DOI:
10.1039/C9RA09477F
(Paper)
RSC Adv., 2020,
10, 8115-8129
Organic template-assisted green synthesis of CoMoO4 nanomaterials for the investigation of energy storage properties†
Received
13th November 2019
, Accepted 14th January 2020
First published on 25th February 2020
Abstract
Transitional metal oxide nanomaterials are considered to be potential electrode materials for supercapacitors. Therefore, in the past few decades, huge efforts have been devoted towards the sustainable synthesis of metal oxide nanomaterials. Herein, we report a synergistic approach to synthesize spherical-shaped CoMoO4 electrode materials using an inorganic–organic template via the hydrothermal route. As per the synthesis strategy, the precursor solution was reacted with the organic compounds of E. cognata to tailor the surface chemistry and morphology of CoMoO4 by organic species. The modified CoMoO4 nanomaterials revealed a particle size of 23 nm by X-ray diffraction. Furthermore, the synthesized material was scrutinized by Fourier transform infrared spectroscopy, X-ray photoelectron spectroscopy, field emission scanning electron microscopy and energy dispersive spectroscopy. The optical band gap energy of 3.6 eV was calculated by a Tauc plot. Gas chromatography-mass spectrometry identified cyclobutanol (C4H8O) and octodrine (C8H19N) as the major stabilizing agents of the CoMoO4 nanomaterial. Finally, it was revealed that the bioorganic framework-derived CoMoO4 electrode exhibited a capacitance of 294 F g−1 by cyclic voltammetry with a maximum energy density of 7.3 W h kg−1 and power density of 7227.525 W kg−1. Consequently, the nanofeatures and organic compounds of E. cognata were found to enhance the electrochemical behaviour of the CoMoO4-fabricated electrode towards supercapacitor applications.
1. Introduction
One key challenge of the present time is the increasing demand of energy storage devices to store renewable energies.1–6 Among such storage systems, supercapacitors (SCs) are the candidates with the most potential owing to their high power density in energy storage devices.5–7 The SCs can efficiently and sustainably fulfill the demands of energy storage as compared to batteries, which are generally of low power density.7–10 SCs are of different types depending on the nature of the electrode material,1,6,9,15–18 and their performances are critically reliant on their electrode material.6,9,15–18 Therefore, in order to achieve high SC performance, an efficient way is to develop electrode materials with tailored and enhanced morphology.19,20 Over recent years, transition metal oxides have been widely investigated as electrode materials.3,6,9–18 The notable advantages of using metal oxides as electrode materials are earth abundance, high theoretical capacity, economical use and ability to be modified in different morphologies and structures.9–14 In particular, constructing and modifying the morphologies as well as the surface chemistry of the metal oxides at the nanoscale level have been highly investigated among the scientific community.8–15 The modified nanostructures of transition metal oxides with carbon and oxygen-containing surface functional groups have greatly enhanced the electrochemical performance of SCs.10–14 Nevertheless, the synthesis of metal oxide nanomaterials with modified surface chemistry has certain limitations such as high cost, large-scale production, sophisticated techniques and complicated synthesis procedures.1,6,7,9,20 Thus, the sustainable synthesis of metal oxide nanomaterials with effective functional groups is still a great challenge in the 21st century.45,48,51,54,59
In this regard, herein, we developed a sustainable synthesis route to synthesize metal oxide nanostructures (CoMoO4) via an inorganic–organic framework. Euphorbia cognata Boiss has been used as a source of organic compounds for the first time. The Euphorbiaceae species are reported to have phytochemicals such as diterpenoids, tannins, flavonoids, taraxerol, terpenoids, lectins, cycloartenol, alkaloids, and neriifolins.74,75 These phytochemicals have been extensively used as reducing and capping agents in the synthesis of nanoparticles.23–28 However, these studies lack an in-depth mechanism and have been unable to identify the incorporated bioactive compounds in the synthesized nanomaterials. Moreover, the reported plant-mediated synthesis is often limited to the mono metal oxides. Therefore, we were motivated to investigate E. cognata for the synthesis of CoMoO4 nanocomposites for the first time. First of all, we have analyzed and identified the organic compounds of the respective plant leaves (shown in S1, S2 and S3†). It revealed different phenolic compounds such as decanoic acid, octodrine, cyclobutanol, D-alanine, and cyclohexylethylamine. Synthetic D-alanine, decanoic acid, and cylcobutanal have been used as fuel and reducing agents in the synthesis of nanomaterials in a number of studies.21,22,71–73 Therefore, the selection of the plant was made on the basis of its significant phytoconstituents that could be used as reducing and stabilizing agents in the synthesis of nanomaterials. Furthermore, to the best of our knowledge, E. cognata has not been previously investigated for the synthesis of nanomaterials.
Plant phytochemicals have been reported as reducing (as well as capping) agents in the synthesis of nanoparticles.23–28 Thus, we believed that E. cognata would induce carbon, oxygen and N-containing organic compounds in the synthesized material. Previously, metal oxides with carbon nanotubes, graphene, and carbon black have been investigated as an outstanding approach for acquiring carbon and oxygen-containing surface functional groups to improve the conductivity and capacitance of the capacitor.10,11,15,16,19,20 Here, we have tailored the surface chemistry of CoMoO4 to introduce C, H, O, N-related functional groups via organic species of E. cognata. We believe that when such functional groups are introduced in the metal oxide nanomaterials, their electronic structure and morphology will be changed, which will create more active sites and quick diffusion pathways. This will lead to better electrical conductivity and an improved redox behaviour of the SC electrode.
2. Materials and methods
Molybdenum(II) acetate (Mo2(O2CCH3)4), cobalt(II) acetate tetrahydrate (C4H6CoO4·4H2O), ethanol (C2H5OH), methanol (CH3OH), ethylene glycol (C2H6O2) and citric acid (C6H8O7) were purchased from Merck Chemicals, Ltd. Double-distilled and deionized water was used throughout the experiment. The phytochemical extract of E. cognata plant leaves was used as reducing and stabilizing agents in the synthesis of CoMoO4 and was sampled from Rawalakot AJK Pakistan. Acetylene black, polyvinylidenedifluoride (PVDF) and N-methyl pyrrolidinone (NMP) were used in the fabrication of the electrode.
2.1. Synthesis of CoMoO4
The organic–inorganic framework-based strategy to synthesize CoMoO4 nanomaterials (Fig. 1) was developed by modifying reported methodologies, particularly relating to metal–organic frameworks (MOFs).19,20,23–28,59,60 250 ml of 20 mM aqueous solutions of C4H6CoO4·4H2O and Mo2(O2CCH3)4 were prepared separately in deionized water. Each solution was subjected to constant magnetic stirring at room temperature until complete dissolution was achieved; afterwards, both solutions were mixed together. In another beaker, 2 g of dried powdered leaves of E. cognata were treated with deionized water on vigorous stirring for 30 minutes at 60 °C to extract organic compounds. After 30 minutes, the extract was cooled down at room temperature and then filtered through Whatman filter paper. Hereinto, 20 ml of the plant organic complex was added into a mixed solution of the precursor on continuous stirring at 70 °C for 2 h, and then incubated at room temperature under dark conditions for 24 hours to attain the phyto-functional groups, and to complete the hydrolysis and precipitation process. Hereafter, the mixture of inorganic metal precursors and organic species of E. cognata was first evaporated at 95 °C overnight. The dried powder was then annealed at 450 °C for 4 hours to procure the CoMoO4 nanomaterial.
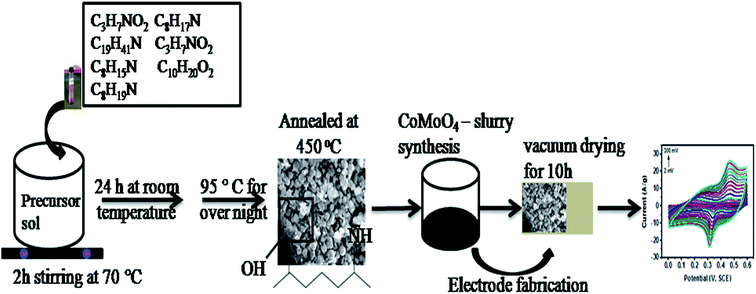 |
| Fig. 1 Schematic diagram of the modified hydrothermal method via organic compounds to synthesize CoMoO4 for fabrication of the electrode. | |
The thermally annealed CoMoO4 nanomaterial was mixed with acetylene black and polyvinylidenedifluoride (PVDF) in an 8
:
1
:
1 ratio in the presence of N-methyl pyrrolidinone (NMP) following reported methodologies.29–32 The prepared slurry was then consistently pasted onto the porous Ni foam and dried at 60 °C under vacuum for 10 h. The mass loading was accurately measured by weighing the nickel foam before and after electrode preparation using an analytical balance.
3. Characterization
The functionalized CoMoO4 was well characterized by ultraviolet-visible spectroscopy (UV-Vis, spectrophotometer 1602, Biomedical services, Spain) for band gap and optical properties. Phyto-stabilizing agents were analyzed by gas chromatography-mass spectroscopy (GC-MS-QP5050, SHIMADZU) and Fourier transforms infrared spectroscopy (FTIR, 8400, Shimadzu, Japan). The phase identification and crystallinity were examined by an XRD5 PANalytical X'Pert Pro. A Quanta 250-FEG scanning electron microscope (FE-SEM) with a Gatan 3View attachment was used to study the morphology and energy-dispersive X-ray spectroscopy (EDX) was used to study the chemical composition of the synthesized CoMoO4. The surface chemistry was investigated by X-ray photoelectron spectroscopy (XPS) using a Kratos Axis Ultra spectrometer with a monochromated Al Kα X-ray source.
3.1. Electrochemical measurements
Electrochemical characterizations of the organic compound stabilized CoMoO4 were carried out using the three-electrode system, where fabricated nickel foam with CoMoO4 was used as the working electrode, a platinum wire was used as the counter electrode and a saturated calomel electrode was used as the reference electrode. All experiments were conducted in 3 M KOH aqueous solution. The electrochemical properties of CoMoO4 were investigated by cyclic voltammetry (CV) at various scan rates (2 to 300 mV s−1), galvanostatic charge–discharge (GCD) at various current densities (0.5–30 A g−1) and electrochemical impedance spectroscopy (EIS) with a frequency range of 50 mHz to 10 kHz.
4. Results and discussion
4.1. Phyto-functionalized CoMoO4 nanomaterial
In order to identify the bioactive organic compounds of the plant extract, the spectroscopic characterization of a prepared extract of E. cognata leaves was carried out and revealed the distinctive peaks as shown in S1,† Fig. 1b. The identified peaks in the ultraviolet and visible regions correspond to plant phenols, as well as flavonoids comprising a benzene ring with the conjugation of ring A and its configurations. The absorbance values at 297.31 nm and 337.81 nm suggest the presence of plant flavonoids having conjugation of rings B and C. The additional absorption bands at 364.10 and 385.96 nm are indicative of flavones and flavonols in the plant extract.33
The dried powdered leaves of E. cognata were subjected to scan at the full frequency range (400 to 4000 cm−1) of FTIR (Fig. 1a, S1†). FTIR illustrated the vibration modes associated with phytochemicals at frequencies (cm−1): 3417.98 (O–H stretching and H bond), 2962.76 (C–H), 2918.40 (C–H), 1639.55 (N–H and –C
C–), 1417.73 (C–C medium stretch (in ring)), 1261.49 (C–H wag (–CH2X) bond, C–N and C–O), 1097.53 (C–N stretch), 1024.24 (C–N stretch), 864.14 (
C–H, N–H wag and C–H oop), 800.49 (
C–H, N–H wag and C–H oop) and 536.2 (C–Br). Therefore, in agreement with the UV analysis of the leaves, the FTIR studies illustrated the alcohol, phenol, aromatic, alkanes, 1° and 2° amines, aliphatic amines, carboxylic acid, esters and ether groups as the organic constituents of the investigated plant leaves.
In order to confirm the possible role of the organic compounds of E. cognata in the synthesis of the nanomaterials, a CoMoO4 pellet was first dried at 95 °C and revealed the presence of associated phytochemicals, as shown in Fig. 2a. Afterwards, CoMoO4 was annealed at 450 °C and subjected to FTIR to identify the functional groups of the incorporated bioactive compounds, as depicted in Fig. 2b. Fig. 2b revealed the presence of a Mo6 and Co34,35 metal bond in the fingerprint region (500 to 400 cm−1), along with major organic compounds of the E. cognata leaves as part of the reducing and stabilizing agents of CoMoO4. Table 1 further explains the organic groups of CoMoO4 corresponding to alcohol and phenol, aldehydes, ester, saturated aliphatics, nitro-compounds (N–O), aliphatic amines, carboxylic acid (O–H bend) and 1°, 2° amines, in agreement with the reported literature.23–26 It is worth noting that after calcinations, vibration peaks at 1576.17 cm−1, 1411.04 cm−1, 674.33 cm−1 and 610.07 cm−1 seemed to disappear (Table 1, Fig. 2b). This is possibly because of the fact that at higher temperatures (450 °C), some organic compounds were degraded/transformed into their metabolites. However, after the annealing process, alcohol, phenol, aldehydes, saturated aliphatics and carboxylic acid were found to be present in the synthesized nanomaterial as stabilizing agents, while a vibrational frequency appeared at 521.12 cm−1 corresponding to the M–O and M–C bonds (M = Co and Mo).
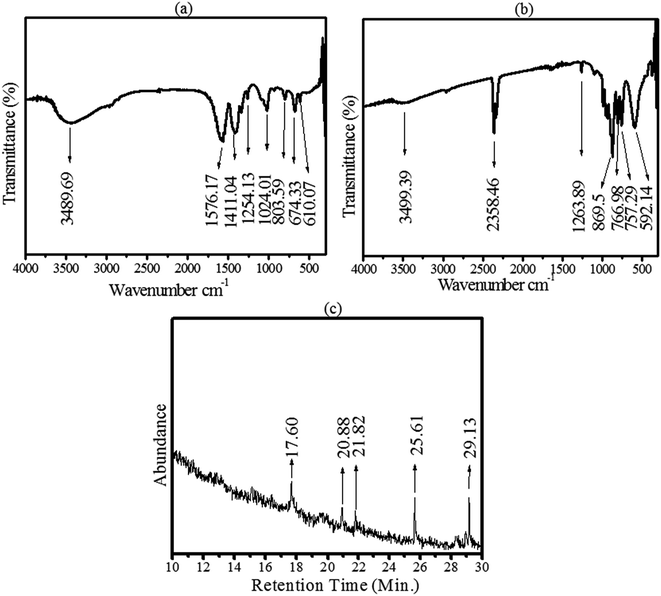 |
| Fig. 2 Identification of organic stabilizing agents of CoMoO4. (a) FTIR spectra of CoMoO4 after drying at 95 °C. (b) FTIR of CoMoO4 after annealing at 450 °C, and (c) GC-MS spectra of CoMoO4. | |
Table 1 Functional groups of organic compounds of CoMoO4 identified by recorded peak frequencies via FTIRa
Peaks (cm−1) after oven drying |
Peaks (cm−1) of annealed sample |
Bond |
Assignments |
Functional groups |
υ = stretching, δ = bending, ρ = rocking, ω = wagging. |
3489.69 |
3499.39 |
O–H |
υ |
Plant phenolic compounds23,24 |
|
2358.46 |
C N |
υ |
Nitrile compounds of E. cognata23,26 |
1576.17 |
|
C–C (in ring) |
υ |
Aromatic ring of phenol and flavonoids of E. cognata24–26 |
1411.04 |
|
C–C (ring) |
υ |
Phenolic compounds of the plant23–26 |
1254.13 |
1263.89 |
C–O |
υ |
Phenol and carboxylic acids of the plant leaves26 |
1024.01 |
|
C–N |
υ |
Plant amines groups of phytocompounds such as C8H19N25,26 |
803.59 |
869.5 |
C–H |
δ |
Hydrocarbon (unsaturated)24–26 |
|
766.98 |
C–H |
ρ |
Alkane (saturated hydrocarbon) from E. cognata phytochemicals23–25 |
|
757.29 |
C–H |
ρ |
Alkane (paraffin) saturated hydrocarbon of the leaves extract24–26 |
674.33 |
|
N–H |
ω |
1°, 2° amines of plant phenols24 |
610.07 |
|
–C C–H:C–H |
δ |
Unsaturated hydrocarbon such as alkynes23–26 |
|
529.14 |
C–M, M–O |
υ |
Metal oxides (Co–O, Mo–O)23–26 |
The organic stabilizing agents were further characterized by GC-MS. The results pertaining to the GC-MS analysis of a methanolic extract of E. cognata identified numerous compounds at their particular retention times (as shown in the annotated mass spectra on S1,† Fig. 3, Table 1, S2 and S3 in the ESI†). The GC-MS profile of E. cognata leaves revealed the presence of octodrine, alanine, cyclobutanol, decanoic acid, azabicyclo, 2-aminodecane and cyclohexylethylamine, as shown in Fig. 1. These bioactive compounds as stabilizing agents were identified in the methanolic suspension of the CoMoO4 nanocomposite as shown in Fig. 2c. The identified peaks in Fig. 2c correspond to cyclobutanol (C4H8O) and octodrine (C8H19N), according to the GC-MS profiling of the NIST library. Therefore, GCMS endorsed the functionalization of CoMoO4 via bioorganic compounds of E. cognata.
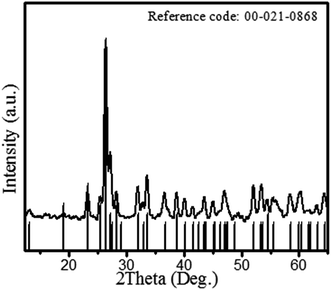 |
| Fig. 3 XRD patterns of the CoMoO4 nanomaterial. | |
Fig. 3 shows the XRD patterns of the bioorganic compound-derived CoMoO4 nanomaterial. The diffraction patterns in Fig. 3 demonstrate well-defined prominent peaks at 2 theta (θ) = 13.2, 19.07, 23.30, 25.45, 26.42, 27.21, 28.32, 29.51, 32.02, 33.59, 36.66, 38.75, 40.13, 41.6, 43.54, 43.99, 45.07, 46.2, 47.05, 48.2, 52.09, 53.47, 54.04, 58.41, 60.42 and 64.35, corresponding to the (001), (−201), (021), (201), (002), (−112), (−311), (310), (−131), (022), (−222), (400), (040), (003), (222), (−422), (−223), (113), (−403), (241), (−133), (−204), (−440), (024), (−424) and (243) hkl planes, respectively. The peak patterns were exactly matched with CoMoO4 of ICSD 00-021-0868. However, a minor shift (less than 0.1%) of the diffraction peaks towards lower diffraction angles was observed for some peaks. This indicates a compression of the crystal lattice due to the stress of organic stabilizing agents. The observed XRD patterns revealed the growth of monoclinic shape CoMoO4 with a space group of C2/m and cell parameters (Å) of a: 10.21, b: 9.268, c: 7.022. The crystallite size of CoMoO4 was calculated from the full width half maximum (FWHM) of the peaks using the following Debye–Scherrer's equation:
D = (0.9 × λ)/(β cos θ) |
where
λ = X-ray wavelength and
β = full width at half maximum intensity of the peak (in Rad). The average particle size of cobalt molybdate was 23.5 nm. It is noted that no individual peak corresponding to Co, Co
3O
4, MoO
3, or Mo
3O
4 was found in
Fig. 3. Therefore, the final product was CoMoO
4 as observed from the peaks pattern of XRD, which was confirmed by EDX (
Fig. 4).
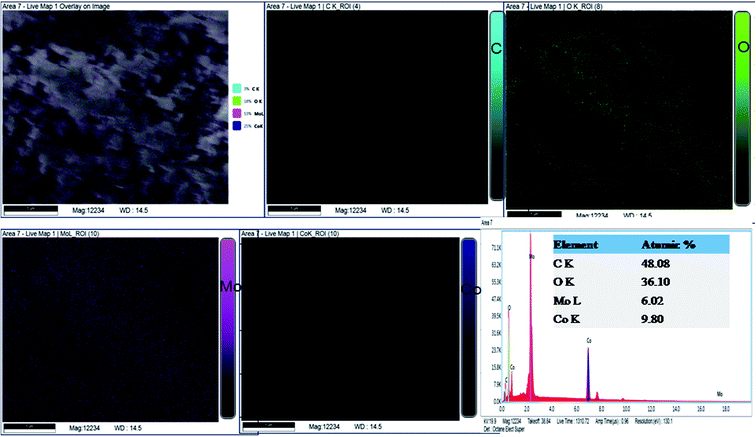 |
| Fig. 4 Elemental analysis of the synthesized nanomaterial using energy dispersive X-ray spectroscopy. | |
The EDX spectrum (Fig. 4) describes the chemical composition of the fabricated material to consist of Co, Mo, O and C. According to the atomic ratios of Co, Mo and O, the nanomaterial is classified as CoMoO4. In agreement with the XRD phase analysis, EDX identified the synthesis of cobalt molybdate with a chemical formula of CoMoO4. Nevertheless, the EDX study demonstrated the presence of a significant atomic percentage of carbon because of the organic functional groups of the stabilizing agents. Continuing with the investigation of the chemical composition, CoMoO4 was subjected to XPS analysis to examine the local bonding environment of the synthesized material and to speculate the functional groups related to the bioactive compounds of E. cognata. The survey scan in Fig. 5a–d shows the composition of surface to be consistent with C, O, Co and Mo. The presence of Mo, Co, O2 and C as major elements of the surface chemistry of the sample is in good agreement with the EDX analysis.
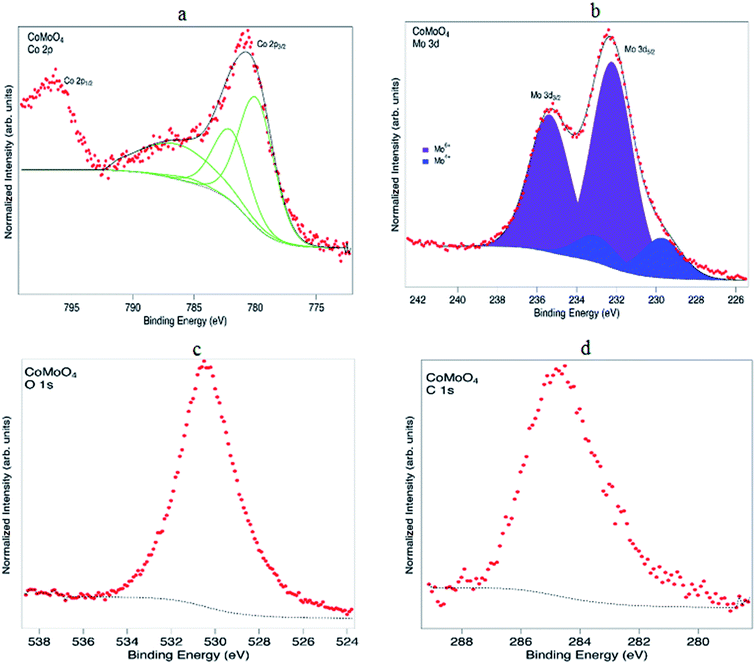 |
| Fig. 5 (a) XPS spectra of Co 2p recorded from CoMoO4, (b) spectra of Mo 3d, (c) XPS spectrum of O 1s and (d) spectrum of C 1s of CoMoO4. | |
Continuing with the investigation of chemical composition, CoMoO4 was subjected to XPS analysis to examine the surface composition of the synthesized material and to verify the presence of organic content of functional groups related to bioactive compounds of E. cognata at the surface. All spectra are calibrated on the binding energy scale relative to the C 1s hydrocarbon peak. The narrow XPS scans in Fig. 5(a–d) show the surface to contain C, O, Co and Mo in agreement with the EDX analysis.
The main Co 2p3/2 peak is rather broad and centred at a binding energy of 780.7, with a broad satellite feature at a binding energy (B.E.) of ∼787 eV. The Co 2p3/2 peak is fitted with multiplet structure for Co2+ ions as detailed by Gupta and Sen37 and Biesinger et al.38 A reasonable agreement is obtained, suggesting the surface Co is mainly in the 2+ state. The peak at 796.6 eV is the Co 2p1/2 peak which arises due to spin-orbit splitting.38 Fig. 5b shows the Mo 3d5/2 and Mo 3d3/2 peaks centred at 232.3 and 235.4 eV, respectively.61 These peaks must be fitted with two doublets at binding energies of 232.3 eV (3d5/2) and 235.4 eV (3d3/2) from Mo6+ in CoMoO4
43 and 229.7 eV (3d5/2) and 233.1 eV (3d3/2) from Mo4+.62 As for carbon and oxygen, for samples that have been exposed to air the information is often difficult to interpret. The C 1s peak at 284.8 eV is associated with C–C and C–H species, which may imply that there are compounds arising from the plant extract on the surface. Unfortunately due to some charging of the sample the region at higher binding energy where C–O species would lie was not recorded. The O 1s shows a main peak at an energy of ∼530 eV, consistent with metal oxides.42,44,63,64 However, as in the case of C, since the sample has been exposed to air there will also be contributions to the O 1s from water and hydroxide species.
FE-SEM images of the bioorganic-assisted CoMoO4 are presented in Fig. 6a–c. The CoMoO4 nanomaterial was examined at different magnifications with a well-defined nanostructure. It can be observed in Fig. 6 that at lower magnifications (10 and 5 μm), the spherical particles arranged themselves in quite uniform and regular structures. Thus, FE-SEM depicted the uniformly distributed spherical-shaped bioorganic-derived CoMoO4 nanomaterials. In order to understand the effects of the bioactive compounds on the morphology of CoMoO4, a blank experiment (also called the sol–gel experiment) was carried out involving citric acid (C6H8O7) and ethylene glycol (C2H6O2) in the ethanol (C2H5OH) solvent. All reducing and gelling agents in the control experiments were of synthetic analytic grade. The details of the controlled experiments are given in the ESI.† It can be observed that the chemical synthesis yielded microstructures (Figure 6d–f), which were larger in size than the organic template-assisted nanomaterial. The ethylene glycol-assisted particles had a nearly cuboidal shape, while the bio-templated particles were spherical in shape. Conversely, the chemically synthesized particles revealed less agglomeration, but with microstructures. The organic framework derived shows little agglomeration at the nanoscale level. Therefore, the bio-template successfully synthesized nanoparticles, while the chemical synthesis yielded prominent microstructures. Moreover, from the present investigation and reported literature,23–25 it was concluded that the phytochemicals of the leaves not only reduced the particle size but also prevented the agglomeration of the particles at the nanoscale.
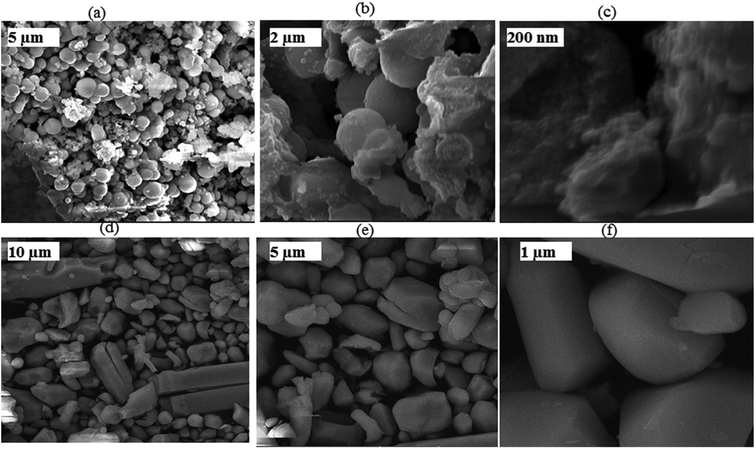 |
| Fig. 6 (a–c) FE-SEM images of the organic template-assisted CoMoO4 at different magnifications. (d–f) FE-SEM images of the controlled sample (ethylene glycol assisted CoMoO4) at different magnifications. | |
4.2. Band gap energy
Fig. 7a shows the absorption spectrum of the fabricated CoMoO4 metal oxides. From Fig. 6a, a wide-range absorption can be observed, which gradually increases towards the lower wavelength with a maximum absorption peak at 275 nm. The maximum absorption band indicates the presence of a blueshift due to the presence of Co oxide, which has a smaller band gap that absorbs the light in a blue shift. However, a small absorption at 379 nm is also indicated in Fig. 7a. Fig. 7b represents the Tauc plot used to determine the optical band gap energy (Eg) of the synthesized material using the following relation:
where α is the absorption coefficient, and hv and Eg are defined as the photon and band gap energies, respectively. As seen in Fig. 7a, the band absorption edge of CoMoO4 was found at 349 nm, corresponding to its band-gap excitation. However, the corresponding band gap energy was 3.6 eV for the synthesized CoMoO4 composite from the Tauc plot.
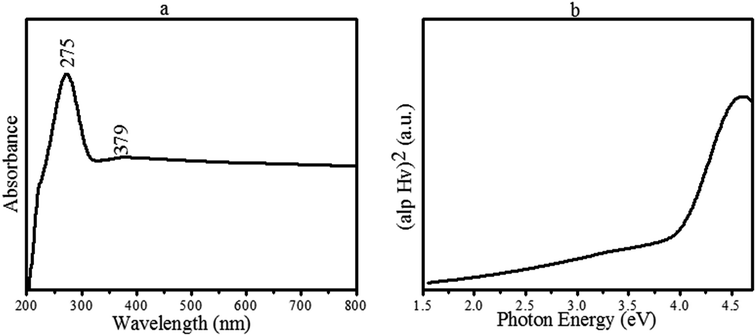 |
| Fig. 7 (a) UV-Vis absorption spectra of CoMoO4, and (b) band gap energy of CoMoO4 via Tauc's plot. | |
5. Supercapacitive studies
The electrochemical properties of the organic compound-derived CoMoO4 were characterized by CV, GCD and EIS. Fig. 8a shows typical voltammograms of the fabricated electrode at scan rates ranging from 2 to 300 mVs−1. It is worth describing here that in agreement with the previous studies,24,29,30 the Ni foam was just used as a mechanical support and current collector in the current investigation. It has no contribution to the capacitance of the electrode because of the negligible integrated area of the CV curves. However, the synthesized CoMoO4 electrode revealed characteristics redox peaks, as shown in Fig. 8a. Li et al. reported that in the CoMoO4 electrode, the Mo species are not involved in the redox reactions, as its role is to enhance the electrical conductivity of the electrode material. Therefore, according to the reported literature,9–12,58 the emergence of the prominent redox peaks of the CoMoO4 electrode are due to the faradaic reactions of M–O and/or M–OH (M = Co), as stated below:
3Co(OH)2 + 2OH− ↔ Co3O4 + 4H2O + 2e− |
Co3O4 + H2O + OH− ↔ 3CoOOH + 2e− |
CoOOH + OH− ↔ CoO2 + H2O + 2e− |
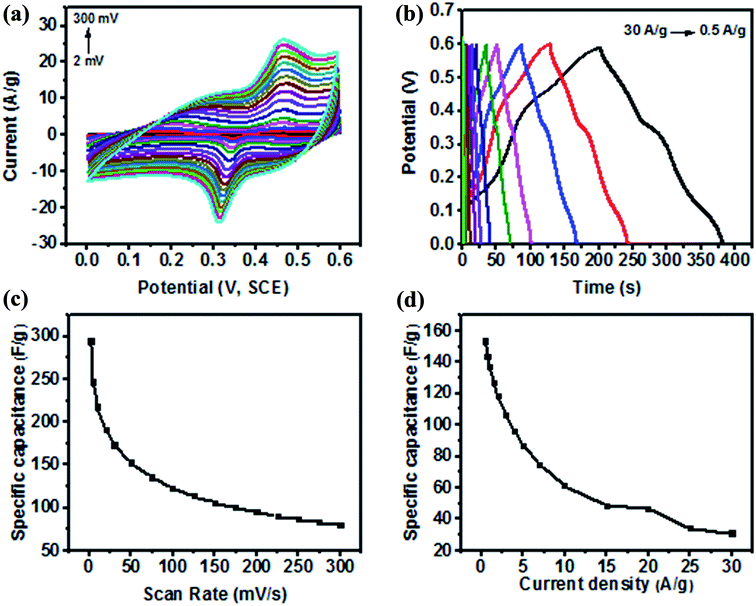 |
| Fig. 8 (a) Cyclic voltammograms of the CoMoO4 electrode at various scan rates. (b) Galvanostatic charge–discharge characteristics of CoMoO4 at various current densities. (c) Variation of the specific capacitance as a function of the scan rate, and (d) variation of the specific capacitance as a function of the applied current density. | |
Moreover, the redox peaks also emerged due to the N-containing groups of the incorporated organic compounds (C8H19N). The organic compounds are reported to have a huge reversible redox potential that makes them interesting candidates for pseudocapacitive energy storage,36 particularly due to the introduction of nitrogen atoms. Pettong et al.,17 Xie et al.15 and Zhou et al.78 reported in their studies that the introduction of nitrogen atoms greatly improved the pseudocapacitance behaviour of electrodes. In the current study, the redox peaks of CoMoO4 were found to exhibit a direct positive correlation with the current density and scan rate. The oxidation reduction peaks were found to be increased with increasing current density and increasing the scan rate from 2 to 300 mV s−1, as observed in Fig. 8a. The potentials of the redox peaks were shifted accordingly in more positive and negative directions with increasing scan rate, creating a wider potential difference (Ec − Ea) at higher potential, under quasi-reversible kinetics.32,34,49,53 This is possibly attributed to the limited ion diffusion rate during the faradaic reactions to satisfy electronic neutralization at higher scan rates.13,14,32 This behaviour of the electrode is associated with a typical battery-type electrode.13,14,32,34 It is further noted that the separation between the redox peaks increased with increasing scan rate, indicating the fast faradaic reactions because of the spherical nanostructure of CoMoO4. According to Zhang et al. and Bhagwan et al., such redox behaviour is due to the ohmic resistance of the electrode.4,41 Therefore, the cyclic voltammetry results indicated the functionalized CoMoO4 as a pseudocapacitor that frequently relied on its redox behaviour as a battery-type electrode. This is because of the presence of Mo, Co, O2, and C at the surface of the electrode as major constituents of the active material as shown by XPS in Fig. 3 and by EDX in Fig. 4. The battery-type behaviour is often associated with most metal oxides as reported by Duraisamy et al., Wang et al., and Hussain et al., where they described such pseudocapacitive behaviours of metal oxide-based electrodes.8,34,53 However, the fabricated CoMoO4 electrode revealed an enhanced peak current density, as well as a larger increment of the integrated area than MoO3,66 MoO2,65 Co,8 Co3O4,18 and Co3O4/CoO34 due to enhanced active sites provided by nanostructures, as well as O and C functional groups. The larger integrated area of the CV curve is significantly important as it reflects the capacitance of the electrode by the following relation:
where
Q is the area under the CV curve, ∂
v/∂
t is the scan rate, Δ
V is the potential window and
m is the mass of the CoMoO
4 on the Ni electrode. As a result, greater the area under redox peaks, higher will be the capacitance.
1,29,30
As shown in Fig. 8c, the fabricated electrode revealed a higher capacitance of 294 F g−1 at 2 mV s−1, while the specific capacitance was observed to decrease with increasing scan rates because of the inaccessibility of the OH− ions of the electrolyte to some parts of the electrode at higher scan rates. Despite this limitation of the electrolyte, a higher capacitance of 122 F g−1 and 80 F g−1 were still achievable at the maximum scan rates of 100 and 300 mV s−1, respectively. This indicated the excellent rate capability (Fig. 8c) of CoMoO4 because of a large number of active sites provided by Mo, Co and the functional groups of the organic species, as well as by spherical-shaped nanoparticles. The results of the present study is in agreement with the previous study of Altin et al.,77 where they reported the favourable electrochemistry (considerable specific capacitance) of different ruthenium complexes with four different organic compounds. They reported that the specific capacitance of ruthenium was increased due to the higher surface areas of the organic compounds.
However, the specific capacitance calculated for CoMoO4 in the current study (even at the highest rate of 300 mV s−1) is much higher than the capacitance of 36 F g−1 of the MoO3 nanorods,66,67 12 F g−1 of Co3O4,47 77 F g−1 of the commercial Co3O4 electrode,18 and 60 F g−1 of the Co electrode8 at the lowest scan rate of 5 mV s−1. Moreover, Table 2 depicts a comparison of the capacitance of the CoMoO4 electrode with previously reported electrodes. The comparison vividly demonstrates that the bioorganic compound-derived electrode revealed a much higher potential for the supercapacitor with an outstanding rate capability because of the combined effects of the surface chemistry and nanoscale morphology analyzed by XPS and FE- SEM, respectively.
Table 2 Comparison of the supercapacitive behaviour of the fabricated nanomaterial with reported investigations by cyclic voltammetry
Electrode |
Electrolyte |
Specific capacitance (F g−1) |
Scan rate (mV s−1) |
References |
CoMoO4@C300 |
KNO3 |
108.1 |
10 |
58 |
ZnCo2O4 nanowire |
PVA/H3PO4 |
0.4 mF |
100 |
46 |
Co3O4/CB |
KOH |
52 |
5 |
47 |
MoO3-coated TiO2 nanotubes with four MoO3 deposition cycles |
KCl |
51.3 |
5 mV s−1 |
68 |
MoO3-coated TiO2 nanotubes with 2 MoO3 deposition cycles |
KCl |
44.9 |
5 mV s−1 |
68 |
Co-doped TiO2 NT/RGO |
Na2SO4 |
34.8 |
5 |
49 |
Pure MoO3 |
KCl |
<10 |
5 mV s−1 |
66 |
CoMoO4 |
3 M KOH |
294 |
2 |
Present work |
123 |
100 |
80.2 |
300 |
The specific capacitance was further calculated from the charge–discharge curve (Fig. 8b) using the following equation:
where
I is the discharge current (A), Δ
t is the discharge time (s), Δ
V is the potential window (V), and
m is the mass (g) of the CoMoO
4 nanocomposites on the electrode. The capacitance of CoMoO
4 at various current densities was studied as shown in
Fig. 8b and d. The GCD of the CoMoO
4 investigations revealed the highest capacitance of 153 F g
−1 at 0.5 A g
−1, and the lowest capacitance of 31 F g
−1 at the highest current density of 30 A g
−1. The calculated capacitance is much greater than the capacitance of 20 F g
−1 of MoO
2,
70 23 F g
−1 of the MoO
3 nanowires,
69 and 17.9 F g
−1 of Co/Co
3O
4 at a lower current density of 1 A g
−1.
56 In agreement with CV, the GCD measurements revealed an enhanced rate capability of CoMoO
4 due to increased active sites offered by organic compounds. The organic molecules as an electrode material revealed more reactive sites, thus revealing the redox stability that can provide a high specific capacity.
80 These results are consistent with those of Peng
et al.,
80 Miroshnikov
et al.,
79 and Xu
et al.,
81 where it was reported that organic compounds (particularly having carbonyl and/or hydroxyl groups) are mostly derived from biomass, and mainly composed of C, H, O, and N elements, which were positively correlated with increasing capacitance, power and energy density. In another study, Fang
et al. revealed the excellent specific capacitance (423 F g
−1 at 0.5 A g
−1) of biomass (betel nut) derived doped carbon. Furthermore,
Table 3 summarizes a comparison of the capacitance of the bioorganic framework-synthesized CoMoO
4 with previous investigations, and revealed the comparatively improved potential of the fabricated electrode for the supercapacitor. Therefore, in agreement with the literature,
1,29,30,55,69,70 the current investigations revealed the higher electrode capacitance at lower current densities and lower capacitance at higher current rates, which indicates a battery-type electrode. Moreover, the CoMoO
4 electrode revealed fast charge and discharge times because of the carbon-based organic stabilizing agents (C
![[double bond, length as m-dash]](https://www.rsc.org/images/entities/char_e001.gif)
O, C–H) in the sample as revealed by XPS. From
Fig. 8b, it can be further observed that the area under the charging and discharging curve is larger at lower current densities and
vice versa. This indicates the fast charging and discharging of CoMoO
4 at 30 A g
−1, and the slowest charging and discharging of the electrode at 0.5 A g
−1. Such inverse correlation between the charge–discharge time and the applied current density proposes that the insufficient faradaic reactions occur due to the poor utilization of the active mass at higher current density.
29–32
Table 3 Comparison of supercapacitive behaviour of CoMoO4 nanomaterial with reported investigations via galvanostatic charge–discharge measurements
Electrode |
Electrolyte |
Specific capacitance (F g−1) |
Current density (A g−1) |
References |
Co-doped TiO2NT/RGO |
Na2SO4 |
27.5 |
0.2 |
49 |
Mo-doped ZnO nanoflakes |
KOH |
46.2 |
10 |
50 |
MnMoO4 |
NaOH |
9.7 |
1 |
65 |
CoO/CNT |
KOH |
17.4 |
0.25 |
52 |
CoMoO4 |
NaOH |
62.8 |
1 |
65 |
MoO3 nanowire |
Na2SO4 |
23 |
0.5 |
69 |
CoMoO4 |
|
153.2 |
0.5 |
Present work |
97 |
5 |
49 |
10 |
Nevertheless, the presented results from the GCD measurements are in good agreement with the CV results; the fabricated electrode depicted pseudo-capacitance behaviour with outstanding redox peaks. To understand the conducting behaviour of the functionalized CoMoO4-based electrodes, EIS measurements were performed for the estimation of the internal resistance and charge transfer resistance. The impedance results are shown in Fig. 9a–c. The Nyquist plot shown in Fig. 9b presented a semicircle arc in the low-frequency region (Rct), and the intercept at the real part in the high frequency range stands for the internal resistance (Ri). Fig. 9a shows a line in the low frequency region called the Warburg element (Zw).1,29,30 It was observed in Fig. 9b that the organic-stabilized CoMoO4 has a smaller intercept of 0.38 Ω at the real axis in the high frequency region. Thus, it has a smaller Ri that is due to the nanostructures, as well as the hydrophilic (oxygen) functional groups of the organic species on the surface, as revealed by XPS. The internal resistance of the CoMoO4 electrodes is smaller than numerous fabricated metal oxide-based electrodes.8–10,16,32,49 Moreover, Miroshnikov et al.79 and Fang et al.76 demonstrated outstanding capacitive behaviour by EIS analysis of organic compounds and biomass-carbon based electrodes, respectively, due to the C, H, N, and O atoms of the electrode material. Therefore, in the current study, the low internal resistance was strongly attributed to the fact that the pathways for electron transport were not only provided by Mo and Co, but also the organic stabilizing agents (C4H8O and C8H19N) of CoMoO4 as indicated by XPS (Fig. 4) and MS spectra (Fig. 2b).
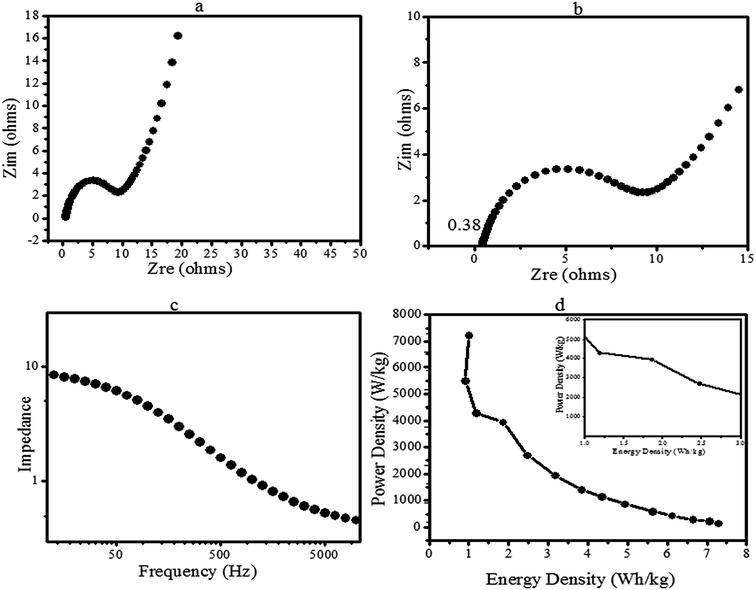 |
| Fig. 9 (a) Nyquist plot of CoMoO4 in the low frequency region, (b) Nyquist plot (semicircle arc) in the high-frequency region, (c) variation of impedance as a function of frequency, and (d) Ragone plot of CoMoO4 (inset depicts the response of the energy density at higher power density). | |
The fabricated electrode has a low charge-transfer resistance (Rct) according to the semicircle arc in the high frequency region. This is due to the faradaic reactions of Co–Mo–O, as stated by Ujjain et al., Xiao et al., and Zequine et al.16,32,49 Thus, Rct confirms the enhanced pseudo-capacitance of the electrode. It can be further observed in the plot of Fig. 9a that there is a clear separation between the semicircle and vertical line of Zw. The plot sharply increased in Fig. 9a and nearly became vertical (more close to 45°), corresponding to the Z-real axis indicating the smaller Warburg impedance. Therefore, the lower Zw confirms the rapid diffusion of ions into the electrolyte and fast adsorption onto the electrode surface due to the surface chemistry and connected nanoparticles (Fig. 6) of CoMoO4.
Fig. 9c presents the total impedance as a function of frequency. The total impedance of the electrode was observed to be decreased in the present investigation, which is consistent with Gervas et al. and Bhoyate et al.29,30 Thus, the lower total impedance further designated the better conductive characteristics of CoMoO4. These results indicated the good electrical conductivity of the fabricated electrode with faradaic reactions. Finally, the storage potential of the fabricated electrode was estimated by the energy density (E) and power density (P) using the discharge voltage via the following equations and are expressed as W h kg−1 and W kg−1:
where
C (F g
−1) is the capacitance
via galvanostatic charge discharge, Δ
V (V) is the potential window and
t (s) is the discharge time.
29,30,56 In the present study, the maximum calculated energy density of CoMoO
4 was 7.3 against a power density of 146, which is remarkably higher than the energy density of MoO
2, MoO
3,
66,69 Co–carbon (1.241 W h kg
−1), and Co
3O
4@Carbon (0.97 W h kg
−1).
56 The phytofabricated CoMoO
4 exhibited an enhanced energy storage potential in comparison with several reported devices.
39,40,49,56,65–70 On the other hand, the maximum recorded power density of CoMoO
4 was 7272.5 W kg
−1 (7.3 kW kg
−1), which is much higher than the power densities of MoO
3,
66,70 Co
3O
4 nanorods,
57 CoO/Co
3O
4,
49 CoO nanofibers
56 and Co
3O
4@Carbon,
56 respectively. The Ragone plot (
Fig. 9d) shows that when the power density increased, the energy density decreased and
vice versa. A negative correlation between the energy density and powder density is a general phenomena associated with numerous other fabricated supercapacitors.
29–32 The inset in the Ragone plot (
Fig. 9d inset) revealed the energy response of CoMoO
4 at the higher power densities. It is worth reporting that, in the current research, the energy density remained at 1.85 W h kg
−1 at the higher power density of 4 kW kg
−1. This lower energy density of CoMoO
4 is still higher than the energy densities of Co@Carbon//Co@Carbon and Co
3O
4@Carbon//Co
3O
4@Carbon.
56 Consequently, in comparison with the previously reported investigation, the energy density and power density strongly suggested the potential of CoMoO
4 as an energy storage device.
Thus, in agreement with the low resistance, outstanding rate capability and efficient redox behaviour, the energy density and power density demonstrated the possibility of the fabricated CoMoO4 electrode as a potential candidate for a supercapacitor.
6. Conclusion
Hereinto, we have successfully developed an inorganic–organic template for the synthesis of nanospherical-shaped cobalt molybdate using bioactive compounds of E. cognata. The obtained organic compound-assisted CoMoO4 not only revealed the stabilization by octodrine and cyclobutanol, but also facilitated the enhanced electro-active sites for energy storage devices. CoMoO4 demonstrated its efficient redox behaviour with a specific capacitance of almost 300 F g−1 in 3 M KOH aqueous solution. Furthermore, significant capacitance values of 123 and 61.2 F g−1 were still retained at a higher scan rate of 100 mV s−1 and current density of 10 A g−1, respectively. Such excellent rate capability has been ascribed to the synergetic effects of the nanostructures and increased active sites created by C and O-related functional groups. In addition, the pathways provided by the hydrophilic functional oxygen groups of the organic stabilizing compounds, have reduced the resistance and enhanced diffusion/transport of electrons. Consequently, the considerable specific capacitance, good rate capability, and enhanced electrochemical conductivity promote the potential application of bioorganic compound-fabricated CoMoO4 nanomaterials for supercapacitors.
Conflicts of interest
The authors declare that they have no known conflict of interest that would influence the work reported in this paper.
Acknowledgements
The authors acknowledge the Higher Education Commission of Pakistan, Department of Environmental Sciences, Fatima Jinnah Women University Rawalpindi Pakistan and the Department of Materials, Photon Science Institute and the Sir Henry Royce Institute, The University of Manchester U.K. The authors express their sincere acknowledgment to the Polymer Chemistry Program and the Kansas Polymer Research Center, Pittsburg State University for providing financial and research support to complete the electrochemical part of this project.
References
- L. Fu, Q. Qu, R. Holze, V. V. Kondratiev and Y. Wu, Composites of metal oxides and intrinsically conducting polymers as supercapacitor electrodes: The best of both worlds?, J. Mater. Chem. A, 2019, 7, 14937–14970 RSC.
- S. Lichušina, L. Staišiūnas, V. Jasulaitienė, A. Selskis and K. Leinartas, Capacitive properties, structure, and composition of porous Co hydroxide/oxide layers formed by dealloying of Zn–Co alloy, J. Appl. Electrochem., 2019, 49(5), 503–515 CrossRef.
- W. Su, R. Miao, B. Tao and F. Miao, High-performance symmetric supercapacitor based on flower-like zinc-cobalt-molybdenum hybrid metal oxide, Ionics, 2019, 1–9 Search PubMed.
- J. Bhagwan, G. Nagaraju, B. Ramulu and J. S. Yu, Promotive Effect of MWCNT on ZnCo2O4 Hexagonal Plates and Their Application in Aqueous Asymmetric Supercapacitor, J. Electrochem. Soc., 2019, 166(2), A217–A224 CrossRef CAS.
- A. Ali, M. Ammar, M. Ali, Z. Yahya, M. Y. Javaid, S. ul Hassan and T. Ahmed, Mo-doped ZnO nanoflakes on Ni-foam for asymmetric supercapacitor applications, RSC Adv., 2019, 9(47), 27432–27438 RSC.
- I. Shakir, M. Shahid, S. Cherevko, C. H. Chung and D. J. Kang, Ultrahigh-energy and stable supercapacitors based on intertwined porous MoO3–MWCNT nanocomposites, Electrochim. Acta, 2011, 58, 76–80 CrossRef CAS.
- Q. Zhang, Z. Liu, B. Zhao, Y. Cheng, L. Zhang, H. H. Wu and Y. Wu, et al., Design and understanding of dendritic mixed-metal hydroxide nanosheets@ N-doped carbon nanotube array electrode for high-performance asymmetric supercapacitors, Energy Storage Materials, 2019, 1(6), 632–645 CrossRef.
- I. Hussain, S. G. Mohamed, A. Ali, N. Abbas, S. M. Ammar and W. Al Zoubi, Uniform growth of Zn-Mn-Co ternary oxide nanoneedles for high-performance energy-storage applications, J. Electroanal. Chem., 2019, 837, 39–47 CrossRef CAS.
- D. Kong, Y. Wang, S. Huang, J. Hu, Y. Von Lim, B. Liu and H. Y. Yang, et al., 3D self-branched zinc-cobalt Oxide@ N-doped carbon hollow nanowall arrays for high-performance asymmetric supercapacitors and oxygen electrocatalysis, Energy Storage Materials, 2019, 23, 653–663 CrossRef.
- C. Chen, S. Wang, X. Luo, W. Gao, G. Huang, Y. Zeng and Z. Zhu, Reduced ZnCo2O4@ NiMoO4.H2O heterostructure electrodes with modulating oxygen vacancies for enhanced aqueous asymmetric supercapacitors, J. Power Sources, 2019, 409, 112–122 CrossRef CAS.
- Y. Liu, X. Cao, L. Cui, Y. Zhong, R. Zheng, D. Wei and J. Liu, et al., Zn-Ni-Co trimetallic carbonate hydroxide nanothorns branched on Cu(OH)2 nanorods array based on Cu foam for high-performance asymmetric supercapacitors, J. Power Sources, 2019, 437, 226897 CrossRef CAS.
- Y. Yang, Y. Zhou, Z. Hu, W. Wang, X. Zhang, L. Qiang and Q. Wang, 3D thin-wall cell structure nickel-cobalt-molybdenum ternary phosphides on carbon cloth as high-performance electrodes for asymmetric supercapacitors, J. Alloys Compd., 2019, 772, 683–692 CrossRef CAS.
- Z. Xu, T. Wang, L. Wang, J. Xu, P. Liu, X. Lan and F. Jiang, et al., Aniline-grafting graphene oxide/polyaniline composite prepared via interfacial polymerization with high capacitive performance, Int. J. Energy Res., 2019, 43(13), 7693–7701 CAS.
- K. D. Poopalam, L. Raghunanan, L. Bouzidi, S. K. Yeong and S. S. Narine, Lipid-derived monoamide as phase change energy storage materials, Int. J. Energy Res., 2019, 43(13), 6934–6950 CAS.
- F. Xie, M. Zhou, G. Wang, Q. Wang, M. Yan and H. Bi, Morphology-dependent electrochemical performance of nitrogen-doped carbon dots@polyaniline hybrids for supercapacitors, Int. J. Energy Res., 2019, 43(13), 7529–7540 CAS.
- S. K. Ujjain, G. Singh and R. K. Sharma, Co3O4@ reduced graphene oxide nanoribbon for high performance asymmetric supercapacitor, Electrochim. Acta, 2015, 169, 276–282 CrossRef CAS.
- T. Pettong, P. Iamprasertkun, A. Krittayavathananon, P. Sukha, P. Sirisinudomkit, A. Seubsai and M. Sawangphruk, et al., High-performance asymmetric supercapacitors of MnCo2O4 nanofibers and N-doped reduced graphene oxide aerogel, ACS Appl. Mater. Interfaces, 2016, 8(49), 34045–34053 CrossRef CAS PubMed.
- W. Du, R. Liu, Y. Jiang, Q. Lu, Y. Fan and F. Gao, Facile synthesis of hollow Co3O4 boxes for high capacity supercapacitor, J. Power Sources, 2013, 227, 101–105 CrossRef CAS.
- S. Wu, J. Liu, H. Wang and H. Yan, A review of performance optimization of MOF-derived metal oxide as electrode materials for supercapacitors, Int. J. Energy Res., 2019, 43(2), 697–716 CrossRef CAS.
- J. Yu, X. Gao, Z. Cui, Y. Jiao, Q. Zhang, H. Dong and L. Dong, et al., Facile Synthesis of Binary Transition Metal Sulfide Tubes Derived from NiCo-MOF-74 for High-Performance Supercapacitors, Energy Technol., 2019, 7(6), 1900018 CrossRef.
- H. A. Abdelgadir and J. Van Staden, Ethnobotany, ethnopharmacology and toxicity of Jatropha curcas L. (Euphorbiaceae): A review, S. Afr. J. Bot., 2013, 88, 204–218 CrossRef CAS.
- S. Mondal, D. Ghosh and K. Ramakrishna, A complete profile on blind-your-eye mangrove Excoecaria agallocha L. (Euphorbiaceae): Ethnobotany, phytochemistry, and pharmacological aspects, Pharmacogn. Rev., 2016, 10(20), 123 CrossRef CAS PubMed.
- W. R. Rolim, M. T. Pelegrino, B. de Araújo Lima, L. S. Ferraz, F. N. Costa, J. S. Bernardes and A. B. Seabra, et al., Green tea extract mediated biogenic synthesis of silver nanoparticles: characterization, cytotoxicity evaluation and antibacterial activity, Appl. Surf. Sci., 2019, 463, 66–74 CrossRef CAS.
- A. Hussain, M. Oves, M. F. Alajmi, I. Hussain, S. Amir, J. Ahmed and I. Ali, et al., Biogenesis of ZnO nanoparticles using Pandanus odorifer leaf extract: anticancer and antimicrobial activities, RSC Adv., 2019, 9(27), 15357–15369 RSC.
- A. Shelar, J. Sangshetti, S. Chakraborti, A. V. Singh, R. Patil and S. Gosavi, Helminthicidal and Larvicidal Potentials of Biogenic Silver Nanoparticles Synthesized from Medicinal Plant Momordica charantia, Med. Chem., 2019, 15(7), 781–789 CrossRef CAS PubMed.
- S. B. Jaffri and K. S. Ahmad, Augmented photocatalytic, antibacterial and antifungal activity of prunosynthetic silver nanoparticles, Artif. Cells, Nanomed., Biotechnol., 2018, 46(1), 127–137 CrossRef CAS PubMed.
- J. P. Mehta, T. Tian, Z. Zeng, G. Divitini, B. M. Connolly, P. A. Midgley and A. E. Wheatley, et al., Sol–Gel synthesis of robust metal–organic frameworks for nanoparticle encapsulation, Adv. Funct. Mater., 2018, 28(8), 1705588 CrossRef.
- D. Gnanasangeetha and D. S. Thambavani, One pot synthesis of zinc oxide nanoparticles via chemical and green method, Res. J. Mater. Sci., 2013, 2320, 6055 Search PubMed.
- C. Gervas, M. D. Khan, S. Mlowe, C. Zhang, C. Zhao, R. K. Gupta and N. Revaprasadu, et al., Synthesis of Off-Stoichiometric CoS Nanoplates from a Molecular Precursor for Efficient H2/O2 Evolution and Supercapacitance, ChemElectroChem, 2019, 6(9), 2560–2569 CrossRef CAS.
- S. Bhoyate, P. K. Kahol, B. Sapkota, S. R. Mishra, F. Perez and R. K. Gupta, Polystyrene activated linear tube carbon nanofiber for durable and high-performance supercapacitors, Surf. Coat. Technol., 2018, 345, 113–122 CrossRef CAS.
- S. Palchoudhury, K. Ramasamy, R. K. Gupta and A. Gupta, Flexible Supercapacitors: A Materials Perspective, Front. Mater., 2019, 5, 83 CrossRef.
- C. Zequine, S. Bhoyate, F. Wang, X. Li, K. Siam, P. K. Kahol and R. K. Gupta, Effect of solvent for tailoring the nanomorphology of multinary CuCo2S4 for overall water splitting and energy storage, J. Alloys Compd., 2019, 784, 1–7 CrossRef CAS.
- J. L. Aleixandre-Tudo and W. Du Toit, The Role of UV-Visible Spectroscopy for Phenolic Compounds Quantification in Winemaking, in Frontiers and New Trends in the Science of Fermented Food and Beverages, IntechOpen, 2018, DOI:10.5772/intechopen.79550.
- E. Duraisamy, H. T. Das, A. S. Sharma and P. Elumalai, Supercapacitor and photocatalytic performances of hydrothermally-derived Co3O4/CoO@ carbon nanocomposite, New J. Chem., 2018, 42(8), 6114–6124 RSC.
- A. Pramanik, S. Maiti, M. Sreemany and S. Mahanty, High electrochemical energy storage in self-assembled nest-like CoO nanofibers with long cycle life, J. Nanopart. Res., 2016, 18(4), 93 CrossRef.
- A. Borenstein, O. Hanna, R. Attias, S. Luski, T. Brousse and D. Aurbach, Carbon-based composite materials for supercapacitor electrodes: a review, J. Mater. Chem. A, 2017, 5(25), 12653–12672 RSC.
- R. P. Gupta and S. K. Sen, Calculation of multiplet structure of core p-vacancy levels, Phys. Rev. B: Solid State, 1974, 10(1), 71–77 CrossRef CAS.
- M. C. Biesinger, B. P. Payne, A. P. Grosvenor, L. W. Lau, A. R. Gerson and R. S. C. Smart, Resolving surface chemical states in XPS analysis of first row transition metals, oxides and hydroxides: Cr, Mn, Fe, Co and Ni, Appl. Surf. Sci., 2011, 257(7), 2717–2730 CrossRef CAS.
- M. S. Yadav, A. K. Sinha and M. N. Singh, Electrochemical behaviour of ZnO–AC based nanocomposite electrode for supercapacitor, Mater. Res. Express, 2018, 5(8), 085503 CrossRef.
- M. S. Yadav, N. Singh and A. Kumar, Synthesis and characterization of zinc oxide nanoparticles and activated charcoal based nanocomposite for supercapacitor electrode application, J. Mater. Sci.: Mater. Electron., 2018, 29(8), 6853–6869 CrossRef CAS.
- C. Zhang, S. Bhoyate, C. Zhao, P. K. Kahol, N. Kostoglou, C. Mitterer and C. Rebholz, et al., Electrodeposited Nanostructured CoFe2O4 for Overall Water Splitting and Supercapacitor Applications, Catalysts, 2019, 9(2), 176 CrossRef.
- S. Oswald, Binding energy referencing for XPS in alkali metal-based battery materials research (I): basic model investigations, Appl. Surf. Sci., 2015, 351, 492–503 CrossRef CAS.
- N. S. McIntyre, D. D. Johnston, L. L. Coatsworth, R. D. Davidson and J. R. Brown, X-ray photoelectron spectroscopic studies of thin film oxides of cobalt and molybdenum, Surf. Interface Anal., 1990, 15(4), 265–272 CrossRef CAS.
- R. Lindsay and G. Thornton, Probing well-characterized metal oxide surfaces with synchrotron radiation, J. Phys.: Condens. Matter, 2001, 13(49), 11 CrossRef.
- H. Wu, Z. Lou, H. Yang and G. Shen, A flexible spiral-type supercapacitor based on ZnCo 2 O 4 nanorod electrodes, Nanoscale, 2015, 7(5), 1921–1926 RSC.
- B. Liu, D. Tan, X. Wang, D. Chen and G. Shen, Flexible, Planar-Integrated, All-Solid-State Fiber Supercapacitors with an Enhanced Distributed-Capacitance Effect, Small, 2013, 9(11), 1998–2004 CrossRef CAS PubMed.
- W. Zhou, J. Liu, T. Chen, K. S. Tan, X. Jia, Z. Luo and T. Yu, et al., Fabrication of Co3O4-reduced graphene oxide scrolls for high-performance supercapacitor electrodes, Phys. Chem. Chem. Phys., 2011, 13(32), 14462–14465 RSC.
- L. S. Aravinda, K. K. Nagaraja, H. S. Nagaraja, K. U. Bhat and B. R. Bhat, ZnO/carbon nanotube nanocomposite for high energy density supercapacitors, Electrochim. Acta, 2013, 95, 119–124 CrossRef CAS.
- H. Xiao, W. Guo, B. Sun, M. Pei and G. Zhou, Mesoporous TiO2 and Co-doped TiO2 nanotubes/reduced graphene oxide composites as electrodes for supercapacitors, Electrochim. Acta, 2016, 190, 104–117 CrossRef CAS.
- A. Ali, M. Ammar, M. Ali, Z. Yahya, M. Y. Javaid, S. ul Hassan and T. Ahmed, Mo-doped ZnO nanoflakes on Ni-foam for asymmetric supercapacitor applications, RSC Adv., 2019, 9(47), 27432–27438 RSC.
- J. S. Suroshe and S. S. Garje, Capacitive behaviour of functionalized carbon nanotube/ZnO composites coated on a glassy carbon electrode, J. Mater. Chem. A, 2015, 3(30), 15650–15660 RSC.
- Y. G. Zhu, Y. Wang, Y. Shi, J. I. Wong and H. Y. Yang, CoO nanoflowers woven by CNT network for high energy density flexible micro-supercapacitor, Nano Energy, 2014, 3, 46–54 CrossRef CAS.
- H. Wang, C. Shen, J. Liu, W. Zhang and S. Yao, Three-dimensional MnCo2O4/graphene composites for supercapacitor with promising electrochemical properties, J. Alloys Compd., 2019, 792, 122–129 CrossRef CAS.
- Z. Li, Z. Zhou, G. Yun, K. Shi, X. Lv and B. Yang, High-performance solid-state supercapacitors based on graphene-ZnO hybrid nanocomposites, Nanoscale Res. Lett., 2013, 8(1), 473 CrossRef PubMed.
- K. Subramani and M. Sathish, Facile synthesis of ZnO nanoflowers/reduced graphene oxide nanocomposite using zinc hexacyanoferrate for supercapacitor applications, Mater. Lett., 2019, 236, 424–427 CrossRef CAS.
- E. Dai, J. Xu, J. Qiu, S. Liu, P. Chen and Y. Liu, Co@ Carbon and Co3O4@ Carbon nanocomposites derived from a single MOF for supercapacitors, Sci. Rep., 2017, 7(1), 12588 CrossRef PubMed.
- T. Meng, Q. Q. Xu, Z. H. Wang, Y. T. Li, Z. M. Gao, X. Y. Xing and T. Z. Ren, Co3O4 nanorods with self-assembled nanoparticles in queue for supercapacitor, Electrochim. Acta, 2015, 180, 104–111 CrossRef CAS.
- J. Li, C. Zhao, Y. Yang, C. Li, T. Hollenkamp, N. Burke and W. Chen, et al., Synthesis of monodispersed CoMoO4 nanoclusters on the ordered mesoporous carbons for environment-friendly supercapacitors, J. Alloys Compd., 2019, 810, 151841 CrossRef CAS.
- T. Prabhakaran and J. Hemalatha, Chemical control on the size and properties of nano NiFe2O4 synthesized by sol–gel autocombustion method, Ceram. Int., 2014, 40(2), 3315–3324 CrossRef CAS.
- C. C. Vidyasagar, Y. A. Naik, T. G. Venkatesha and P. Manjunatha, Sol–gel synthesis using glacial acetic acid and optical properties of anatase Cu–TiO2 nanoparticles, J. Nanoeng. Nanomanuf., 2012, 2(1), 91–98 CrossRef CAS.
- A. Habibi-Yangjeh, M. Mousavi and K. Nakata, Boosting visible-light photocatalytic performance of g-C3N4/Fe3O4 anchored with CoMoO4 nanoparticles: novel magnetically recoverable photocatalysts, J. Photochem. Photobiol., A, 2019, 368, 120–136 CrossRef CAS.
- P. C. Kao, C. J. Hsieh, Z. H. Chen and S. H. Chen, Improvement of MoO3/Ag/MoO3 multilayer transparent electrodes for organic solar cells by using UV–ozone treated MoO3 layer, Sol. Energy Mater. Sol. Cells, 2018, 186, 131–141 CrossRef CAS.
- M. Abu-Samha, K. J. Børve, M. Winkler, J. Harnes, L. J. Saethre, A. Lindblad and S. Svensson, et al., The local structure of small water clusters: imprints on the core-level photoelectron spectrum, J. Phys. B: At., Mol. Opt. Phys., 2009, 42(6), 069801 CrossRef.
- A. Furlan, J. Lu, L. Hultman, U. Jansson and M. Magnuson, Crystallization characteristics and chemical bonding properties of nickel carbide thin film nanocomposites, J. Phys.: Condens. Matter, 2014, 26(41), 415501 CrossRef PubMed.
- L. Q. Mai, F. Yang, Y. L. Zhao, X. Xu, L. Xu and Y. Z. Luo, Hierarchical MnMoO 4/CoMoO 4 heterostructured nanowires with enhanced supercapacitor performance, Nat. Commun., 2011, 2, 381 CrossRef PubMed.
- T. Tao, Q. Chen, H. Hu and Y. Chen, MoO3 nanoparticles distributed uniformly in carbon matrix for supercapacitor applications, Mater. Lett., 2012, 66(1), 102–105 CrossRef CAS.
- W. Q. Tang, J. Y. Xu and Z. Y. Gu, Metal–Organic-Framework-based Gas Chromatographic Separation, Chem.–Asian J., 2019, 14(20), 3462–3473 CrossRef CAS PubMed.
- D. Guan, X. Gao, J. Li and C. Yuan, Enhanced capacitive performance of TiO2 nanotubes with molybdenum oxide coating, Appl. Surf. Sci., 2014, 300, 165–170 CrossRef CAS.
- I. Shakir, M. Shahid, S. Cherevko, C. H. Chung and D. J. Kang, Ultrahigh-energy and stable supercapacitors based on intertwined porous MoO3–MWCNT nanocomposites, Electrochim. Acta, 2011, 58, 76–80 CrossRef CAS.
- W. Shaheen, M. F. Warsi, M. Shahid, M. A. Khan, M. Asghar, Z. Ali and I. Shakir, et al., Carbon coated MoO3 nanowires/graphene oxide ternary nanocomposite for high-performance supercapacitors, Electrochim. Acta, 2016, 219, 330–338 CrossRef CAS.
- L. Chauhan, A. K. Shukla and K. Sreenivas, Dielectric and magnetic properties of Nickel ferrite ceramics using crystalline powders derived from DL alanine fuel in sol–gel auto-combustion, Ceram. Int., 2015, 41(7), 8341–8351 CrossRef CAS.
- V. Catalani, M. Prilutskaya, A. Al-Imam, S. Marrinan, Y. Elgharably, M. Zloh and O. Corazza, et al., Octodrine: new questions and challenges in sport supplements, Brain Sci., 2018, 8(2), 34 CrossRef PubMed.
- P. A. Cohen, J. C. Travis, P. H. Keizers, P. Deuster and B. J. Venhuis, Four experimental stimulants found in sports and weight loss supplements: 2-amino-6-methylheptane (octodrine), 1, 4-dimethylamylamine (1, 4-DMAA), 1, 3-dimethylamylamine (1, 3-DMAA) and 1, 3-dimethylbutylamine (1, 3-DMBA), Clin. Toxicol., 2018, 56(6), 421–426 CrossRef CAS PubMed.
- P. Y. Mali and S. S. Panchal, Euphorbia neriifolia L.: Review on botany, ethnomedicinal uses, phytochemistry and biological activities, Asian Pac. J. Trop. Med., 2017, 10(5), 430–438 CrossRef CAS PubMed.
- S. Mondal, D. Ghosh and K. Ramakrishna, A complete profile on blind-your-eye mangrove Excoecaria agallocha L. (Euphorbiaceae): ethnobotany, phytochemistry, and pharmacological aspects, Pharmacogn. Rev., 2016, 10(20), 123 CrossRef CAS PubMed.
- J. Fang, D. Guo, C. Kang, S. Wan, S. Li, L. Fu and Q. Liu, et al., Enhanced hetero-elements doping content in biomass waste-derived carbon for high performance supercapacitor, Int. J. Energy Res., 2019, 43(14), 8811–8821 CAS.
- S. Altin, E. Öz, S. Altundağ, A. Bayri, T. Roisnel, V. Dorcet and S. Yaşar, et al., Investigation of hybrid-capacitor properties of ruthenium complexes, Int. J. Energy Res., 2019, 43(13), 6840–6851 CAS.
- C. Zhou, X. Yan, J. Wang, X. Yuan, D. Wang, Y. Zhu and X. Cheng, Mn3O4 nanoparticles on activated carbonitride by soft chemical method for symmetric coin cell supercapacitors, Int. J. Energy Res., 2019, 43(14), 8481–8491 CAS.
- M. Miroshnikov, K. Kato, G. Babu, K. P. Divya, L. M. R. Arava, P. M. Ajayan and G. John, A common tattoo chemical for energy storage: henna plant-derived naphthoquinone dimer as a green and sustainable cathode material for Li-ion batteries, RSC Adv., 2018, 8(3), 1576–1582 RSC.
- H. Peng, Q. Yu, S. Wang, J. Kim, A. E. Rowan, A. K. Nanjundan and J. Yu, et al., Molecular design strategies for electrochemical behavior of aromatic carbonyl compounds in organic and aqueous electrolytes, Adv. Sci., 2019, 6(17), 1900431 CrossRef PubMed.
- Y. Xu, M. Zhou and Y. Lei, Organic materials for rechargeable sodium-ion batteries, Mater. Today, 2018, 21(1), 60–78 CrossRef CAS.
Footnote |
† Electronic supplementary information (ESI) available. See DOI: 10.1039/c9ra09477f |
|
This journal is © The Royal Society of Chemistry 2020 |
Click here to see how this site uses Cookies. View our privacy policy here.