a major challenge in waste management. In the walnut fruit processing industry, large amounts of shells are produced as agricultural by-products and discarded or burned produced as fuel. Walnut (Juglans regia L.) is a valuable tree nut in the Juglandaceae family. The fruit is composed of four main parts: the kernel, the skin, the shell, and the husk. The importance of walnuts is mostly related to theirs valuable kernels. However, their shells are currently experiencing as much interest as their kernels due to the beneficial effects of the shells. In the past several years, walnut shell (WS) has been widely explored as a naturally inert plant-based biosorbent. In this review, we first highlight recent scientific literature regarding the development of adsorbents from WS in the form of carbon-based materials including unmodified/modified WS, and activated carbons (ACs). Next, we discuss the potential applications of WS-derived by-products as natural yet effective adsorbents for the removal of various hazardous materials including heavy metals (HMs), synthetic industrial dyes, and harmful chemicals.
1. Introduction
Currently, environmental contamination from various hazardous materials is a primary concern worldwide. It is a significant risk, and scientists believe that it endangers public health. For this reason, many recent studies have focused on the effective removal and elimination of such to reduce their harmful effects on living organisms.1 Annually, large amounts of toxic materials resulting from human industrial activities are discharged into the environment. Different synthetic dyes, various HM ions, oils derived from crude and processed petroleum, and pharmaceuticals are known to be significant hazardous compounds.2 Hazardous materials show carcinogenic and toxic effects, and thus is a growing interest worldwide for in the development of cost-effective, rapid, secure, regenerating and applicable biosorbents for the removal of such dangerous substances from industrial wastewaters before their discharge into terrestrial and aquatic ecosystems.3 Accordingly, the removal of different hazardous materials that threaten human health and affect various forms of life in aquatic ecosystems is now an important topic of study among environmental scientists. Currently, agricultural by-products and carbon-based products derived from waste crops seem to be the most attractive materials for the removal of various toxic chemicals because of their abundance, renewability, and cost-effectiveness. In recent years, various plant-based waste residues have been considered as adsorbents.4 Hazardous material separation using low-cost alternative adsorbents can be advantageous since provides a double advantage, i.e., waste product management and water purification. Additionally, the low-cost ACs derived from biomass similar or better than non-renewable coal-based granular activated carbons (GACs) and may successfully replace GACs. Consequently, the material used to produce ACs is critical because considerably affects the adsorption capacity. Nutshells, as standard and inexpensive agricultural waste materials, are used for this purpose.5
Walnut is as a popular tree nut that has been widely cultivated across the world for its valuable kernel. Inside the walnut fruit, the kernel is surrounded by three distinct layers called the seed coat, the shell, and the green husk. Unlike the kernel, the remaining parts of the fruit are regarded as waste products and are discarded without being put to any practical use.6 Recently, the walnut shell has gained increasing attention due to its high availability and several unique properties. One of the main and recently highlighted applications of WS by-products is the use of processed or unprocessed WS as biosorbent.7 Accordingly, this review aims to summarize up-to-date developments in the preparation and application of unmodified and modified WS in the form of ACs, as well as chemically modified WS in adsorption procedures for the removal of HMs, dyes, oils, pharmaceuticals and other hazardous substances from aqueous solutions. Additionally, important parameters for obtaining optimal adsorption conditions for different hazardous materials are highlighted. Furthermore, the thermodynamics of the adsorption reactions are discussed. Building upon the information derived from the literature review, concluding remarks and suggestions for future research are provided.
2. Walnuts
The Persian, English, or common walnut is valuable tree nut in the family Juglandaceae. Due to its valuable kernel, walnut is commercially cultivated in most parts of the world. It is considered a high-density nutrient that is rich in protein and essential fatty acids.8,9 In recent years, there has been a rapid increase in global walnut production, particularly in Asian countries, which appreciate these products for their high nutritional value and antioxidative potential.10 It has been reported that walnut kernels have high amounts of phenolic compounds and that their consumption provide many beneficial effects for human health.11–26 Black walnut (Juglans nigra L.) is another important species of this genus that is valuable for its wood.27 The fruit and timber of the common walnut have been extensively used in human nutrition and industrial applications.18 The shell and husk are the main by-products of the walnut fruit and are produced in high amounts upon processing the walnut fruit to obtain the kernel. Recent investigations of these by-products demonstrated that they contain valuable compounds including phenolics.6,28–30 The green husk of the walnut fruit is available in high amounts. Recently, Oliveira, et al.31 recognized the aqueous extract of the low-cost green husk as an excellent natural source of phenolic compounds with antimicrobial and antiradical activities. The leaves of walnut are also considered another source of beneficial medicinal compounds and have been comprehensively applied in folk medicine for the treatment of hyperhidrosis, skin inflammations, and ulcers. Additionally, its prepared extracts or identified constituents show anti-septic, anti-helminthic, anti-diarrheic, antioxidant and astringent properties.20,32–38 The other parts of the walnut tree, such as the branches and shoots have recently been used for the extraction of useful compounds with antioxidant and antimicrobial properties.39,40 Moreover, walnut kernels are valuable nutraceuticals considering that their regular consumption is reported to decrease the risk of human coronary heart disease.15,17–19,22,24,25,41–44 The health benefits of walnut are commonly attributed to its chemical composition,13,32,45 as it is known as a good source of tocopherols and tocotrienols, proteins, fibre, sterols, folate, essential fatty acids, melatonin, tannins, and other polyphenols.11,13,16,28,36 Studies on different types of nuts have indicated that walnut has the highest content of antioxidant compounds, particularly tocopherols and polyphenols, and most of the walnut kernel phenolic compounds are concentrated in the pellicle or skin.13,18,46,47 Recently, some valuable constituents such as pyrogallol and juglone (5-hydroxy-1,4-naphthoquinone) have gained considerable attention. Juglone is found in different parts of the walnut tree and shows excellent biological activities, in particular anticancer properties.48,49
3. Walnut fruit
From a nutritional standpoint, the fruit of walnut is the most crucial part of the tree because the seed inside the fruit is generally what is consumed by humans. It is a rounded, single-seeded stone fruit that has four distinct different parts (see Fig. 1 for more details). The green husk or hull is characterized as a thick an external thick layer of walnut fruit. This part is cracked when the fruit ripens fully on the tree. After its separation, the remaining part of the fruit is called the nut.6 The woody and hard material that is the middle portion of the fruit is called the shell. In most walnut cultivars, the shell must be cracked mechanically to obtain the meat.7 The internal portion of the fruit is the kernel or seed, which consumed as the nutritious part of the fruit.50
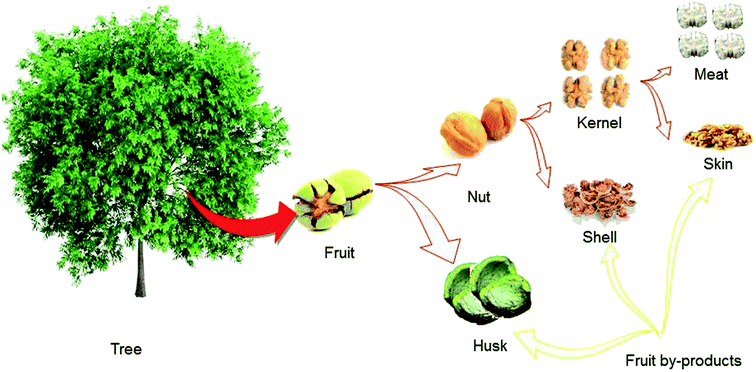 |
| Fig. 1 Different parts of walnut fruit and the corresponding by-products. The walnut fruit mainly consists of the husk, shell, skin, and kernel. The husk, shell and skin, are the main agricultural waste products of walnut fruit. | |
Similar to that in other tree nuts such as almonds, the kernel of the walnut is covered by a thin leathery light brown layer called the skin, seed coat, or pellicle. This part of the walnut fruit has not been comprehensively studied in comparison with those of other tree nuts.30 The most critical and divergent antioxidants are concentrated in this part of the fruit and act as protective agents against harmful UV radiation effects, as well as microbial, fungal, and viral contamination.
4. Walnut by-products
As shown in Fig. 1, during walnut fruit processing to obtain the valuable kernel, large amounts of green husk and woody shell are produced and discarded without being put to any practical use. In some areas, both shells and husks are burned as fuel for heating purposes, which may cause environmental pollution.7 The husk and the shell account for more than 60% of the walnut fruit by weight. The shell makes up a considerable percentage of the fruit by weight (∼40%) and is available as an abundant and low-cost agricultural by-product in walnut production centres. It is crucial point is to reconsider the value of the husk and the shell, not as agricultural waste products to be generated and discharged into the environment during the production of the walnut kernel, but as valuable by-products with applications in the pharmaceutical, nutritional and industrial fields.30
5. Walnut shell
The middle part of the walnut fruit surrounding the kernel is called the shell. It is considered a hard organic abrasive that is chemically inert, nontoxic, and biodegradable. It has multiple advantages, including cost-effectiveness, outstanding durability and elasticity. In addition, it leaves no scratches or pitting during cleaning and is environmentally friendly. Its availability as a renewable resource can be regarded as an additional advantage.51 The shell portion of the walnut fruit is a lignocellulosic agricultural waste composed of cellulose (17.74%), hemicellulose (36.06%), and lignin (36.90%).52
WS is the primary waste material remaining after consumption of the edible part of walnut and has limited applications.53 However, after powdering and sizing, it may be used as a medium in abrasive blasting for polishing and cleaning soft metals, plastics, wood, and stone. It may also be applied in the pre-treatment of some parts and devices before painting as well as for the effective deburring of electrical components, casting, moldings, etc. Furthermore, WS may be used for cleaning and polishing by barrel tumbling, as a filtration medium in hydration systems, as an extender for adhesives materials, as a loss circulation material in the oil industry, and as a filler in casting. The processing of WS enables its use as a scrub in the soap and cosmetic industries and as an abrasive suitable for polishing jewellery, gun casings, and some metal materials.
WS powder has also been found to have excellent adsorbency,54 and it is also an excellent reinforcing material,55 which allows its use as an efficient biosorbent. Considering its unique mechanical properties, low ash content, and bulk density, WS has also been used to produce ACs and to remove HM ions and oils.
Since WS is considered an agricultural waste material, it has no commercial value. Thus, this cellulose-rich agro-waste material is suitable for the development of commercially biodegradable packaging films.56 WS can be used as a carbonaceous sorbent to control various HMs from industrial liquid streams.57 Additionally, it is a suitable medium for crude oil separation from water.51 Finally, WS has some antioxidant compounds such as flavonoids,28 and recently, in addition to ACs some valuable compounds, such as charcoal and pyroligneous acids have been obtained from WS.58
6. Carbon-based materials derived from walnut shell
6.1. Modified walnut shell
Lignin, cellulose, and hemicellulose are the primary components that make up agricultural crop residues. Lignin is known to be a relatively hydrophobic and aromatic natural polymer, whereas cellulose is a typical biopolymer that is present in plant cell walls. Hydroxyl (–OH), carbonyl (–C
O), and carboxyl (–COOH) groups are considered significant polar functional groups in natural sorbents derived from waste crops, and are potentially involved in the bonding of pollutants during the sorption process. Various agricultural waste products are good alternatives to expensive adsorbents because they naturally have large amounts of functional groups on their structure.52 In their natural form, the binding of adsorbates to the adsorbents developed from agricultural waste residues is not significant because of the presence of methyl esters in the chemical constituents. However, the metal-binding potential of the adsorbent ca. be increased by treating the adsorbent with a base or by modifying the methyl esters to –COOH groups. For example, sodium hydroxide (NaOH) transforms cellulose I into cellulose II by penetrating into the structure of cellulose, thus reducing the crystallinity and increasing the porosity and surface area.59 In chemically modified adsorbent, the –OH groups of cellulose macromolecules are more easily accessible, which induces the liberation of new adsorption sites on the adsorbent, in the end, the adsorption capacity of the adsorbent is improved. The untreated form of WS shows a low removal capability and its process kinetics are slow. Thus, chemical modification could be considered to enhance its adsorption capacity. The introduction of chemically functional groups may improve the efficiency of adsorption, for instance, amide (CONH2), –COOH, and –OH groups. Among them, the addition of the –COOH group is the most commonly used procedure.60 The effect of alkali pretreatment on the adsorption capability of WS has been reported. WS modified with citric acid contains phenolic compounds that are abundant in –OH and –COOH groups, which may serve as active sites for the successful removal of different hazardous materials.52
6.2. Activated carbons prepared from walnut shell
ACs are some of the most extensively accepted adsorbents for the purification of gaseous and aqueous solution systems on an industrial scale. ACs are prepared in three main steps: a raw material pretreatment process, a low-temperature carbonization process, and an activation procedure. The carbonized material is activated using two different methods: chemical activation (CA), and physical activation (PA). In the PA process, the raw material is activated after the carbonization of the porous structure developed on the surface and inside of the carbon material. Generally, for the reduction of carbon loss, activation is executed at temperatures between 800 and 1000 °C under anaerobic condition. In the CA method, chemical agents are added to the raw materials and then heated with an inert gas as a protectant. In this preparation method, the carbonization and activation processes progress are simultaneously. CA has some advantages compared to PA, such as a more easily controllable activation reaction, a larger specific surface area (SSA) of the product and a shorter activation time. Currently, it is the dominant process for producing high-performance ACs.61 The price of ACs has increased over time. For this reason, to develop a sustainable method, it is essential to explore new methods for as alternatives to ACs production using non-conventional and low-cost methodologies. All materials with carbonaceous structures can be used for the preparation of ACs, but the raw materials used and the activation process determine the properties of the obtained ACs. Nutshells, fruit pits, paper mill waste (lignin), wood, charcoal, brown and bituminous coals, lignite, bone, and peat are the most commonly used starting materials for the production of ACs.62 Walnut is an ordinarily accepted nutrient food but WS has no commercial value. Consequently, to turn the produced waste shell biomass into a valuable product, WS can be used to prepare ACs. WS has been considered as the raw material for the preparation of ACs via both PA and CA methods. For example, using potassium carbonate (K2CO3) as a chemical activator, ACs were prepared from five different types of nutshell.63 Almond, coconut, oil palm, pistachio, and walnut shells were used as the starting materials. The ACs from all the samples had maximum SSAs at the preparation temperature of 800 °C. The authors found that K2CO3 is a useful activation reagent, but it worked differently at temperatures below 527 and above 27 °C. In addition, Kim, et al.64 reported the use of WS as the raw material for the production of GACs via the CA process. It was shown that the iodine value increased with increasing activation temperature. However, at temperature above 400 °C, the iodine was thermally degraded. The microporous structure of the ACs was also destroyed at activation times longer more than 1 h and at 375 °C. The increasing concentration of the zinc chloride (ZnCl2) solution improved the iodine value. However, it decreased with excessive ZnCl2. The degree of activation by ZnCl2 was compared with that by CaCl2 and a high degree of activation was observed for WS activated by ZnCl2. High contents of carbon and oxygen are associated with the presence of polysaccharides such as lignin, cellulose, and hemicellulose in the WS.65 A high iodine number and specific surface area are reported for the ACs obtained from WS. These properties are associated with the low ash and high lignin contents of the shells and hard shells such as WS have relatively low ash contents.66 Due to high carbon and low ash contents, WS is therefore a suitable material for the production of ACs.
7. Hazardous material removal with walnut shell
7.1. Removal of heavy metals
Due to human activities, natural water resources are polluted by discharged wastewater from public residual and industrial wastewater. Typical industrial wastewater contains poisonous substances such as acids, HMs, and dyes. To preserve water quality, it is essential for industries to treat their effluent before discharging it into the environment.67 Agricultural by-products may be used as green adsorbents to resolve the problems concerning HM pollution, especially that from medium-sized and small industrial facilities.68 Different processes such as ion exchange, electrocoagulation, chemical precipitation, membrane separation, biosorption, and adsorption have been developed to eliminate different metal ions from wastewater before inflow into water for human use.69 Among these processes, adsorption is considered an economical and efficient technology because many adsorbents can be provided by forestry and agricultural residues (normally biomass) with is one method of “dealing with waste by waste”.70 The adsorption of HM ions from the aqueous phase on carbonaceous adsorbents is a diffusion-based process that includes two main steps: first the adsorbate is transferred from the aqueous phase to the external surface of the carbon through the liquid film that surrounds the carbon particle, and second, the HM ions move deeper into the pores and are adsorbed via chemical complexation and/or ion-exchange at the chemically active sites.71,72
At least 20 different HM ions have been determined to be toxic elements,73 and thus, their release into fresh waters could have adverse outcomes for human health and aquatic organisms.74 As a highly available agricultural by-product with a high SSA, excellent chemical stability, high mechanical strength, and easy regeneration, WS has proven effective as an adsorbent in the removal of HMs from wastewater.54,75 Copper (Cu2+), chromium (Cr6+), arsenic (As3+), cadmium (Cd2+), lead (Pb2+), caesium (Cs+), nickel (Ni2+), zinc (Zn2+), manganese (Mn2+), iron (Fe2+), and mercury (Hg2+) are 11 HM ions that are considered to be removable by WS. The optimized operational conditions, including solution pH, adsorbent dosage, temperature, agitation speed, and contact time are the main parameters considered in identifying the maximum removal efficiency (%) for HM ion removal by adsorption by WSACs (Table 1). Additionally, the corresponding maximum adsorption capacity (qm), thermodynamics of the adsorption reaction, and fitting and kinetic models, are summarized in Table 2. Further discussion regarding each HM ion is presented in the following sections.
Table 1 The optimal conditions for the removal of various HM ions by WSa
No |
HM ions |
pH |
Adsorbent dosage |
Temp. (°C) |
Agitation speed (rpm) |
Contact time (min) |
Efficiency (%) |
Ref. |
— not reported. Modified WS. Ordinary WS. |
1 |
Cu2+ |
— |
0.3 g l−1b |
30 |
Shaking |
30 |
63 |
Kim, et al.64 |
5.6 |
1 g l−1b |
40 |
180 |
60 |
— |
Xie, et al.76 |
7 |
2 g l−1c |
Room temp. |
Shaking |
80 |
90 |
Feizi and Jalali77 |
7 |
0.1 gb |
Ambient temp. |
— |
— |
97.5 |
Kazemipour, et al.78 |
2 |
Cr6+ |
2 |
2 g l−1b |
40 |
200 |
— |
99 |
Ghasemi, et al.79 |
5 |
1 g l−1b |
40 |
180 |
60 |
— |
Xie, et al.76 |
2 |
1.5 g/0.15 lb |
27 |
150 |
1440 |
— |
Nethaji and Sivasamy80 |
3.5 |
0.5 g/0.02 lc |
25 ± 1 |
200 |
100 |
85.32 |
Pehlivan and Altun75 |
2 |
0.1 g/0.02 lb |
25 ± 1 |
Shaking |
120 |
75 |
Altun and Pehlivan52 |
1 |
0.80 gb |
35 |
200 |
120 |
99.4 |
Lu, et al.81 |
3 |
As3+ |
5.5 |
0.03 g l−1b |
24 |
120, 90 |
420, 1440 |
— |
Jafari-Mansoorian, et al.82 |
10 |
40 g l−1b |
20 |
60 |
40 |
88 |
Saqib, et al.83 |
4 |
Cd2+ |
6 |
0.2 g l−1b |
30 |
150 |
240 |
41.96 |
Gondhalekar and Shukla84 |
6–10 |
0.1 gb |
Ambient temp. |
— |
— |
50.9 |
Kazemipour, et al.78 |
7 |
2 g l−1c |
Room temp. |
Shaking |
200 |
90 |
Feizi and Jalali77 |
5 |
20 g l−1b |
25 |
150 |
30 |
∼90 |
Almasi, et al.85 |
6.92 |
0.56 g l−1b |
25 |
Shaking |
40.42 |
99.72 |
Saffari86 |
5 |
Pb2+ |
5 |
0.18 g l−1b |
35 |
— |
— |
89.61 |
Cheng, et al.60 |
6–10 |
0.1 gb |
Ambient temp. |
— |
— |
96 |
Kazemipour, et al.78 |
5.5 |
2 g l−1b |
25 |
140 |
150 |
94.12 |
Yi, et al.87 |
4.5 |
0.5 g/0.05 lb |
25 |
Shaking |
14 days |
100 |
Moreno-Barbosa, et al.88 |
5 |
1.0 g l−1b |
45 |
65 |
400 |
99 |
Saadat, et al.89 |
6.3 |
13.5 g l−1b |
— |
65 |
300 |
98.2 |
Saadat and Karimi-Jashni90 |
5 |
20 g l−1b |
25 |
150 |
30 |
∼90 |
Almasi, et al.85 |
4 |
5 g l−1c |
25 |
120 |
60 |
62.9–83.7 |
Gala and Sanak-Rydlewska91 |
6 |
Cs+ |
— |
20 g l−1b |
Room temp. |
200 |
2880 |
— |
Ding, et al.54 |
4 |
15 g l−1b |
25 ± 1 |
200 |
1440 |
99.8 |
Ding, et al.92 |
7 |
Ni2+ |
8 |
10 g l−1b |
45 |
65 |
300 |
88.3 |
Karimi-Jashni and Saadat59 |
6 |
2 g l−1b,c |
Room temp. |
Shaking |
120 |
90 |
Feizi and Jalali77 |
8 |
Zn2+ |
8 |
2 g l−1c |
Room temp. |
Shaking |
200 |
90 |
Feizi and Jalali77 |
6–10 |
0.1 gb |
Ambient temp. |
— |
— |
71 |
Kazemipour, et al.78 |
4.5 |
0.5 g/0.05 lb |
25 |
Shaking |
14 days |
98.4 |
Moreno-Barbosa, et al.88 |
9 |
Mn2+ |
8 |
2 g l−1c |
Room temp. |
Shaking |
200 |
96.5 |
Feizi and Jalali77 |
10 |
Fe2+ |
8 |
2 g l−1c |
Room temp. |
Shaking |
20 |
90 |
Feizi and Jalali77 |
4.5 |
2 g l−1b |
40 |
200 |
— |
96.2 |
Ghasemi, et al.79 |
11 |
Hg2+ |
5 |
0.02 g/0.05 lb |
25 |
720 |
60 |
90 |
Zabihi, et al.93 |
5 |
0.05 gb |
29 |
720 |
60 |
— |
Zabihi, et al.57 |
Table 2 The adsorption capacity, thermodynamic, fitting and kinetic models for the removal of different HM ions by the WSa
No |
HM ions |
qmb |
Fitting model |
Kinetic model |
Thermodynamic |
Ref. |
— not reported. Maximum adsorption capacity. Modified WS. Ordinary WS. |
1 |
Cu2+ |
30 mg l−1c |
Freundlich |
— |
Not spontaneous, exothermic |
Kim, et al.64 |
204.08 mg g−1c |
Langmuir |
Pseudo-second order |
Spontaneous, endothermic |
Xie, et al.76 |
38.8 mg g−1d |
Langmuir |
Pseudo-second order |
— |
Feizi and Jalali77 |
— |
— |
— |
— |
Kazemipour, et al.78 |
2 |
Cr6+ |
43.12 mg g−1c |
Langmuir–Freundlich |
Pseudo-second order |
Spontaneous, endothermic |
Ghasemi, et al.79 |
51.28 mg g−1c |
Langmuir |
Pseudo-second order |
Spontaneous, endothermic |
Xie, et al.76 |
6.01 mg g−1c |
Langmuir |
Pseudo-second order |
Spontaneous, endothermic |
Nethaji and Sivasamy80 |
8.01 mg g−1d |
Langmuir |
— |
— |
Pehlivan and Altun75 |
154 mg g−1d |
Langmuir |
— |
Spontaneous, endothermic |
Altun and Pehlivan52 |
596 mg g−1c |
3.5 mg g−1c |
Langmuir |
Pseudo-second order |
— |
Lu, et al.81 |
1 mg g−1d |
3 |
As3+ |
0.2 mg l−1c |
Langmuir, Freundlich |
— |
— |
Saqib, et al.83 |
3.42 mg g−1c |
Langmuir |
— |
— |
Jafari-Mansoorian, et al.82 |
4 |
Cd2+ |
14.29 mg g−1c |
Langmuir |
Pseudo-second order |
Spontaneous, exothermic |
Gondhalekar and Shukla84 |
— |
— |
— |
— |
Kazemipour, et al.78 |
76.9 mg g−1d |
Langmuir |
Pseudo-second order |
— |
Feizi and Jalali77 |
11.6 g kg−1c |
Langmuir |
Pseudo-second order |
— |
Almasi, et al.85 |
70.78 mg l−1c |
— |
— |
— |
Saffari86 |
5 |
Pb2+ |
210.14 mg g−1c |
Langmuir |
— |
Spontaneous, endothermic |
Cheng, et al.60 |
— |
— |
— |
— |
Kazemipour, et al.78 |
81.96 mg g−1c |
Langmuir |
Pseudo-first order |
— |
Yi, et al.87 |
32.362 mg g−1c |
Langmuir |
— |
— |
Moreno-Barbosa, et al.88 |
294.10 mg g−1c |
Langmuir |
Pseudo-second order |
— |
Saadat, et al.89 |
— |
— |
— |
— |
Saadat and Karimi-Jashni90 |
32 g kg−1c |
Langmuir |
Pseudo-second order |
|
Almasi, et al.85 |
23.1 mg g−1d |
Langmuir–Freundlich |
— |
— |
Gala and Sanak-Rydlewska91 |
6 |
Cs+ |
14.6 μmolc |
Freundlich |
Pseudo-second order |
Spontaneous, endothermic |
Ding, et al.54 |
6 ± 4.3c |
Langmuir |
Pseudo-second order |
— |
Ding, et al.92 |
7 |
Ni2+ |
8.57 mg g−1c |
Langmuir |
Pseudo-second order |
Spontaneous |
Karimi-Jashni and Saadat59 |
29.4 mg g−1d |
Langmuir |
Pseudo-second order |
— |
Feizi and Jalali77 |
38.9 mg g−1c |
8 |
Zn2+ |
33.3 mg g−1d |
Langmuir |
Pseudo-second order |
— |
Feizi and Jalali77 |
— |
— |
— |
— |
Kazemipour, et al.78 |
6.079 mg g−1c |
Langmuir |
— |
— |
Moreno-Barbosa, et al.88 |
9 |
Mn2+ |
28.6 mg g−1d |
Langmuir |
Pseudo-second order |
— |
Feizi and Jalali77 |
10 |
Fe2+ |
62.6 mg g−1d |
Langmuir |
Pseudo-second order |
— |
Feizi and Jalali77 |
39.25 mg g−1c |
Langmuir, Freundlich |
Pseudo-second order |
Spontaneous, endothermic |
Ghasemi, et al.79 |
11 |
Hg2+ |
151.5 mg g−1c |
Langmuir, Freundlich |
Pseudo-second order |
— |
Zabihi, et al.93 |
151.5 mg g−1c |
Langmuir, Freundlich |
Pseudo-second order |
Endothermic |
Zabihi, et al.57 |
7.1.1. Zinc ions removal. Zn2+ is one of the most significant elements that is frequently detected in effluents released from manufacturing facilities in association with acid mine effluent, galvanization factories, natural minerals, and municipal wastewater processing plants. The World Health Organization (WHO) set the highest tolerable concentration in drinking water of Zn2+ as 5.0 mg l−1.94 Zn2+ is non-biodegradable and is bioaccumulated throughout the food chain, thus, its removal is of great importance. In a batch model study performed by Moreno-Barbosa, et al.,88 they attempted to remove Pb2+ and Zn2+ ions from solution through adsorption onto synthesized watermelon shell activated carbons (WMSACs) and walnut shell activated carbons (WSACs) in a CA process using phosphoric acid (H3PO4) 40%. The production yields were 85 and 80% for the WMSACs and WSACs, respectively. The surface area and pore volume of WSACs were 10 and 13% higher than those of the WMSACs, respectively. Additionally, surface areas of 789 m2 g−1 and 710 m2 g−1 were reported for the WSACs and WMSACs, respectively. Moreover, the WSACs demonstrated a better resistance to high temperatures than the WMSACs. Likewise, the adsorption isotherm data were better fitted by the Langmuir model. According to the experimental results, 1 g of WMSACs adsorbed >1 mg of Pb2+ and Zn2+ more than 1 g of WSACs. This higher adsorption potential indicated the importance of pore distribution in the adsorption system, as WMSACs have a mesoporous and microporous composition, while WSACs only contain micropores. In addition, surface chemistry was reported to be an important variable in the adsorption process as the WMSACs had a lower point of zero charge pH (pHPZC) than the WSACs (3.05 for WMSACs and 4.5 for WSACs) and the pH of each metal solution was adjusted in 4.5. This suggests that the electrostatic interactions between the ion and the carbon surface had increased. Further findings reported that orange peel, hazelnut shell, and WS can be appropriately employed as low-cost adsorbents for Zn2+ and Cd2+ removal from water, and their highest sorption abilities were 15.51 and 19.8 mg g−1, 11.55 and 16.65 mg g−1, and 26.60 and 21.10 mg g−1, respectively. In addition, Zn2+ and Cd2+ ion biosorption from aqueous solution to organic wastes such as orange peel, hazelnut shell, and WS were investigated using batch adsorption studies.95 Hazelnut shells had the highest removal efficiency for Zn2+ and Cd2+ ions. The method was reported to be quick and all the studied biosorbents were capable of removing 90% of HM ions. The sorption process can be performed on a heterogeneous surface with chemisorption. The raw material WS was also employed as regional agricultural biomass in Yasouj city, Iran. for the preparation of ACs.96 Using the Taguchi experimental design, the optimum condition of the synthesis process was obtained and the adsorption ability of the generated ACs towards Zn2+ was considered as a response. The activation time and the impregnation ratio were found to be effective parameters for defining the optimum conditions, according to the data analysis. The ACs developed under optimum conditions had a mesopore structure with an SSA of 1388 m2 g−1. The adsorption capacity of the produced carbon for Zn2+ was 89 mg g−1. Additionally, the composite biosorbents obtained from WS and snail shells were used to study the biosorption process of Zn2+ ions in industrial wastewater.97 Composite adsorbents were provided by activating WS with H3PO4 to achieve acid-treated WS (AWS). Then, WS and AWS were separately impregnated on chitosan (CS) to prepare WS impregnated on CS (WSCS) and acid-treated WS impregnated on CS (AWSCS). A pH of 5, 1 g adsorbent dosage, Zn2+ ion initial concentration of 30 mg l−1, contact time of 2 h, agitation speed of 150 rpm, particle size of 60 BSS and temperature of 30 °C resulted in the greatest adsorption capabilities 3.1104, 3.8052, 16.4474 and 17.6991 mg g−1 for WS, AWS, WSCS and AWSCS, respectively. Among the investigated kinetic models, the pseudo-second-order kinetic model gave the best fitting model. Zn2+ ions adsorption on the prepared adsorbents was film diffusion-controlled. According to the experimental results from this work, AWSCS has the high potential to be regarded as an alternative effective and inexpensive biosorbent in the remediation of HMs in wastewater. Zn2+ ion adsorption on the derived composite biosorbents was exothermic, endogenic, favourable, and non-spontaneous. Based on the qm values presented in Table 2, WS adsorption capacity for Zn2+ is not sufficient with only 6–33.3 mg g−1 scavenging potential.
7.1.2. Iron ion removal. Of the HMs, Fe2+ is an essential element in haemoglobin for carrying oxygen molecules in the blood circulatory system. However, it may cause adverse effects at high concentrations, and thus, excess amounts of Fe2+ must be removed from the environmental effectively. In comprehensive research by conducting batch experiments, the residues of sunflower, potato, canola, and WS were used as sorbents to sorb different HMs (Fe2+, Mn2+, Zn2+, Ni2+, Cu2+, and Cd2+) from aqueous solution. The highest sorption of HMs by the various sorbents was observed at approximately pH 4–8 and the optimal time for reaching equilibrium was in the range of 20–600 min. The authors observed that the ability of sorbents to sorb of HMs was as follows: sunflower residue > potato residue > canola residue > WS residue. The order of metal sorption in the competitive system for the WS was reported as Ni2+ < Cu2+ < Zn2+ < Mn2+ < Cd2+ < Fe2+. The Langmuir isotherm model fit the sorption results well. The kinetics of HM sorption followed pseudo-second-order kinetic. In this study, electrostatic interaction and ion exchange, as well as complexation were reported to be the main sorption mechanisms in the adsorption of the studied HMs.77WS is reported to be a suitable material for biochar development. Walnut shell biochar (WSB) is also recommended as a green reducing agent to reduce the iron phase in waste copper slag and to clean up hazardous waste. Biochar has been used as a reducing agent in research to reduce waste copper slag for iron recovery. The reduction of copper slag by WSB was theoretically evaluated, and the iron in the slag was effectively retrieved through reduction and magnetic separation techniques.98 As presented in Table 2, the qm values achieved for the removed Fe2+ ions (40–63 mg g−1) indicated that WS could be considered a suitable biosorbent for the separation of this HM from contaminated wastewater.
7.1.3. Nickel ion removal. At relatively low levels, Ni2+ is poisonous and cancerous. Ni2+ is resistant to degradation and accumulates in living organisms, causing respiratory failure, embolisms, lung cancer, pulmonary fibrosis, asthma, chronic bronchitis, congenital disabilities, heart disorders, allergic reactions and renal oedema. The maximum permitted Ni2+ discharge concentration is 2 mg l−1. A practical and cost-effective technology is therefore required to remove Ni2+ from aqueous solution.99 Using pre-treated WS, univariate studies with a factorial design were employed to study the different factors that influence Ni2+ removal.59 The pH of the solution was noted to be the most critical parameter in Ni2+ removal. The highest pretreated WS adsorption capability for Ni2+ was 8.57 mg g−1. The adsorption mechanism of Ni2+ on pre-treated WS obeyed pseudo-second-order kinetics. In another study, different agricultural residues of sunflower, potato, canola, and WS were used as sorbents to sorb HMs including Fe2+, Mn2+, Zn2+, Ni2+, Cu2+, and Cd2+. The plant residues showed considerable capacity for the removal of Fe2+, Mn2+, Zn2+, Cu2+, and Cd2+ from aqueous solution, but the Ni2+ sorption by residues was negligible. The authors improved the capability of natural sorbents to remove Ni2+ from aqueous solution by using 0.5 M sodium hydroxide (NaOH) as the chemical activation agent.77 The removal of Ni2+ ions from aqueous solutions containing 100 mg l−1 of Ni2+ using WS has also been described.100 At a pH of 5.85 with a 5 min contact time for a 5 mg l−1 solid-to-liquid ratio and an initial HM concentration of 100 mg l−1, a maximum removal efficiency of 43.23% was achieved. Moreover, the effects of pH, contact time, initial metal concentration, adsorbent concentration, and co-ions in synthetic solutions and mining waste leachate were considered for the removal of Zn2+, Cd2+ and Ni2+ using WS.101 Metal adsorption was found to be dependent on the solution's initial pH; since the adsorption rate improved as the pH increased, demonstrating the highest affinity at pH 5–7. Additionally, the quantity of the removed metal increased as the initial concentration increased. Increasing the adsorbent dosage increased the removal, but reduced the quantity adsorbed per WS unit mass. The presence of co-ions existence reduced HM adsorption, with divalent ions having a more adverse impact than monovalent ions. Zn2+, Cd2+ and Ni2+ adsorption on WS was reported to match the Langmuir and Freundlich isotherms. According to the qm values obtained in some research (Table 2), only 8–38 mg g−1 of Ni2+ is a removable by WS, which is a considerable the amount compared to the amount of other HMs removed by WS.
7.1.4. Copper ion removal. As an important wastewater contaminant, Cu2+ ions are principally discharged from plating manufactures, mines, and metal cleaning, chemical production, and metal-processing facilities. Cu2+ is considered a non-biodegradable pollutant and is a very toxic HM. It can lead to severe damage in the human body through bioaccumulation.102 The results of several investigations show that adsorption is regarded as the most useful technique for removal of HMs such as Cu2+ ions. A study Kazemipour, et al.78 reported the successful removal of Pb2+, Cd2+, Zn2+, and Cu2+ from industrial wastewater onto the carbon produced from walnut, hazelnut, almond, and pistachio shells as well as apricot stones. In this work, to achieve maximum removal efficiency, the heating time and temperature were optimized at 15 min and 800 °C, respectively. Maximum removal occured at pH 6–10, a 3 ml min−1 flow rate, and an adsorbent dosage of 0.1 g. In another investigation reported by Kim et al.,64 they characterized the adsorption capacities of the prepared GACs developed from WS through CA by using ZnCl2 as an activating agent. The ACs were tested as adsorbents in synthetic wastewater containing Cu2+ ions and the Freundlich model interpreted well the adsorption of Cu2+ ions well. It was suggested that the adsorption potential of the manufactured ACs reached commercialization quality since it was determined to be better than that obtained from coconut shells. Furthermore, in another work published by Xie et al.,76 they developed acid and acid–base modified WSACs and carried out batch experiments to study their adsorption behaviours towards Cu2+ and Cr6+. In this effort, the kinetics data matched excellently with pseudo-second-order kinetics. The results showed that the acid–base-prepared ACs presented adequate Cu2+ adsorption capacity, with a highest adsorption potential of 204.08 mg g−1. Additionally, it was suggested that Cu2+ adsorption on acid–base ACs was a chemical ion-exchange procedure. Thermodynamically, Cu2+ adsorption was spontaneous and endothermic. The authors concluded that the pH and the surface chemistry of the ACs, rather than the carbon composition properties, had a critical function in the uptake of the Cu2+ ions from aqueous solution, while the Cr6+ adsorption was closely associated with the pH and textural characteristics.Ultrasound technology is currently applied in various ways in different fields. The effects of ultrasound technology (UST) on the adsorption process of Cu2+ into GACs obtained from WS were recently studied. The GAC adsorption rates of 1.7–3.86 mmol g−1 Cu2+ using UST, and 0.66 to 2.7 mmol g−1 Cu2+ without UST were reported. Furthermore, the adsorption efficiency was enhanced with increases in the initial concentration and SSA.103 Ultrafine water mist (UWM)-plasma alteration is a new approach for promoting the adsorption potential of raw materials. It has been suggested that nonthermal plasma in UWM can enhance the content of –COOH groups on the surface of raw WS which increases its efficiency in the extraction of Cu2+ from wastewater.104 Characterization studies using techniques including Brunner–Emmett–Teller (BET) measurements, scanning electron microscopy-/energy-dispersive X-ray analysis (SEM-EDX), and X-ray photoelectron spectroscopy (XPS) showed that more –COOH groups were created on the surface of modified WS (MWS). In the introduced technology, the disassociated oxygen atoms in water mists with plasma bonded to the WS and formed active sites containing –COOH groups. After the chemisorption of Cu2+ by the –COOH groups, the COOH groups' content in the WS diminished because several groups were changed into –C
O groups. For the raw WS, the efficiency of Cu2+ removal was 33.5%; however, after plasma modification for 15 min under 3 g min−1 water mist, the efficiency increased considerably (98%). The highest Cu2+ adsorption potential of the UWM-plasma-modified WS was 39.4 mg g−1 at pH 5.3 and 25 °C, approximately 8 times higher than that of the raw WS. Based on the qm values obtained in different studies for the removal of Cu2+ ions (see Table 2 for more details), the WS adsorption capacity for such metal ions is in the range of 30–204 mg g−1. This adsorption capacity is reasonable for the practical application of WS when compared to its adsorption of other HMs such as Ni2+, Zn2+ and Mn2+.
7.1.5. Chromium ion removal. Cr6+ ion is another hazardous HM ion, and its removal using WS has been reported in several investigations. For example, an attempt to remove Cr6+ from an aqueous solution using ACs derived from WS biomass through alkali impregnation with NaOH.80 The authors impregnated WS with NaOH, and different ACs were created by varying the impregnation ratio of char: NaOH to 1
:
1 (AC1), 1
:
3 (AC2), and 1
:
5 (AC3). The adsorption of Cr6+ onto the ACs fit well with the Langmuir isotherm model, and the data followed the pseudo-second-order kinetic model. Film diffusion and intraparticle diffusion were the main mechanisms of the adsorption reaction. The ACs with the highest impregnation ratio (AC3) had better adsorption characteristics compared to AC2 and AC1. Ghasemi et al.79 synthesized highly effective ACs from WS through a two-step CA process and thermal pyrolysis using ZnCl2 to facilitate Cr6+ and Fe2+ removal from an aqueous solution and studied their single and binary solute adsorption. It was demonstrated that the developed ACs had a porous structure with excellent textural characteristics such as high BET surface area (1223 m2 g−1) and high total pore volume (0.85 cm3 g−1). The results of adsorption experiments showed that at both pH levels of 4.5 and 2, the maximum removals were 96.2 and 99% at 40 °C for Fe2+ and Cr6+ ions, respectively. Conclusively, it was claimed that under similar conditions, the developed WSACs showed better adsorption performance than other ACs.Other studies reported Cr6+ adsorption in water using WS modified by H3PO4.81 Cr6+ ion biosorption from aqueous solution was also investigated in batch experiments with the use of shells from walnut, hazelnut, and almond shell.75 In this study, kinetic experiments showed that the dilute Cr6+ solutions attained equilibrium in 100 min. The biosorption potential of all the investigated shells was dependent on the pH which was optimized at 3.5. The adsorption data suited the Langmuir isotherm model well. The maximum sorption capacity conformed to the Langmuir isotherm and was reported to be 8.01 mg g−1 for WS. At a concentration of 0.5 mM, removal percentage by WS was obtained to be 85.32%. The same research group,52 used MWS as an adsorbent to remove Cr6+ ions from aqueous solution after treatment with citric acid in batch experiments. The rate of adsorption was studied under various conditions, including different amounts of adsorbent (0.02–0.20 g), pH (2–9), contact times (10–240 min), temperatures and initial Cr6+ concentrations (0.1–1.0 mM). In all cases, Cr6+ adsorption was pH-dependent and for the citric acid modified walnut shell (CAMWS), a maximum equilibrium was obtained at pH values between 2 and 3. Under experimental conditions, the highest adsorption capabilities of CAMWS and untreated WS for Cr6+ ions were 596 and 154 mg g−1, respectively. The column studies performed under different operating conditions showed that WS is an efficient and cost-effective adsorbent for Cr6+ removal and can be a solution for industries that discharge Cr6+. In this study, better adsorption results were obtained with low influent flow rate, a low influent Cr6+ concentration, and a high bed depth. The Cr6+ adsorption equilibrium (Langmuir isotherm constant = 0.6754 l mg−1) fit well with the Langmuir isotherm model.105 According to the qm values reported in the literature (Table 2), WS and in particular MWS have high potential to be used as an effective biosorbent for Cr6+ ion elimination. Additionally, of all the examined HMs, WS has the highest adsorption capacity for Cr6+ (in the range of 1–596 mg g−1).
7.1.6. Arsenic ions removal. As3+ is an HM that is considered a toxic pollutant because of the hazard it poses to both human health and the environment. One of the most popular methods for As3+ removal from an aqueous solution is the adsorption process. For example, Jafari-Mansoorian et al.,82 evaluated As3+ adsorption from aqueous solutions using ACs derived from WS. According to the Langmuir model, a qm of 3.15 mg g−1 was achieved using the WSACs. Additionally, the qm of the provided WSACs was reported to be 2.05 mg g−1 by the Freundlich model. The results of this work showed that an increase in the adsorbent dosage and a reduction in the pH improved As3+ adsorption. Adsorption decreased with increasing pH and initial As3+ concentration. In another study, Saqib et al.83 studied As3+ bioremediation from aqueous solutions by low-cost materials derived from blue pine wood shavings (Pinus wallichiana), WS, and chickpea testa. It has been shown that blue pine wood shavings have great potential as a remediation substance for the removal of As3+. Considerable biosorption capacity (88%) was also achieved for WS. However, the biosorption capacity of chickpea testa was not sufficient. As3+ biosorption in this study fit well to both the Langmuir and Freundlich isotherms. In total, the results of the adsorption studies summarized in Table 2 indicate tha WS could only adsorb low amounts of As3+ ions from contaminated aqueous solutions (0.2–3.42 mg g−1).
7.1.7. Caesium ion removal. Due to its relatively long half-life and varied sources, Cs+ an abundant element that is thought to be very dangerous. Because of its similar chemical properties to potassium ion (K+), it can easily be taken up by aquatic and terrestrial organisms.106 Thus, its separation and elimination from waste solutions is necessary. Ding et al.,54 conducted equilibrium, kinetic, and thermodynamic modelling studies of Cs+ adsorption from aqueous solution using WS. They incorporated nickel hexacyanoferrate (NiHCF) into the prepared biosorbent, which served as a high selectivity trapping agent for Cs+. It was indicated that the adsorption of Cs+ by NiHCF-WS is an endothermic and spontaneous process. In another investigation by the same research group,92 the prepared novel NiHCF functionalized-WS was developed to enable the selective removal of Cs+ ions from aqueous solution. Their results showed that the removal of Cs+ was fast and reached equilibrium in 2 h. Acidic conditions were favoured for Cs+ removal because of the unique features of NiHCF-WS. Additionally, the fabricated NiHCF-WS could selectively eliminate Cs+ in the presence of other ions (Na+ and K+). Using NiHCF-WS, it was demonstrated that approximately 99.8% of the liquid waste was reduced. Based on the qm values (see Table 2 for more details) extracted from the Cs+ ion adsorption studies, the WS adsorption capacity of Cs+ ions is not significant (6–14.6 mg g−1), thus, additional treatment procedures should be tested to enhanced its adsorption capacity.
7.1.8. Mercury ion removal. Hg2+ is one of the key pollutants on the United States Environmental Protection Agency (US EPA) pollutants list, as it can easily pass the blood–brain barrier and influence the brains of foetuses. High Hg2+ concentrations trigger lung and kidney function impairment, chest pain and dyspnoea. The reasonable Hg2+ discharge thresholds for inland and drinking water are 10 and 1 μg l−1, respectively. While the discharge of Hg2+ into aquatic systems has decreased in recent years, there is still a shortage of efficient, inexpensive means to treat wastewater that contains Hg2+.107 In pursuit of producing a more economical and effective carbonaceous sorbent to enable the control of Hg2+ ions from industrial liquid streams, Zabihi et al.93 evaluated the adsorption ability of a powdered carbonaceous sorbent derived from the Iranian WS and prepared by CA method using ZnCl2 as an activating reagent. This adsorbent had not been previously used to remove Hg2+ from water to the best of the authors' knowledge. Hg2+ adsorption from aqueous solution was performed under various experimental conditions such as different treatment, times, concentrations of metal ions, adsorbent quantities, pH values and solution temperatures. Hg2+ uptake was shown to decrease with increasing solution pH. The selection of the proper procedure resulted in the preparation of a microporous AC with 0.45 g cm−3 density, 737 mg g−1 iodine number, and 780 m2 g−1 BET surface area. The optimum monolayer sorption capability of the adsorbent was 151.5 mg g−1. The same research group,57 studied the adsorption of Hg2+ ions from industrial liquid streams on ACs prepared from WS. In this study, carbonaceous sorbents derived from the local WS were also prepared by the CA process with ZnCl2. Microporous ACs with distinct BET values of 780 (ACs A, 1
:
0.5 ZnCl2) and 803 (ACs B, 1
:
1 ZnCl2) m2 g−1 were the result of an appropriate selection of preparation processes. For ACs A and B, the monolayer adsorption capacities of the adsorbents were 151.5 and 100.9 mg g−1, respectively. Based on the corresponding qm values shown in Table 2, it can be concluded, that compared the adsorption potential of WS for other HMs such as Cr2+ and Pb2+, the adsorption potential of WS of the removal of Hg metal ions is moderate (151 mg g−1).
7.1.9. Lead ion removal. Pb2+, a well-known prestigious HM, poses a significant danger to organisms and the environment; in the recent years, Pb2+ removal has attracted increasing attention. The release of large amounts of Pb2+' into terrestrial and aquatic ecosystems has raised public concern. Because of its toxicity, accumulation in food chains and persistence in nature, Pb2+ can threaten human lives. It is a strong metabolic poison and enzyme inhibitor and can accumulate in the brain, bones, muscles, and kidneys. The WHO recommends that the highest tolerable concentration of Pb2+ in drinking water is 0.1–0.05 mg l−1.108 Thus, as a toxic and hazardous metal ion, its removal by WS via adsorption has been comprehensively studied. Using single-walled carbon nanotubes (SWCNTs) Saadat et al.,89 synthesized a novel SWCNT-doped walnut shell composite (SWCNTs/WSC), and characterized its ability to adsorb Pb2+ from aqueous solution. First, they determined the Pb2+ removal capacity of different synthesized carbon nanostructures, including SWCNTs, multiwalled carbon nanotubes (MWCNTs), SWCNTs doped with iron (SWCNTs/Fe), and carbon nanofibres (CNFs). The SWCNTs had highest sorption capability for Pb2+ ions. Further, the authors prepared a novel adsorbent, SWCNTs/WSC, with 99% through the immobilization of SWCNT particles on the WS surface. The developed adsorbent was tested for its potential to remove Pb2+ ions from aqueous solution. The prepared SWCNTs/WSC showed effective removal of Pb2+ ions from aqueous solution. The adsorption followed the equation of the Langmuir isotherm well, with an expected maximum uptake of 185.2 mg g−1. The removal of Pb2+ by adsorption onto Chinese WSACs was also explored,87 and the results showed that they might be successfully applied to remove Pb2+ from wastewater. By varying the contact time, temperature, pH, adsorbent dose and initial concentration of Pb2+, the authors performed batch experiments, and the adsorption equilibrium was obtained within 150 min. Although the effect of temperature was not notable, the adsorption of Pb2+ was mostly pH-dependent, and the greatest elimination was obtained at pH 5.5. The efficiency of Pb2+ removal was improved by increasing the dosage of ACs to 2.0 g l−1 which produced a maximum removal potential of 94.12%. The findings proposed that the Langmuir isotherm model with a qm of 81.96 mg g−1 described the equilibrium conditions well. The results showed that the adsorption kinetic data were described well by the pseudo-first-order model. Saadat and Karimi-Jashni90 used a factorial design and simplex methodologies to analyse the separation of Pb2+ ions and the optimized Pb2+ adsorption onto the MWS. They attempted to remove Pb2+ ions from aqueous solution using chemically modified WS in a batch system. The adsorbent dosage of 13.5 g l−1, solution pH of 6.3, and initial metal concentration of 45.3 mg l−1, with an efficiency of 98.2% were recommended as the optimum conditions for Pb2+ removal. A comparative study of Pb2+ sorption from aqueous solution onto WS and plum stones was also performed, and the maximum sorption capacity obtained for WS was 23.1 mg g−1. The Pb2+ depletion percentage in the solution was reported to be in the range of 62.9–83.7.91 The adsorption equilibrium and the kinetics of Pb2+ removal from aqueous solution by MWS with acrylic acid were also investigated in the temperature range of 15–35 °C.60 MWS samples with distinct graft yields (3.65, 10.45, 18.49, and 19.66 wt%) were prepared by reactions with acrylic acid and WS. The effects of the adsorbent dose, graft yield, contact time, temperature, and pH on the adsorption of Pb2+ were analysed. Both WS and MWS-4 (19.66 wt%) were characterized, and the Langmuir isotherm model interpreted the isotherm adsorption data better. The maximum adsorption capacity obtained for Pb2+ was 210.14 mg g−1 at 35 °C.In very recent the work, a novel carboxylate-functionalized walnut shell (CFWS) has been prepared with malonate through the esterification of WS.109 The characterization of CFWS characterization by various methods suggested the introduction of –COOH groups on the WS surface. The efficiency of the modified adsorbent at removing Pb2+ ions from aqueous solutions. The investigation data showed that the Langmuir isotherm could thoroughly describe the equilibrium data, and the best adsorption ability for Pb2+ ions was determined to be 192.3 mg g−1 with 0.8 g l−1 of the adsorbent, pH 5.5, and a temperature of 25 °C. Under various experimental conditions, two methods, namely multiple linear regression (MLR) and an artificial neural network (ANN), were adopted to build a practical model for the prediction of the removal of Pb2+ ions. A comparison between the created models demonstrated that the ANN model could more correctly predict the percentage of Pb2+ ions removal. Therefore, an automated wastewater remediation plan could be designed using this ANN model. It should also be noted that the CFWS was obtained from aqueous solution using EDTA-2Na and used to remove Pb2+ ions. Additionally, in a newly published study, an amino-modified walnut shell (AMWS) was developed as an environmentally friendly, green, easy-to-use and sustainable HM trapping agent and its adsorption of Pb2+ ions in aqueous solution was studied.110 The adsorption ability of AMWS for Pb2+ was examined in pH 2–9 and various doses of adsorbents (0.5–2.0 g l−1). The results showed that at pH 7, the qm of AMWS on Pb2+ ions was reasonable. The highest predicted adsorption capacity of Pb2+ ions was achieved with the Langmuir fitting model at 25 °C (56.81 mg g−1). The pseudo-second-order equation represented the adsorption kinetics well. Thermodynamic investigations confirmed that the adsorption process of AMWS for Pb2+ ions was endothermic and spontaneous. In addition, the results showed that AMWS possessed remarkable recovery capacity. In Lu and Guo,111 a low-cost biosorbent for the removal of Pb2+ in aqueous solution was synthesized by grafting sulfur-functionalized WS (SFWS) using xanthate. The synthesized adsorbent was characterized by XPS, Fourier-transform infrared spectroscopy (FT-IR), BET and SEM. This study showed that the adsorption efficiency of SFWS was significantly higher than that of unmodified WS due to ion exchange and complexation by the sulfur-containing functional groups. The adsorption process was exothermic and spontaneous. Additionally, it was found that the Pb2+ adsorption on SFWS followed the Temkin isotherm model which had a high correlation with pseudo-second-order kinetics. Ashrafi, et al.112 investigated WS modification using a solvent-free reaction in which WS was blended with malonic acid cyclic isopropylidene ester, forming carboxylic acid-functionalized WS. The ability of the modified adsorbent simultaneously eliminate Pb2+ and methylene blue (MB) was considered. A high removal percentage, 97.14%, was obtained under the optimum experimental conditions. The FT-IR spectra showed that electrostatic interactions were the main forces driving Pb2+ adsorption.
In another technique, carbon microspheres are produced under N2 flux from WS and then applied as an adsorbent for the elimination of Pb2+, Cu2+, Cr3+ and Cd2+ ions.113 The prepared material was characterized using XPS, SEM, BET, FT-IR and Raman spectroscopy. SEM images revealed an average diameter of 4.55 μm in a uniform sphere-like structure. The removal of hazardous HMs from the synthetic aqueous samples by the prepared carbon microspheres was reported to be selective and rapid. The carbon microspheres in this work were reported to have the highest adsorption capacities ever observed for Cr3+, Pb2+, Cd2+ and Cu2+ at an optimal pH 5, with adsorption capacities of 792, 638, 574 and 345 mg g−1, respectively. Good agreement with the results of adsorption studies was reported using density functional theory predictions, in which Cr3+ had the highest binding affinity to the –OH and –COOH functional groups followed by those of Cu2+, Pb2+, and Cd2+. The results reported for Pb2+ ion removal indicated that WS could be used use as an inexpensive adsorbent for Pb2+. Based on the qm values in Table 2, more than 300 mg g−1 of Pb2+ could be removed using WS. In addition, the highest amounts of adsorption were achieved (>600 mg g−1) ACs in the form of carbon microsphere was used. Such an approach may be applicable for increasing the adsorption capacity of WS for ther HMs.
7.1.10. Cadmium ion removal. It has been well documented that Cd2+ has adverse impact on the atmosphere and poses a serious threat to human health through accumulation in the food chain. Cd2+ is a toxic, carcinogenic, element that tends to accumulate in the body. Of the total Cd2+ discharged from different companies, 50% of the Cd2+ is released by electroplating facilities.114 The biosorption of Cd2+ metal ions on raw and chemically MWS has been reported.84 In this work, WS was treated with 0.1 N aqueous NaOH at 558 °C for 2 h to enhance its adsorptive capacity for Cd2+ ions. Using FT-IR and SEM analysis, the raw WS and alkali-treated WS were characterized by their morphological features and functional groups. Between pH 2 and 6, the adsorption increased drastically and the Langmuir isotherm model presented a better fit for the experimental data than the Freundlich isotherms, as determined by the providing high correlation coefficients. The biosorption capacity of raw WS and treated WS for Cd2+ ions was determined to be 4.20 and 14.29 mg g−1, respectively. The adsorption conformed to pseudo-second-order kinetics, suggesting a chemisorption process and monolayer coverage. There was no observable reduction in the desorption performance until the third cycle of the treatment.The use of different biochars to eliminate HMs from aqueous solution has progressed in recent years. Biochar is also known as an effective soil amendment for Cd2+ remediation. It is recommended that WSB be applied as a Cd2+ sorbent for soil remediation. Biochar prepared using WS was incubated in Cd (NO3)2 and kaolin for 15 days. The authors found that WSB could diminish the mobility of Cd2+. The biochar R50 value increased from 61.31 to 69.57–72.24% after incubation, indicating an increase in biochar stability. Their results explained that the improved biochar stability was possibly due to mechanical separation and the development of complexes and precipitates, created on the biochar surface or its inside.115
The application of nanomaterials to biochar surface may improve their ability to eliminate metal ions elimination. Accordingly, the effectiveness of WSB alone in combination with nanoscale zero-valent iron (WSB-nZVI) in Cd2+ removal in aqueous solution was examined. The results of this study revealed that in aqueous solution, WSB-nZVI provided a notable advantage to the performance of WSB for Cd2+ removal. The presence of functional groups on the WSB surface via adsorption and precipitation processes, as well as the nZVI developed on the WSB-nZVI from complexation and adsorption processes, resulted in an improved Cd2+ removal capacity compared to that of the raw WSB adsorbent. The predicted qm for the Cd2+ removal based on the recommended model was 99.72%, with initial Cd2+ concentration of 70.78 mg l−1, pH 6.92, adsorbent dose of 0.56 g l−1 and contact time of 40.42 min.86 A study of the kinetic and equilibrium of Pb2+ and Cd2+ removal from aqueous solution using processed WS was also undertaken by Almasi et al.85 In this study, the structural characterization by FT-IR spectroscopy of the adsorbent prepared from WS confirmed that –OH, –COOH, and –C
O groups were involved in Pb2+ and Cd2+ ions sorption. The highest adsorption was obtained within the pH range of 4–6. The Langmuir isotherm model was well adapted to the equilibrium kinetics. The highest monolayer adsorption potentials were determined to be 32 g kg−1 and 11.6 g kg−1 for Pb2+ and Cd2+ ions, respectively. The adsorption isotherms of the various HM ions removed by the WS were well fitted by the Langmuir and Freundlich models, and the kinetic data for the adsorption of the investigated HM by WS followed pseudo-second-order kinetic models. Furthermore, the sorption process was mostly spontaneous due to the negative values reported for ΔG. Additionally, in most studies, the reaction was enthalpy driven and endothermic. Thus, WS and its derivatives are suitable materials for use in the development of adsorption processes to eliminate HMs from industrial wastewater. Unprocessed WS an MWS presented high adsorption capacities for Cr6+, Pb2+ and Cu2+ ion removal (more than 200 mg g−1). The elimination Hg2+, Cd2+, Fe2+, Ni2+, Zn2+ and Mn2+ by WS and MWS is reported to be moderate (<200 mg g−1), and WS can only adsorb low levels of Cs+ and As3+ ions.
As shown in Table 1, considerable increases in the elimination of HM ions using WS were observed upon increasing the solution pH to 7, after which a reduction was observed. Accordingly, the highest HM removal values were achieved at pH values between 4 and 7. The reduced metal removal obtained at low pH values can be attributed to the mobility of H+ ions and their higher concentration; H+ ions have a higher adsorption affinity than HM ions. The lower H+ at higher pH values with a negatively charged adsorbent surface results in a greater attraction of metal cations. Nonetheless, a decrease in adsorption was observed under alkaline conditions, which may be due to the formation of several HM specific hydroxides that are precipitated and contribute to a decrease in the free metal ions in solution. In addition, more oxygen-containing groups on the adsorbent surface are ionized and adsorb more water at higher pH values. The development of water clusters on adsorbents blocks access to adsorption for of metal ions and results in a low adsorption capacity.
7.2. Dye removal
Colorants and pigments are essential in the textile industry and approximately 10
000 colorants are used in the textile industry, as well as in industrial chemistry.116 More than seven million tons of dye are generated worldwide each year and dyes are commonly used in various industries, including the paper, plastics, leather, pharmaceuticals, food, cosmetics, paint manufacturing and textile industries.117 Discharging up to 10% of these colorants during their manufacturing ad consumption can generate numerous issues for water supplies. They may influence of food and water, as well as trigger allergic dermatitis and skin sensitivities. In addition, these dyes can be toxic and dangerous and cause mutation in aquatic. Dyes can be cationic (basic), anionic (direct, acid, and reactive) or nonionic (dispersive). For the industry and the environment, the treatment of these pigmented wastewaters is a primary issue.118 Dye removal with the use of agricultural wastes and forestry residues has recently attracted considerable attention due to their abundance and low price of these by-products.4 WS is used for the removal of different dyes such as reactive brilliant red K-2BP (RBR K-2BP), C. I. Acid Red 97 (AR97), maxilon red (MR GRL), crystal violet (CV), methylene blue (MEB), rhodamine B (RB), reactive red 2 (RR2), malachite green (MG), methyl bromide (MB), and brilliant green (BG). The chemical structures of some basic dyes are shown in Fig. 2.
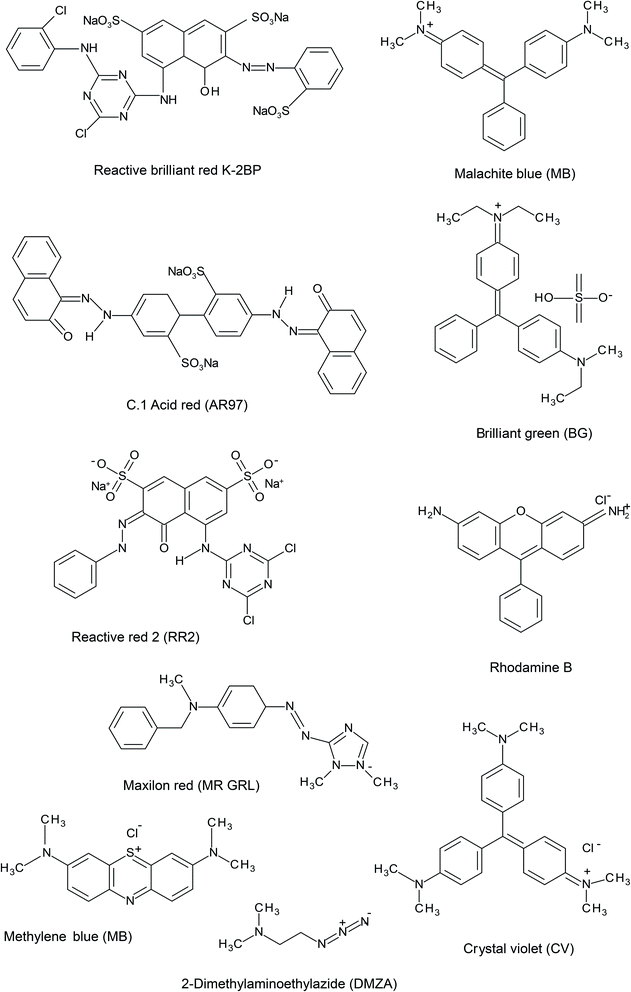 |
| Fig. 2 The chemical structures of several dyes removed by WS: RBR K-2BP, AR97, DAEA, MR GRL, CV, MEB, RB, RR2, MG, MB, and BG. | |
The obtained optimal operational conditions, including pH, adsorbent dosage, temperature, agitation speed, and contact time for the maximum efficiency (%) of the adsorption process are shown in Table 3. Additionally, Table 4 tabulates the values of qm obtained in the optimum experimental conditions, and the fitting and kinetic models, as well as the thermodynamics of the reaction for the different dyes removed by WS. In an investigation conducted by Ghazi Mokri et al.,119 the authors considered the kinetics, thermodynamic parameters, and isotherms of AR97 dye adsorption from an aqueous solution onto WS and recommended that WS could be used in the removal of AR97 dye. In this study, the effect of the operational parameters, including the adsorbent dosage, agitation speed, contact time, pH and temperature, were analysed in a batch method. The results showed that 73.33% of AR97 (50 mg l−1) was decolorized with 12 g l−1 of adsorbent at 60 min of contact time, and an agitation rate of 140 rpm at 18 °C. The adsorption rate followed pseudo-second-order kinetics. The SSA of WS was calculated to be 1.28 m2 g−1 by the BET technique. The thermodynamic values indicated that the nature of the adsorption process was spontaneous, exothermic and principally physical. The equilibrium data were in good agreement with the Freundlich isotherm model.
Table 3 The optimal conditions for the removal of different dyes by WSa
No |
Dye |
pH |
Adsorbent dosage |
Temp.(°C) |
Agitation speed (rpm) |
Contact time (min) |
Efficiency (%) |
Ref. |
— not reported. Modified WS. Ordinary WS. |
1 |
RBR K-2BP |
0.5–11 |
0.1–6 g l−1b |
40 |
180 |
— |
— |
Cao, et al.120 |
2 |
AR97 |
— |
12 g l−1c |
18 |
140 |
60 |
73.33 |
Ghazi Mokri, et al.119 |
3 |
DAEA |
10 |
0.29 gb |
— |
100 |
— |
85.95 |
Ghanbari Pakdehi and Rezaei121 |
4 |
MR GRL |
8 |
5 g l−1c |
45 |
— |
50 |
72.95 |
Deniz122 |
5 |
CV |
6 |
0.2 g l−1c |
45 |
150 |
360 |
83.7 |
Song, et al.123 |
7 |
0.8 g l−1b |
Room temp. |
200 |
13 |
— |
Ashrafi, et al.124 |
6 |
MEB |
7 |
0.75 g l−1b |
25 |
270 |
1440 |
— |
Yang and Qiu125 |
6.8 |
0.5 g l−1c |
20 |
4000 |
15 |
— |
Miyah, et al.126 |
7 |
RB |
3 |
0.05 gc |
— |
— |
30 |
100 |
Shah, et al.127 |
8 |
RR2 |
6.7 |
0.5 gc |
25 ± 3 |
— |
— |
— |
Almasi, et al.128 |
9 |
MG |
5 |
0.03 g/0.02 lc |
Room temp. |
250 |
120 |
87 |
Dahri, et al.129 |
10 |
MB |
— |
— |
— |
— |
— |
— |
Hall, et al.130 |
11 |
BG |
7 |
0.8 g l−1b |
25 ± 2 |
200 |
13 |
— |
Ashrafi, et al.124 |
Table 4 The adsorption capacity, thermodynamic, and fitting and kinetic models for the removal of various dyes by the WSa
No |
Dye |
qmb |
Fitting model |
Kinetic model |
Thermodynamic |
Ref. |
— not reported. Maximum adsorption capacity. Modified WS. Ordinary WS. |
1 |
RBR K-2BP |
568.18 mg g−1c |
Langmuir |
Pseudo-second order |
Spontaneous, endothermic |
Cao, et al.120 |
2 |
AR97 |
— |
Freundlich |
Pseudo-second order |
Spontaneous, exothermic |
Ghazi Mokri, et al.119 |
3 |
DAEA |
166.67 mg g−1c |
Freundlich |
Pseudo-second order |
Spontaneous, endothermic |
Ghanbari Pakdehi and Rezaei121 |
4 |
MR GRL |
58.21 mg g−1d |
Hill |
Logistic |
Endothermic |
Deniz122 |
5 |
CV |
714.3 mg g−1d |
Langmuir–Freundlich |
Pseudo-second order |
Spontaneous, endothermic |
Song, et al.123 |
96.01–123.2 mg g−1c |
Langmuir |
Pseudo-second order |
— |
Ashrafi, et al.124 |
6 |
MEB |
315 mg g−1c |
Redlich–Peterson |
— |
— |
Yang and Qiu125 |
Langmuir–Freundlich |
178.9 mg g−1d |
Langmuir |
Pseudo-second order |
Spontaneous, exothermic |
Miyah, et al.126 |
7 |
RB |
2.292 mg g−1d |
Langmuir |
Pseudo-second order |
Spontaneous, endothermic |
Shah, et al.127 |
8 |
RR2 |
1 mg l−1d |
Langmuir |
— |
— |
Almasi, et al.128 |
9 |
MG |
90.8 mg g−1d |
Langmuir |
Pseudo-second order |
Spontaneous, endothermic |
Dahri, et al.129 |
10 |
MB |
— |
— |
— |
— |
Hall, et al.130 |
11 |
BG |
79.07–146.40 mg g−1c |
Langmuir |
Pseudo-second order |
— |
|
Accordingly, the preparation of a new, low-cost and easy-to-use regeneration adsorbent by chemically MWS and an investigation of the kinetics and thermodynamics of the removal of anionic dye RBR K-2BP by this adsorbent was reported by Cao et al.120 In this study, epichlorohydrin and diethylenetriamine as an etherifying agent and crosslinking agent, respectively, were used in the synthesis of chemically MWS, and they were characterized by FT-IR, SEM, electron dispersive spectroscopy and thermogravimetric (TG) analysis. They evaluated the effects of adsorbent dosage (0.1–6 g l−1) and pH (0.5–11) on the adsorption capacity of chemically MWS. The highest adsorption capacity for RBR K-2BP was calculated to be 568.18 mg g−1 at 40 °C, which was nearly 10 times the adsorption capacity of the raw material, with Langmuir isotherm as the best-fitting model. The kinetics of adsorption were also well fitted with pseudo-second-order kinetics. According to thermodynamic characterization, the adsorption mechanism was spontaneous and endothermic. In addition, the chemically MWS was inexpensive, showed excellent recovery capacity and was promising for RBR K-2BP removal. The treatment of wastewater-contaminated CV using WS suggested that it is an effective adsorbent for the removal of this dye, and it is also inexpensive.123 Moreover, the removal of BG and CV from mono- and bi-component aqueous solutions using NaOH-modified WS has been studied.124
The possibility of using WS as a biosorbent for the removal of MR GRL dye from aqueous media was evaluated by Deniz et al.122 The authors determined the nonlinear kinetic and equilibrium models and then concluded that WS might offer an efficient and cost-effective technology for MR GRL removal from aqueous solution. In turn, to remove MEB as a cationic dye, ACs were prepared from the WS via the vacuum CA process.125 In another study, the efficiency of WS powder as a low-cost adsorbent for MB removal from aqueous solution was investigated in batch-scale experiments.126 The authors showed that MB can be removed from dye-bearing effluent in an ecofriendly way using WS powder. Kinetic, equilibrium, and thermodynamic studies were conducted on the removal of RB from aqueous solution and wastewater by WS.127 It was shown that WS, as a natural adsorbent, could be used for the removal of RR2 from aqueous solution.128 Equilibrium, kinetic, thermodynamic, and regeneration studies were also carried out to investigate water remediation with the use of WS as a low-cost adsorbent of MG.129 Hall et al.130 considered the end-products of tree nut and tree fruit production from walnut and almond shells as well as peach and prune pits that were grown in California, US for the preparation of ACs and used them as sorbents to remove MB in ventilation effluent from postharvest fumigation chamber. AC sorbents were prepared using different methods of pyrolysis, activation, and quenching.
By employing a solvent-free reaction, Ashrafi, et al.112 examined the modification of WS and obtained –COOH–functionalized WS. This material was mixed with malonic acid cyclic isopropylidene ester. The modified adsorbent efficiency for the simultaneous removal of Pb2+ and MEB was investigated. A high removal percentage, 92.61%, was obtained for MEB under the optimum experimental conditions. The FT-IR spectra revealed that π–π interactions and hydrogen bonding (H-bonding) were the main driving forces of MB adsorption on the developed adsorbent. It can be deduced from the available literature that the adsorption isotherm of various dyes removed by the WS was well fitted by the Langmuir and Freundlich models, and the kinetic data for the adsorption generally followed the pseudo-second-order kinetic model. The reaction was enthalpy driven, and it was endothermic. Furthermore, the sorption process of different dyes eliminated by WS was mostly spontaneous and favourable due to the negative values obtained for ΔG. Thus, WS can be considered in the adsorption processes for the removal of different industrial dyes. Additionally, the data presented in Table 4 show that high amounts of dyes (more than 500 mg g−1 of RBR K-2BP, CV and >300 mg g−1 of MEB) can be removed by the WS. Furthermore, the adsorption capacity of WS for other dyes, including DAEA, MEB (>150 mg g−1) and MR GRL, MG and BG is moderate. For dyes RB and RR2, the obtained qm values are below 5 mg g−1. For various dyes removed by WS, the optimum pH values were reported at neutral or alkaline conditions, which could be attributed to the increase in the negative charge on the surface of the adsorbent with increasing pH and the H+ ion reduction competing with the dye cations for the same positions on the biosorbent. For the anionic dyes removed by WS, the acidic pH supported the adsorption of the negatively-charged dye on the WS when the surface change of the WS was positive.
7.3. Oil separation
At various stages of production, transportation, refining, and use, oils can cause environmental pollution. Cutting liquids, fats, lubricants, heavy hydrocarbons such as tars, grease, crude oils (COs), diesel oil, and light hydrocarbons such as kerosene, jet fuel, and gasoline are the main oils found in contaminated waters.1 Petroleum refineries, metal manufacturing and machining, and food processors are the primary industrial sources of oily waste. Kitchen and human wastes are the main sources of oil in municipal wastewater.131 The capability of WS, as a low-cost and effective biosorbent, to remove different oils has also been studied. Standard mineral oil (SMO), vegetable oils (VOs), DoALL bright-edge 80 (DBE80), COs, refinery wastewater (RWW), and sunflower oils (SOs) were the main oils tested for removal by WS. Table 5 summarizes the values obtained under the optimal operational conditions, including the pH, adsorbent dosage, temperature, agitation speed, and contact time for maximum removal efficiency (%). Furthermore, to facilitate for better comparison, the qm or biofiltration capacity values along with the fitting and kinetic models, and the thermodynamics of the adsorption process for the separated oils using WS are summarized in Table 6. Batch kinetic studies were conducted by Srinivasan and Viraraghavan51 to evaluate the oil sorption capacities of WS media in pure oil and oil in an aqueous medium. They investigated SMO, VOs, and DBE80, found that the separated oil could be recovered from the WS media by applying pressure and showed that WS media was a suitable sorbent for oil removal. In 2010, the same authors132 conducted another batch study to evaluate the efficiencies of four biomaterials, i.e., fungal biomasses of Mucor rouxii and Absidia coerulea as well as CS and WS media, to remove the SMO, VOs and cutting oil from water. They demonstrated that M. rouxii had a better oil adsorption capacity than A. coerulea biomass, but the capacity of M. rouxii was still lower than those of CS and WS media. To compare the effectiveness of WS and polymeric resins at removing oil from water, two cross-linked polymeric resins based on glycidyl methacrylate-divinylbenzene (GMA-DVB) and styrene-divinylbenzene (STY-DVB) were synthesized, characterized and evaluated. The authors assumed that unlike WS, polymeric resins did not lose weight during processes that require interruption. They showed that WS HAD high REMOVAL efficiency (∼94%) but STY-DVB showed even better removal efficiency (∼100%) and additionally presented better mechanical resistance. Moreover, it was suggested that polymeric resins, mainly those based on GMA, can be chemically modified to remove specific contaminants that remain in the water after conventional treatment.133 The ability of pulverized WS to remove oil from aqueous solution has also been examined and demonstrated. The study involved a two-phase process in which the WS was used as a filtering bed for the accumulation and adsorption of oil onto its surface. The efficiency of oil removal from synthetic wastewater samples reached 96%, while the test results showed 75% efficiency of oil removal from the actual RWW discharged from the Al-Daura refinery in southern Baghdad.134 The qm values in the Table 4 show that, of the different oils that were tested with WS, SMO (>300 mg g−1), VOs (>500 mg g−1) and DBE80 (>500 mg g−1) could be effectively removed. Additionally, the capacity of WS to remove the mentioned oils is higher in an aqueous medium than in pure oil medium.
Table 5 The optimal conditions for the removal of different oils, drugs and other materials by WSa
No |
Materials |
pH |
Adsorbent dosage |
Temp (°C) |
Agitation speed (rpm) |
Contact time (min) |
Efficiency (%) |
Ref. |
— not reported. Modified WS. Ordinary WS. WS media. Pure oil medium. Oil in aqueous medium. |
1 |
Oils |
SMO |
7.5d |
3gc |
10e |
No agitation |
15e |
— |
Srinivasan and Viraraghavan51 |
20f |
140f |
5 |
0.2 gc |
20 |
175 |
360 |
83 |
Srinivasan and Viraraghavan132 |
7.6 |
38 |
VOs |
7.5d |
3 gc |
10e |
No agitation |
15e |
— |
Srinivasan and Viraraghavan51 |
20f |
90f |
5 |
0.2 gc |
20 |
175 |
360 |
96 |
Srinivasan and Viraraghavan132 |
7.6 |
60 |
DBE80 |
7.5d |
3gc |
10e |
No agitation |
15e |
— |
Srinivasan and Viraraghavan51 |
20f |
90f |
5 |
0.2 gc |
20 |
175 |
360 |
96 |
Srinivasan and Viraraghavan132 |
7.6 |
73 |
COs |
— |
— |
— |
— |
— |
∼94.2 |
Aversa, et al.133 |
RWW |
— |
4 gc |
25 ± 2 |
Shaking |
2880 |
75 |
Ziad Ismail134 |
SOs |
— |
4 gc |
25 ± 2 |
Shaking |
2880 |
96 |
Ziad Ismail134 |
2 |
CFX |
6.5 |
0.03 gb |
30 |
200 |
1200 |
100 |
Nazari, et al.135 |
— |
— |
30 |
— |
— |
— |
Nazari, et al.136 |
6.5 |
0.03 gb |
30 |
200 |
— |
— |
Nazari, et al.137 |
6.5 |
0.6 g l−1b |
30 |
Fixed-bed column |
— |
— |
Nazari, et al.138 |
3 |
EA |
6.3–7.2 |
— |
30 |
Packed bio-filter |
44 days |
>80 |
Zare, et al.139 |
4 |
Naphthalene |
7 |
1.0 g l−1b |
25 |
200 |
2400 |
— |
Zhu, et al.140 |
5 |
NOMs |
3 |
0.03 g l−1b |
— |
180 |
5 |
— |
Naghizadeh, et al.141 |
6 |
Methanol |
— |
— |
30 |
— |
120 |
— |
Yu, et al.142 |
7 |
Benzene |
12 |
0.3 g l−1b |
Ambient |
190 |
60 |
— |
Mataji and Khoshandam143 |
8 |
PH3 |
— |
— |
— |
— |
— |
— |
Yu, et al.142 |
9 |
NO3− |
6.5 |
200 gb |
25 |
— |
2 |
80 |
Alighardashi and Shahali144 |
2 |
1 gb |
20 |
Shaking |
15 |
41.7 |
Taghizadeh and Vahdati145 |
10 |
NH3–N |
6.8 |
15 g l−1b |
32 |
160 |
390 |
∼60 |
Ding, et al.146 |
11 |
SO2 |
— |
— |
90 |
Fixed-bed |
— |
— |
Guo, et al.147 |
12 |
MCPs |
2-CP |
2–4 |
1 g/0.04 lc |
— |
— |
240 |
∼75 |
Kuśmierek and Świątkowski148 |
3-CP |
2–4 |
1 g/0.04 lc |
— |
— |
240 |
∼75 |
Kuśmierek and Świątkowski148 |
4-CP |
2–4 |
1 g/0.04 lc |
— |
— |
240 |
∼75 |
Kuśmierek and Świątkowski148 |
Table 6 The adsorption capacity, thermodynamic, fitting and kinetic models for the removal of different oils, drugs and other materials by WSa
No |
Materials |
qmb |
Fitting model |
Kinetic model |
Thermodynamic |
Ref. |
— not reported. Maximum adsorption or bio-filtration capacity. Modified WS. Ordinary WS. Pure oil medium. Oil in aqueous medium. pH = 5. pH = 7.6. |
1 |
Oils |
SMO |
300 mg g−1e |
— |
— |
— |
Srinivasan and Viraraghavan51 |
560 mg g−1f |
∼80 mg g−1g |
— |
— |
— |
Srinivasan and Viraraghavan132 |
∼40 mg g−1h |
VOs |
510 mg g−1e |
— |
— |
— |
Srinivasan and Viraraghavan51 |
580 mg g−1f |
∼90 mg g−1g |
— |
— |
— |
Srinivasan and Viraraghavan132 |
∼60 mg g−1h |
DBE80 |
580 mg g−1e |
— |
— |
— |
Srinivasan and Viraraghavan51 |
740 mg g−1f |
∼90 mg g−1g |
— |
— |
— |
Srinivasan and Viraraghavan132 |
∼70 mg g−1h |
COs |
— |
— |
— |
— |
Aversa, et al.133 |
RWW |
∼3 mgd |
Langmuir |
— |
— |
Ziad Ismail134 |
SOs |
∼9 mgd |
Langmuir |
— |
— |
Ziad Ismail134 |
2 |
CFX |
233.1 mg g−1c |
Freundlich, Toth |
Pseudo-second order |
Spontaneous, endothermic |
Nazari, et al.135 |
— |
Freundlich |
— |
— |
Nazari, et al.136 |
233.10 mg g−1c |
Freundlich, Toth |
— |
— |
Nazari, et al.137 |
233.1 mg g−1c |
Freundlich |
— |
— |
Nazari, et al.138 |
3 |
EA |
— |
Michaelis–Menten |
Zero-order |
— |
Zare, et al.139 |
4 |
Naphthalene |
7.21 mg g−1c |
Freundlich |
Pseudo-second order |
— |
Zhu, et al.140 |
5 |
NOMs |
37.93 mg g−1c |
— |
— |
— |
Naghizadeh, et al.141 |
6 |
Methanol |
248.02 mg g−1c |
— |
— |
— |
Yu, et al.142 |
7 |
Benzene |
61.22 mg g−1c |
Langmuir Freundlich |
Pseudo-second order |
— |
Mataji and Khoshandam143 |
8 |
PH3 |
284.12 mg g−1c |
— |
— |
— |
Yu, et al.142 |
9 |
NO3− |
10 mg g−1c |
Langmuir |
— |
— |
Alighardashi and Shahali144 |
— |
Freundlich |
— |
— |
Taghizadeh and Vahdati145 |
10 |
NH3–N |
9.79 mg g−1c |
Langmuir |
Pseudo-second order |
Spontaneous, endothermic |
Ding, et al.146 |
11 |
SO2 |
— |
— |
— |
— |
Guo, et al.147 |
12 |
MCPs |
2-CP |
— |
Freundlich |
Pseudo-second order |
— |
Kuśmierek and Świątkowski148 |
3-CP |
— |
Freundlich |
Pseudo-second order |
— |
Kuśmierek and Świątkowski148 |
4-CP |
— |
Freundlich |
Pseudo-second order |
— |
Kuśmierek and Świątkowski148 |
7.4. Removal of pharmaceuticals
Municipal sewage and industrial wastewater are the primary effluents the release different pharmaceuticals into the environment. Drugs are known as extremely resistant chemicals and are not-biodegradable. They remain in the environment for a long time and are discharged continuously, especially antibiotics. Thus, the disposal and presence of pharmaceuticals in the environment has caused public concern over their long-term effects on human health and has highlighted the need for their removal from wastewater before discharging it into the environment.2 Research on the removal of pharmaceuticals using WS is very scarce, and it is limited to the removal of cephalexin (CFX), the chemical structure of which is shown in Fig. 3. This drug is the most widely used cephalosporin antibiotic with an annual use of 3000 tons and annual sales revenue of $850
000
000 at the beginning of the 21st century. The operational considerations reported under the optimized conditions including the pH, adsorbent dosage, temperature, agitation speed, contact time, efficiency (%), qm, fitting model, kinetic model, and thermodynamics for the CFX removal by WS, are summarized in Tables 5 and 6. Nazari et al.135 prepared an adsorbent by the CA method using ZnCl2 as the chemical activator agent. In this investigation, batch adsorption experiments were undertaken to investigate the adsorption of CFX antibiotics on WSACs. In another investigation performed by the same research group,136 a fixed-bed column study was carried out to study the mass transfer coefficient of CFX adsorption onto WSACs. They calculated the mass transfer coefficients by employing the constant wave propagation theory. They also considered the effect of different conditions on the mass transfer coefficient and developed three models to predict the mass transfer coefficient. The same researchers137 conducted batch adsorption studies of CFX by WSACs both experimentally and mathematically. The adsorbent was prepared via the CA method using the chemical activator ZnCl2. The model revealed good agreement with the experimental data, and the effective diffusivity of CFX in the WSACs was estimated at 0.47 × 10−9 m2 s−1. The same authors also considered the aqueous phase adsorption of CFX by WSACs in a fixed-bed column.138 This investigation showed that the ACs prepared from WS by the CA procedure were an effective and promising adsorbent for removing CFX from aqueous solution in a fixed-bed adsorption column. In the case of pharmaceuticals removed by WS, Table 6 shows that the adsorption isotherm was well fitted with the Toth and Freundlich isotherms, and the kinetic data for the adsorption by WS mostly followed the pseudo-second-order kinetic model. Additionally, the negative values of ΔG indicate that the sorption process was enthalpy driven, endothermic and spontaneous. The adsorption reaction of CFX by WS is highly favorable. Unfortunately, the information regarding pharmaceuticals removal using WS is limited to the elimination of CFX antibiotics. WS exhibits an acceptable adsorption capacity for CFX (233 mg g−1). Thus, it could be used as an effective sorbent in the elimination of other pharmaceuticals.
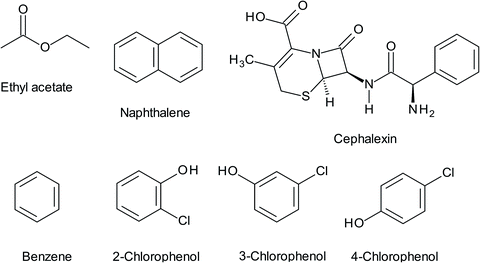 |
| Fig. 3 The chemical structures of some hazardous materials removed by the WS including CFX, EA, naphthalene, benzene, and MCP derivatives (2-CP, 3-CP, and 4-CP). | |
7.5. Separation of other hazardous materials
Different HMs, dyes, and oils can be removed from wastewaters using WS, and various hazardous materials are also reported to be effectively eliminated from contaminated systems using WS. Ethyl acetate (EA), naphthalene, natural organic matter (NOM), methanol, benzene, phosphine (PH3), nitrate (NO3−), ammonia nitrogen (NH3–N), sulfur dioxide (SO2), dimethyl aminoethyl azide (DAEA), monochlorophenols (MCPs) including 2-chlorophenol (2-CP), 3-chlorophenol (3-CP), and 4-chlorophenol (4-CP) (see also Fig. 3), are dangerous chemicals that were tested for removal using WS. Table 5 gives an overview of the adsorbent dosage, pH, temperature, agitation speed, and contact time values obtained as the optimal operational conditions for the maximum removal efficiency (%). Moreover, the qm values, thermodynamics, and fitting and kinetic models for different hazardous materials separated by WS are summarized in Table 6. A biofilter packed with WS served to remove EA from an air stream; in turn, NaOH-treated WS were used as a medium for Pseudomonas putida PTCC 1694 (P. putida) immobilization. The authors concluded that P. putida immobilized on WS has the potential to remove EA from air streams.139 The adsorption of the liquid fuel DAEA, a noncarcinogenic liquid fuel used in space programmes, from dilute aqueous solution on ACs made of WS was considered by Ghanbari Pakdehi and Rezaei,121 who demonstrated the feasibility of using WS for DAEA removal. They concluded that low concentrations of DAEA should be separated from dilute aqueous solutions to prevent environmental pollution. The adsorption of naphthalene from aqueous solution onto fatty acid-modified WS was also reported by Zhu et al.140 Different fatty acids, including capric acid, lauric acid, palmitic acid, and oleic acid were tested in this work to develop a low-cost biosorbent for hydrophobic organic compounds. The authors recommended that, compared with other modified sorbents, oleic acid-grafted WS showed the maximum partition coefficient (4330 ± 8.8 l kg−1) because it showed the lowest polarity and the highest aromaticity. The ACs derived from WS were also investigated for their ability to adsorb benzene from a rich amine stream of gas sweetening systems.143
There have also been attempts to use WS with zinc oxide nanoparticles (ZnO NPs) to remove NOMs from aqueous solution.141 In this study, the authors used humic acid. NOMs are a group of linear and cyclic organic materials, including humic substances, hydrophilic and hydrophobic acids, and amino acids, and represent a mixture of chemically complex polyelectrolytes produced mainly from the decomposition of plant and animal residues. They occur in surface and groundwater resources, and their high concentrations in raw water and municipal water utilities create serious problems. Methanol adsorption by WSACs prepared via KOH activation has also been investigated142 to analyse its adsorption performance under refrigerated conditions.
WS was also used to prepare ACs for desulfurization.147 Titanium ore (TO), TiO2, or Fe2O3 was blended into the carbonized powders, which were extruded into cylinders and activated under the atmospheric CO2. The desulfurization of the samples was evaluated in a fixed bed under a simulated gaseous mixture from coal combustion. The authors found that WS was a useful material for preparation ACs for desulfurization. They observed that the addition of TO, TiO2, or Fe2O3 into the WSACs increased the SSA and concluded that pore structure and metal oxides are the main factors affecting the desulfurization performance. To study the adsorption of phosphine (PH3), ACs were prepared from WS by the KOH and CA processes.149 The adsorption of NH3–N in wastewater by MWS has also reported. An adsorbent was prepared by carbonizing WS using ZnCl2 as the activating agent and was studied to its potential for liquid-phase adsorptive removal of NH3–N.146 The removal of NO3− from aqueous solution has been examined by comparing the characteristics of various samples of ACs made of walnut, pistachio, and almond shells using ZnCl2 as the chemical activator.145 Another study aimed to determine the potential chemical ACs derived from WS to remove NO3− from synthetic aqueous solution and groundwater in a continuous pilot system.144
Walnut, pistachio, and hazelnut shells were also investigated for their ability to remove three MCPs (2-CP, 3-CP, and 4-CP) from aqueous solution.148 The authors concluded that the effectiveness of the MCPs removal from the water on the walnut, pistachio and hazelnut shells for MCP removal from the water was comparable and that the individual differences in the sorption of MCPs were also negligible. According to this study and the data on the optimized conditions summarized in Table 6, it can be observed that the adsorption isotherms of the different considered materials were well fitted with Langmuir and Freundlich models, and the kinetic data for the adsorption by WS usually followed the pseudo-second-order kinetics. Additionally, due to the negative values of ΔG, and the enthalpy-driven, endothermic removal process, the sorption process of the removed hazardous materials was mostly spontaneous. Subsequently, as for the HMs, dyes and oils discussed in earlier sections, WS has high potential to be used as an inexpensive biosorbent for the removal of other hazardous materials. Finally, as shown in the obtained qm values summarized in Table 6, WS could adsorb significant amounts of methanol (>200 mg g−1) and PH3 (>250 mg g−1). Consequently, WS can be considered as an inexpensive and cost-effective adsorbent for the removal of methanol and PH3.
8. Conclusions
The waste by-products of walnut fruit constitute approximately 40–60% of the weight of the fruit and are readily available in walnut production centres as a source of valuable compounds such as phenolics. These by-products are generated in high quantities upon processing the walnut fruit to obtain its kernel, and they are typical discarded or used as fuel. Recent investigations on different parts of the walnut fruit have shown that its waste products could be used effectively due to their many beneficial properties and valuable compounds, with opens new possibilities for enhancing the value of walnut fruit coproducts. This review presented a wide range of hazardous materials such as various HM ions, various dyes, different oils, and various other toxic compounds that could be removed from aqueous solution by ordinary or chemically-modified WS. The ACs from the WS is an appropriate adsorbent for the removal of hazardous chemicals from aqueous solution. Inexpensive, locally available, and effective raw and treated plant-based waste products such as WS could be utilized as alternative materials, rather than expensive commercial ACs for the removal of different dangerous materials. From the large number of investigations reviewed here, it can be inferred that the adsorption mechanism and the adsorption kinetics on various hazardous substances by WS depend on the chemical nature of the investigated materials and various physicochemical experimental conditions such as the solution pH, the initial adsorbate concentration, the adsorbent dosage, and the temperature of the system. Consequently, these factors must be taken into account when the adsorption capacities of different forms of WS are considered.
An additional point is that raw or chemically activated low-cost plant products, such as WS powder, have high potential for applications in the removal of different hazardous materials including HM ions, dyes, oils, and other chemicals from aqueous solution. The biosorption of the different considered substances onto ordinary or processed WS was well described by the Langmuir and Freundlich isotherm models, demonstrating the chemisorption as the dominating mechanism of biosorption. The kinetic data reported for the various investigated HMs, dyes, oils and other hazardous indicated that those reactions mostly followed the pseudo-second-order model. According to the obtained thermodynamic parameters, the adsorption processes for the different studied hazardous materials removed by WS were enthalpy driven, endothermic, and spontaneous. It follows that raw or processed WS is a promising biosorbent for the removal of diverse hazardous substances from industrial wastewaters. This low-cost material is cost-effective and can be used as a bioremediation method for detoxification purposes.
The comprehensive review of the literature also shows the existing gap in the current knowledge and indicates a need for more studies to be performed to: (I) predict the performance of the adsorption process for the removal of various adsorbates by WS from real effluents under a wide range of operating conditions, (II) investigate the adsorbate removal from mixed wastewater, (III) better understand the adsorption mechanism of different hazardous materials on WS, (IV) verify the feasibility of various chemically modified WS types at an industrial scale, and (V) enhance the adsorption rate, shorten the reaction time, and include composite nanomaterial adsorbents during the adsorption process using WS. With these studies, more effective modifications can be made to improve the of WS in treating industrial wastewater.
Conflicts of interest
The authors declare no conflicts of interest.
Abbreviations
2-CP | 2-Chlorophenol |
3-CP | 3-Chlorophenol |
4-CP | 4-Chlorophenol |
ACs | Activated carbons |
AMWS | Amino-modified walnut shell |
ANN | Artificial neural network |
AR97 | C. I. acid red 97 |
As | Arsenic |
AWS | Acid-treated walnut shell |
AWSCS | Acid-treated walnut shell impregnated on chitosan |
BET | Brunner–Emmett–Teller |
BG | Brilliant green |
–C O | Carbonyl |
CA | Chemical activation |
CAMWS | Citric acid-modified walnut shell |
Cd | Cadmium |
CFWS | Carboxylate-functionalized walnut shell |
CFX | Cephalexin |
CNFs | Carbon nanofibre |
–CONH2 | Amide |
–COOH | Carboxyl |
COs | Crude oils |
Cr | Chromium |
CS | Chitosan |
Cs | Caesium |
Cu | Copper |
CV | Crystal violet |
DAEA | Dimethyl amino ethyl azide |
DBE80 | DoALL bright-edge 80 |
EA | Ethyl acetate |
Fe | Iron |
FT-IR | Fourier transform infrared spectroscopy |
GACs | Granular activated carbons |
GMA-DVB | Glycidyl methacrylate-divinylbenzene |
H3PO4 | Phosphoric acid |
H-bonding | Hydrogen bonding |
Hg | Mercury |
HMs | Heavy metals |
K | Potassium |
K2CO3 | Potassium carbonate |
MB | Methyl bromide |
MCPs | Monochlorophenols |
MEB | Methylene blue |
MG | Malachite green |
MLR | Multiple linear regression |
Mn | Manganese |
MR GRL | Maxilon red GRL |
MWCNTs | Multi-walled carbon nanotubes |
MWS | Modified walnut shell |
NaOH | Sodium hydroxide |
NH3–N | Ammonia nitrogen |
Ni | Nickel |
NiHCF | Nickel hexacyanoferrate |
NO3− | Nitrate |
NOMs | Natural organic matters |
–OH | Hydroxyl |
P. putida | Pseudomonas putida |
PA | Physical activation |
Pb | Lead |
PH3 | Phosphine |
PZC | Lower point of zero charge |
qm | Maximum adsorption capacity |
RB | Rhodamine B |
RBR K-2BP | Reactive brilliant red K-2BP |
RR2 | Reactive red 2 |
RWW | Refinery wastewater |
SEM-EDX | Scanning electron microscopy-energy-dispersive X-ray spectroscopy |
SFWS | Sulfur functionalized walnut shell |
SMO | Standard mineral oil |
SO2 | Sulfur dioxide |
SOs | Sunflower oils |
SSA | Specific surface area |
STY-DVB | Styrene-divinylbenzene |
SWCNTs | Single-walled carbon nanotubes |
SWCNTs/Fe | Single-walled carbon nanotubes-doped iron |
SWCNTs/WSC | Single-walled carbon nanotubes-doped walnut shell composite |
Temp | Temperature |
TG | Thermogravimetric |
TO | Titanium ore |
US | United States |
US EPA | United States Environmental Protection Agency |
UST | Ultrasound technology |
UWM | Ultrafine water mist |
VOs | Vegetable oils |
WHO | World health organization |
WMSACS | Watermelon activated carbons |
WS | Walnut shell |
WSACs | Walnut shell activated carbons |
WSB | Walnut shell biochar |
WSB-nZVI | Walnut shell biochar-nanoscale zero-valent iron |
WSC | Walnut shell composite |
WSCS | Walnut shell impregnated on chitosan |
XPS | X-ray photoelectron spectroscopy |
Zn | Zinc |
ZnCl2 | Zinc chloride |
ZnO NPs | Zinc oxide nanoparticles |
Acknowledgements
This work was supported by the Nutrition Research Center, Student Research Committee, Drug Applied Research Center, Tabriz University of Medical Sciences, Tabriz, Iran, and the Institute of Animal Reproduction and Food Research of the Polish Academy of Sciences, Olsztyn, Poland.
References
- R. M. Harrison, Pollution: causes, effects and control, Royal Society of Chemistry, 2001 Search PubMed.
- M. J. Ahmed and B. H. Hameed, Ecotoxicol. Environ. Saf., 2018, 149, 257–266 CrossRef CAS PubMed.
- J. Mo, Q. Yang, N. Zhang, W. Zhang, Y. Zheng and Z. Zhang, J. Environ. Manage., 2018, 227, 395–405 CrossRef CAS PubMed.
- K. A. Adegoke and O. S. Bello, Water Resources and Industry, 2015, 12, 8–24 CrossRef.
- M. T. Yagub, T. K. Sen, S. Afroze and H. M. Ang, Adv. Colloid Interface Sci., 2014, 209, 172–184 CrossRef CAS PubMed.
- A. Jahanban-Esfahlan, A. Ostadrahimi, M. Tabibiazar and R. Amarowicz, Int. J. Mol. Sci., 2019, 20, 3920 CrossRef CAS PubMed.
- A. Jahanban-Esfahlan and R. Amarowicz, RSC Adv., 2018, 8, 22376–22391 RSC.
- G. McGranahan and C. Leslie, in Genetic resources of temperate fruit and nut crops, 1991, pp. 907–974 Search PubMed.
- M. S. Wani, A. Hussain, S. A. Ganie, A. H. Munshi, E. P. Lal and R. C. Gupta, International Journal of Latest Research in Science and Technology, 2016, 5, 90–97 Search PubMed.
- S. Yilmaz, Y. Akça and S. Saçlik, J. Int. Sci. Publ., 2017, 5, 389–397 Search PubMed.
- N. Mishra, A. Dubey, R. Mishra and N. Barik, Food Chem. Toxicol., 2010, 48, 3316–3320 CrossRef CAS PubMed.
- Z. Zhang, L. Liao, J. Moore, T. Wu and Z. Wang, Food Chem., 2009, 113, 160–165 CrossRef CAS.
- M. Kornsteiner, K.-H. Wagner and I. Elmadfa, Food Chem., 2006, 98, 381–387 CrossRef CAS.
- P. M. Kris-Etherton, F. B. Hu, E. Ros and J. Sabaté, J. Nutr., 2008, 138, 1746S–1751S CrossRef CAS PubMed.
- P. M. Kris-Etherton, G. Zhao, A. E. Binkoski, S. M. Coval and T. D. Etherton, Nutr. Rev., 2001, 59, 103–111 CrossRef CAS PubMed.
- T. Fukuda, H. Ito and T. Yoshida, Phytochemistry, 2003, 63, 795–801 CrossRef CAS.
- O. Aruoma, Food Chem. Toxicol., 1994, 32, 671–683 CrossRef CAS PubMed.
- R. Blomhoff, M. H. Carlsen, L. F. Andersen and D. R. Jacobs, Br. J. Nutr., 2006, 96, S52–S60 CrossRef CAS PubMed.
- R. U. Almario, V. Vonghavaravat, R. Wong and S. E. Kasim-Karakas, Am. J. Clin. Nutr., 2001, 74, 72–79 CrossRef CAS PubMed.
- I. F. Almeida, E. Fernandes, J. L. Lima, P. C. Costa and M. Fernanda Bahia, Food Chem., 2008, 106, 1014–1020 CrossRef CAS.
- A. Chauhan, M. M. Essa, B. Muthaiyah, V. Chauhan, K. Kaur and M. Lee, Alzheimer's Dementia, 2010, 6, S69 CrossRef.
- E. B. Feldman, J. Nutr., 2002, 132, 1062S–1101S CrossRef PubMed.
- G. E. Fraser, J. Sabate, W. L. Beeson and T. M. Strahan, Arch. Intern. Med., 1992, 152, 1416 CrossRef CAS PubMed.
- F. B. Hu and M. J. Stampfer, Curr. Atheroscler. Rep., 1999, 1, 204–209 CrossRef CAS PubMed.
- F. Lavedrine, D. Zmirou, A. Ravel, F. Balducci and J. Alary, Prev. Med., 1999, 28, 333–339 CrossRef CAS PubMed.
- J. Yang, R. H. Liu and L. Halim, LWT--Food Sci. Technol., 2009, 42, 1–8 CrossRef CAS.
- D. Vyas, S. K. Sharma and D. R. Sharma, Sci. Hortic., 2003, 97, 141–152 CrossRef CAS.
- V. Akbari, R. Jamei, R. Heidari and A. Jahanban Esfahlan, Food Chem., 2012, 135, 2404–2410 CrossRef CAS PubMed.
- F. Stampar, A. Solar, M. Hudina, R. Veberic and M. Colaric, Food Chem., 2006, 95, 627–631 CrossRef CAS.
- A. Jahanban-Esfahlan, A. Ostadrahimi, M. Tabibiazar and R. Amarowicz, Molecules, 2019, 24, 2133 CrossRef CAS.
- I. Oliveira, A. Sousa, I. C. F. R. Ferreira, A. Bento, L. Estevinho and J. A. Pereira, Food Chem. Toxicol., 2008, 46, 2326–2331 CrossRef CAS.
- J. Bruneton, Pharmacognosy, Phytochemistry, Medicinal Plants, Hampshire, United Kingdom, 2nd edn, 1999 Search PubMed.
- J. S. Amaral, R. M. Seabra, P. B. Andrade, P. Valentao, J. A. Pereira and F. Ferreres, Food Chem., 2004, 88, 373–379 CrossRef CAS.
- S. Hosseini, H. Fallah Huseini, B. Larijani, K. Mohammad, A. Najmizadeh, K. Nourijelyani and L. Jamshidi, Daru, J. Pharm. Sci., 2014, 22, 19 CrossRef PubMed.
- S. Hosseini, S. Mehrzadi, A. R. Najmizadeh, M. Kazem, H. Alimoradi and H. Fallah Huseini, J. Ethnopharmacol., 2014, 152, 451–456 CrossRef PubMed.
- J. A. Pereira, I. Oliveira, A. Sousa, P. Valentão, P. B. Andrade, I. C. F. R. Ferreira, F. Ferreres, A. Bento, R. Seabra and L. Estevinho, Food Chem. Toxicol., 2007, 45, 2287–2295 CrossRef CAS PubMed.
- A. Pitschmann, M. Zehl, A. G. Atanasov, V. M. Dirsch, E. Heiss and S. Glasl, J. Ethnopharmacol., 2014, 152, 599–602 CrossRef CAS PubMed.
- H. Eghbali, S. M. Hosseini-Zijoud, S. Nabati and M. Mahmoodi, Clin. Biochem., 2011, 44, S331 Search PubMed.
- A. Solar, M. Colarič, V. Usenik and F. Stampar, Plant Sci., 2006, 170, 453–461 CrossRef CAS.
- Q. Wei, X. Ma and J. Dong, J. Anal. Appl. Pyrolysis, 2010, 87, 24–28 CrossRef CAS.
- F. H. Epstein, V. Fuster, L. Badimon, J. J. Badimon and J. H. Chesebro, N. Engl. J. Med., 1992, 326, 242–250 CrossRef PubMed.
- D. Kromhout, E. B. Bosschieter and C. d. L. Coulander, N. Engl. J. Med., 1985, 312, 1205–1209 CrossRef CAS PubMed.
- S. B. Lotito and B. Frei, Free Radic. Biol. Med., 2006, 41, 1727–1746 CrossRef CAS PubMed.
- E. Ros, Am. J. Clin. Nutr., 2009, 89, 1649S–1656S CrossRef CAS PubMed.
- W. J. Craig, Am. J. Clin. Nutr., 1999, 70, 491s–499s CrossRef CAS PubMed.
- D. O. Labuckas, D. M. Maestri, M. Perelló, M. L. Martínez and A. L. Lamarque, Food Chem., 2008, 107, 607–612 CrossRef CAS.
- I. Arcan and A. Yemenicioğlu, J. Food Compos. Anal., 2009, 22, 184–188 CrossRef CAS.
- A. Jahanban-Esfahlan, S. Davaran, A. Moosavi-Movahedi and S. Dastmalchi, J. Iran. Chem. Soc., 2017, 7, 1527–1540 CrossRef.
- L. Roufegarinejad, R. Amarowicz and A. Jahanban-Esfahlan, J. Biomol. Struct. Dyn., 2019, 37, 2766–2775 CrossRef CAS PubMed.
- A. Jahanban Esfahlan, R. Jamei and R. Jahanban Esfahlan, Food Chem., 2010, 120, 349–360 CrossRef.
- A. Srinivasan and T. Viraraghavan, Bioresour. Technol., 2008, 99, 8217–8220 CrossRef CAS PubMed.
- T. Altun and E. Pehlivan, Food Chem., 2012, 132, 693–700 CrossRef CAS.
- E. V. Mathias and U. P. Halkar, J. Anal. Appl. Pyrolysis, 2004, 71, 515–524 CrossRef CAS.
- D. Ding, Y. Zhao, S. Yang, W. Shi, Z. Zhang, Z. Lei and Y. Yang, Water Res., 2013, 47, 2563–2571 CrossRef CAS PubMed.
- N. A. Sarsari, S. Pourmousa and A. Tajdini, BioResources, 2016, 11, 6968–6983 CAS.
- K. Harini, C. Chandra Mohan, K. Ramya, S. Karthikeyan and M. Sukumar, Carbohydr. Polym., 2018, 184, 231–242 CrossRef CAS PubMed.
- M. Zabihi, A. Haghighi Asl and A. Ahmadpour, J. Hazard. Mater., 2010, 174, 251–256 CrossRef CAS PubMed.
- Q. Wei, X. Ma, Z. Zhao, S. Zhang and S. Liu, J. Anal. Appl. Pyrolysis, 2010, 88, 149–154 CrossRef CAS.
- A. Karimi-Jashni and S. Saadat, Iran. J. Sci. Technol., Trans. Civ. Eng., 2014, 38, 309–324 Search PubMed.
- L. Cheng, L. Sun, W. Xue, Z. Zeng and S. Li, Environ. Prog. Sustain. Energy, 2016, 35, 1724–1731 CrossRef CAS.
- A. Ahmadpour and D. Do, Carbon, 1996, 34, 471–479 CrossRef CAS.
- A. Mohammad-Khah and R. Ansari, Int. J. ChemTech Res., 2009, 1, 2745–2788 Search PubMed.
- J. i. Hayashi, T. Horikawa, I. Takeda, K. Muroyama and F. Nasir Ani, Carbon, 2002, 40, 2381–2386 CrossRef CAS.
- J.-W. Kim, M.-H. Sohn, D.-S. Kim, S.-M. Sohn and Y.-S. Kwon, J. Hazard. Mater., 2001, 85, 301–315 CrossRef CAS.
- M. Soleimani and T. Kaghazchi, Adv. Chem. Eng. Res., 2014, 3, 34–41 CAS.
- H. Karatas and F. Akgun, Fuel, 2018, 214, 285–292 CrossRef CAS.
- S. Babel and T. A. Kurniawan, J. Hazard. Mater., 2003, 97, 219–243 CrossRef CAS.
- A. Demirbas, J. Hazard. Mater., 2008, 157, 220–229 CrossRef CAS PubMed.
- F. Fu and Q. Wang, J. Environ. Manage., 2011, 92, 407–418 CrossRef CAS PubMed.
- S. E. Bailey, T. J. Olin, R. M. Bricka and D. D. Adrian, Water Res., 1999, 33, 2469–2479 CrossRef CAS.
- W. Liu, J. Zhang, C. Zhang, Y. Wang and Y. Li, Chem. Eng. J., 2010, 162, 677–684 CrossRef CAS.
- T. Budinova, D. Savova, L. Btsyntsarski, C. Ania, B. Cabal, J. Parra and N. Petrov, Appl. Surf. Sci., 2009, 255, 4650–4657 CrossRef CAS.
- A. H. A. Mahvi, D. Naghipour, F. Vaezi and S. Nazmara, Am. J. Appl. Sci., 2005, 2, 372 CrossRef CAS.
- M. Saifuddin and P. Kumaran, Electron. J. Biotechnol., 2005, 8, 43–53 Search PubMed.
- E. Pehlivan and T. Altun, J. Hazard. Mater., 2008, 155, 378–384 CrossRef CAS.
- R. Xie, H. Wang, Y. Chen and W. Jiang, Environ. Prog. Sustain. Energy, 2013, 32, 688–696 CrossRef CAS.
- M. Feizi and M. Jalali, J. Taiwan Inst. Chem. Eng., 2015, 54, 125–136 CrossRef CAS.
- M. Kazemipour, M. Ansari, S. Tajrobehkar, M. Majdzadeh and H. R. Kermani, J. Hazard. Mater., 2008, 150, 322–327 CrossRef CAS PubMed.
- M. Ghasemi, A. A. Ghoreyshi, H. Younesi and S. Khoshhal, Iran. J. Chem. Chem. Eng., 2015, 12, 28–51 Search PubMed.
- S. Nethaji and A. Sivasamy, Clean Technol. Environ. Policy, 2014, 16, 361–368 CrossRef CAS.
- X. Lu, J. Duan and Y. Huang, Agric. Sci. Technol., 2015, 16, 1989–1993 Search PubMed.
- H. Jafari-Mansoorian, M. Farzadkia, M. Ansari, E. Ahmadi, G. Majidi, A. Amraie and A. Joghataie, J. Saf. Promot. Inj. Prev., 2016, 3, 287–294 Search PubMed.
- A. N. S. Saqib, A. Waseem, A. F. Khan, Q. Mahmood, A. Khan, A. Habib and A. R. Khan, Ecol. Eng., 2013, 51, 88–94 CrossRef.
- S. C. Gondhalekar and S. R. Shukla, Environ. Prog. Sustain. Energy, 2015, 34, 1613–1619 CrossRef CAS.
- A. Almasi, M. Omidi, M. Khodadadian, R. Khamutian and M. B. Gholivand, Toxicol. Environ. Chem., 2012, 94, 660–671 CrossRef CAS.
- M. Saffari, J. Chem. Health Risks, 2018, 8, 19–37 CAS.
- Z.-j. Yi, J. Yao, Y.-f. Kuang, H.-l. Chen, F. Wang and Z.-m. Yuan, Water Sci. Technol., 2015, 72, 983–989 CrossRef CAS PubMed.
- J. J. Moreno-Barbosa, C. López-Velandia, A. del Pilar Maldonado, L. Giraldo and J. C. Moreno-Piraján, Adsorption, 2013, 19, 675–685 CrossRef CAS.
- S. Saadat, A. Karimi-Jashni and M. M. Doroodmand, J. Environ. Chem. Eng., 2014, 2, 2059–2067 CrossRef CAS.
- S. Saadat and A. Karimi-Jashni, Chem. Eng. J., 2011, 173, 743–749 CrossRef CAS.
- A. Gala and S. Sanak-Rydlewska, Pol. J. Environ. Stud., 2011, 20, 877–883 CAS.
- D. Ding, Z. Lei, Y. Yang, C. Feng and Z. Zhang, J. Hazard. Mater., 2014, 270, 187–195 CrossRef CAS PubMed.
- M. Zabihi, A. Ahmadpour and A. Haghighi Asl, J. Hazard. Mater., 2009, 167, 230–236 CrossRef CAS PubMed.
- A. Hawari, Z. Rawajfih and N. Nsour, J. Hazard. Mater., 2009, 168, 1284–1289 CrossRef CAS PubMed.
- T. Bakalár and H. Pavolová, Environ. Prot. Eng., 2019, 45, 35–54 Search PubMed.
- S. Davidi, A. Lashanizadegan and H. Sharififard, Mater. Res. Express, 2019, 6, 085621 CrossRef CAS.
- O. Olafadehan, O. Akpo, O. Enemuo, K. O. Amoo and O. G. Abatan, Afr. J. Environ. Sci. Technol., 2018, 12, 334–355 Search PubMed.
- S. Zhou, Y. Wei, B. Li and H. Wang, J. Clean. Prod., 2019, 217, 423–431 CrossRef CAS.
- H. Kalavathy, B. Karthik and L. R. Miranda, Colloids Surf., B, 2010, 78, 291–302 CrossRef CAS.
- Ş. Tulun, T. Bahadır, İ. Şimşek and M. Karataş, Turk. J. Eng., 2019, 3, 102–105 Search PubMed.
- J. Ayala and B. Fernández, Environ. Prot. Eng., 2019, 45, 141–158 Search PubMed.
- A. Ostermann, Y. He, J. Siemens, G. Welp, A. Heuser, F. Wombacher, C. Münker, Q. Xue, X. Lin and W. Amelung, Environ. Sci. Technol., 2015, 49, 4609–4617 CrossRef CAS.
- D. Milenković, M. Milosavljević and A. Bojić, Facta Univ., Ser.: Work. Living Environ. Prot., 2018, 15, 35–44 Search PubMed.
- L. Wu, Z. Shang, S. Chen, J. Tu, N. Kobayashi and Z. Li, RSC Adv., 2018, 8, 21993–22003 RSC.
- M. Banerjee, R. K. Basu and S. K. Das, Process Saf. Environ. Prot., 2018, 116, 693–702 CrossRef CAS.
- A. Nilchi, R. Saberi, M. Moradi, H. Azizpour and R. Zarghami, Chem. Eng. J., 2011, 172, 572–580 CrossRef CAS.
- X. Ma, K. Subramanian, C. Chakrabarti, R. Guo, J. Cheng, Y. Lu and W. Pickering, J. Environ. Sci. Health, Part A: Environ. Sci. Eng., 1992, 27, 1389–1404 Search PubMed.
- K. Zhang, W. Cheung and M. Valix, Chemosphere, 2005, 60, 1129–1140 CrossRef CAS PubMed.
- M. Ashrafi, H. Borzuie, G. Bagherian, M. A. Chamjangali and H. Nikoofard, Sep. Sci. Technol., 2020, 55, 222–233 CrossRef CAS.
- G. Liu, W. Zhang and R. Luo, Polym. Bull., 2019, 76, 1099–1114 CrossRef CAS.
- X.-g. Lu and Y.-t. Guo, Environ. Sci. Pollut. Res., 2019, 26, 12776–12787 CrossRef CAS PubMed.
- M. Ashrafi, G. Bagherian, M. A. Chamjangali, N. Goudarzi and A. H. Amin, Mater. Res. Express, 2018, 5, 065510 CrossRef.
- M. Zbair, H. A. Ahsaine, Z. Anfar and A. Slassi, Chemosphere, 2019, 231, 140–150 CrossRef CAS PubMed.
- S. J. Kulkarni and J. Kaware, Int. J. Innov. Res. Sci. Eng. Technol., 2013, 2, 465–469 Search PubMed.
- Z. Qiu, J. Chen, J. Tang and Q. Zhang, Sci. Total Environ., 2018, 636, 80–84 CrossRef CAS PubMed.
- M. Behnajady, N. Modirshahla, N. Daneshvar and M. Rabbani, Chem. Eng. J., 2007, 127, 167–176 CrossRef CAS.
- A. Afkhami and R. Moosavi, J. Hazard. Mater., 2010, 174, 398–403 CrossRef CAS.
- N. Daneshvar, M. Rabbani, N. Modirshahla and M. Behnajady, J. Photochem. Photobiol., A, 2004, 168, 39–45 CrossRef CAS.
- H. S. Ghazi Mokri, N. Modirshahla, M. A. Behnajady and B. Vahid, Int. J. Environ. Sci. Technol., 2015, 12, 1401–1408 CrossRef CAS.
- J.-S. Cao, J.-X. Lin, F. Fang, M.-T. Zhang and Z.-R. Hu, Bioresour. Technol., 2014, 163, 199–205 CrossRef CAS PubMed.
- S. Ghanbari Pakdehi and F. Rezaei, Desalin. Water Treat., 2016, 57, 27726–27740 Search PubMed.
- F. Deniz, Environ. Prog. Sustain. Energy, 2014, 33, 396–401 CrossRef CAS.
- Y. Song, H. Fang, H. Xu, X. Tan and S. Chen, J. Residuals Sci. Technol., 2016, 13, 243–249 CrossRef CAS.
- M. Ashrafi, G. Bagherian, M. Arab Chamjangali and N. Goudarzi, Anal. Bioanal. Chem. Res., 2018, 5, 95–114 CAS.
- J. Yang and K. Qiu, Chem. Eng. J., 2010, 165, 209–217 CrossRef CAS.
- Y. Miyah, A. Lahrichi, M. Idrissi, A. Khalil and F. Zerrouq, Surf. Interfaces, 2018, 11, 74–81 CrossRef CAS.
- J. Shah, M. R. Jan, A. Haq and Y. Khan, Front. Chem. Sci. Eng., 2013, 7, 428–436 CrossRef CAS.
- A. Almasi, S. A. Mousavi, A. Hesari and H. Janjani, Int. Res. J. Appl. Basic Sci., 2016, 10, 551–556 CAS.
- M. K. Dahri, M. R. R. Kooh and L. B. Lim, J. Environ. Chem. Eng., 2014, 2, 1434–1444 CrossRef CAS.
- W. A. Hall, D. E. Bellamy and S. S. Walse, J. Agric. Food Chem., 2015, 63, 3094–3103 CrossRef CAS PubMed.
- C. S. Hsu and P. R. Robinson, Practical advances in petroleum processing, Springer, 2006 Search PubMed.
- A. Srinivasan and T. Viraraghavan, Bioresour. Technol., 2010, 101, 6594–6600 CrossRef CAS PubMed.
- T. M. Aversa, C. M. Silva, Q. C. Rocha and E. F. Lucas, J. Environ. Sci. Health, Part A: Toxic/Hazard. Subst. Environ. Eng., 2016, 51, 634–639 CrossRef CAS PubMed.
- Z. Ziad Ismail, Al-Khawarizmi Eng. J., 2005, 1, 117–124 Search PubMed.
- G. Nazari, H. Abolghasemi and M. Esmaieli, J. Taiwan Inst. Chem. Eng., 2016, 58, 357–365 CrossRef CAS.
- G. Nazari, H. Abolghasemi and M. Esmaieli, Chem. Chem. Technol., 2016, 10, 81–86 CrossRef CAS.
- G. Nazari, H. Abolghasemi, M. Esmaieli and M. Assar, Desalin. Water Treat., 2016, 57, 27339–27348 CrossRef CAS.
- G. Nazari, H. Abolghasemi, M. Esmaieli and E. Sadeghi Pouya, Appl. Surf. Sci., 2016, 375, 144–153 CrossRef CAS.
- H. Zare, G. Najafpour, M. Rahimnejad, A. Tardast and S. Gilani, Bioresour. Technol., 2012, 123, 419–423 CrossRef CAS PubMed.
- M. Zhu, J. Yao, L. Dong and J. Sun, Chemosphere, 2016, 144, 1639–1645 CrossRef CAS PubMed.
- A. Naghizadeh, R. Khosravi, E. Derakhshani, H. Shahabi and F. Ghasemi, Arch. Hyg. Sci., 2015, 4, 187–191 Search PubMed.
- Q. Yu, M. Li, X. Ji, Y. Qiu, Y. Zhu and C. Leng, J. Wuhan Univ. Technol., Mater. Sci. Ed., 2016, 31, 260–268 CrossRef CAS.
- M. Mataji and B. Khoshandam, Chem. Eng. Commun., 2014, 201, 1294–1313 CrossRef CAS.
- A. Alighardashi and S. Shahali, Water Pract. Technol., 2016, 11, 784–795 CrossRef.
- M. M. Taghizadeh and R. Vahdati, Int. J. Biosci., 2015, 6, 375–379 CrossRef.
- S. Ding, L. Jia and Z. Qu, Study on the adsorption of ammonia nitrogen in wastewater
by modified walnut shell, World Automation Congress (WAC), IEEE, Puerto Vallarta, Mexico, 2012, pp. 1–5 Search PubMed.
- J.-X. Guo, L. Fan, J.-F. Peng, J. Chen, H.-Q. Yin and W.-J. Jiang, J. Chem. Technol. Biotechnol., 2014, 89, 1565–1575 CrossRef CAS.
- K. Kuśmierek and A. Świątkowski, Pol. J. Chem. Technol., 2015, 17, 23–31 Search PubMed.
- Q. Yu, M. Li, P. Ning, H. Yi and X. Tang, Sep. Sci. Technol., 2014, 49, 2366–2375 CrossRef CAS.
|
This journal is © The Royal Society of Chemistry 2020 |