DOI:
10.1039/C9RA09225K
(Paper)
RSC Adv., 2020,
10, 1829-1837
Benzyl isothiocyanate fumigation inhibits growth, membrane integrity and mycotoxin production in Alternaria alternata†
Received
7th November 2019
, Accepted 30th December 2019
First published on 8th January 2020
Abstract
The antifungal activity of benzyl isothiocyanate (BITC) against pear pathotype-Alternaria alternata, the causal agent of pear black spot, and its possible mechanisms were studied. The results indicated that both the spore germination and mycelial growth of A. alternata were significantly inhibited by BITC in a dose-dependent manner. BITC concentrations at 1.25 mM completely suppressed mycelial growth of A. alternata and prevented ≥50% of black spot development in wounded pears inoculated with A. alternata. Microscopic analyses and propidium iodide (PI) staining showed that spore morphology in A. alternata treated with BITC at 0.625 mM was severely damaged. Relative electrical conductivity and lysis ability assays further showed that BITC treatment destroyed the integrity of the plasma membrane. Additionally, mycotoxin production was inhibited by 0.312 mM BITC, and the inhibitory rates of alternariol monomethyl ether (AME), alternariol (AOH), altenuene (ALT) and tentoxin (TEN) were 89.36%, 84.57%, 91.41% and 67.78%, respectively. The above results suggest that BITC exerts antifungal activity through membrane-targeted mechanisms.
1. Introduction
Alternaria sp. is a common genus of ascomycete fungi that are both saprophytic on organic materials and pathogenic to many plants.1 Infection by Alternaria sp. leads to black spot rot of fresh fruits and vegetables, including pears, citruses, apricots, cherry tomatoes and blueberries. During the development and storage, black spots in pears caused by Alternaria alternata is one of the major postharvest diseases leading to fruit deterioration and rotting, which results in economic losses.2–5 A. alternata is a dematiaceous fungus, characterized by dark colonies ranging from grey to olive/brown.6 Microscopic characteristics of A. alternata conidia were ovoid or elliptical, with one to five transverse septa and none or three longitudinal septum. When the conidia adhere to the surface of the fruit, they germinate from the top and partially form the infectious hyphae which colonize the fruit cells and tissues, thereby capturing the nutrients and water of the host, and also producing harmful metabolites such as mycotoxins.7 The necrotrophic pathogen A. alternata in citrus exerts pathogenesis through the production of host-specific toxins.8 The most common host-specific toxins of Alternaria sp. in foods items are alternariol monomethyl ether (AME), alternariol (AOH), altenuene (ALT) and tentoxin (TEN),9–11 which accumulate in fruits and processed food, leading to food safety issues and a threat to human health.1,12 Currently, chemical fungicides have been extensively used to a primary means of combating black spot rot.13 In addition to its antifungal activity, inhibiting mycotoxin synthesis and reducing mycotoxin levels are major methods to control the postharvest decay of vegetables and fruits.14 However, pathogen resistance to various types of fungicides can develop after frequent usage. In addition, synthetic fungicides are not friendly for environment and human health issues.15 Accordingly, natural antifungal products require development to meet environmentally friendly health demands.
Isothiocyanates (ITCs) are naturally-occurring constituents that bear a wide biocidal spectrum, with potential as postharvest fumigants.16–19 ITCs exhibit biocidal activity against fungi,20,21 bacteria,22 insects and nematodes.23–25 ITCs vary according to the R-side groups on the parent glucosinolate, which can be aliphatic, aromatic or heteroaromatic.26 Aromatic ITCs are more antifungal than ITCs with aliphatic R groups,27 and aromatic BITC show higher biocidal activity against Sclerotinia sclerotiorum and Rhizoctonia solani compared to ITCs derived from the aliphatic allyl-isothiocyanate and aromatic p-hydroxybenzyl.28 BITC treatment of low-density polyethylene film (LDPF) bags is an active control strategy for Alternaria rot in tomato fruit.29 BITC also shows potent inhibitory effects against Fusarium culmorum and Staphylococcus aureus among the several isothiocyanate flavors separately treated.30,31 However, the mechanisms by which BITC inhibits fungal activity have not been defined.
The cell membrane is the major target of toxic lipophilic compounds in eukaryotic cells.32 BITC is lipophilic and therefore, it may reacts with enzymes present at the plasma membrane, causing fungal growth inhibition and cell death.29 In addition, mycotoxin production during fungal growth might interact with ITCs after membrane destruction, reducing the levels of aflatoxins produced by Aspergillus parasiticus CECT 2681 in nuts (peanut, cashew, almond, hazelnut and pistachio).33 I. Clemente et al. used active packaging containing BITC to reduce or eliminate ochratoxin production.34 However, M. Mari et al. found that benzyl isothiocyanate showed little or no activity against the pathogens tested on Botrytis cinerea and R. stolonifera.35 There are few studies focusing on the growth, membrane integrity and related mycotoxin production of A. alternata by BITC fumigated. In this study, we evaluated the antifungal activity of BITC against A. alternata through fumigation and in vitro/in vivo assessments. The effects of BITC on cell membrane integrity and mycotoxin production of A. alternata were also investigated.
2. Materials and methods
2.1 Chemicals
Standard benzyl isothiocyanate (CAS: 622-78-6) was purchased by the Tokyo Chemical Industry (Shanghai). BITC was reagent-grade with 99.9% purity and dissolved in 20% ethanol-sterile distilled water (v/v) and then filtrated through a 0.22 μm microporous membrane.
2.2 Fruit and pathogen
‘Zaosu’ pears (Pyrus bretschneideri Rehd) free of physical injury or infection were commercially harvested at the Tiaoshan Farm in Jingtai County, Gansu Province, China, and individually packed and stored at 4 °C cold storage at the laboratory. Pear fruits were used for in vivo assessments within a week.
A. alternata (JT03) was obtained from the post-harvest laboratory at Gansu Agricultural University, Gansu Province, China. The spores were harvested from 6 day-old PDA cultivates and suspended in 20 mL of sterile distilled water. Suspensions were followed by filtration through four layers of sterile gauze. The number of spores (1 × 105 or 1 × 106 spores per mL) were determined using hemocytometer for in vivo and in vitro testing.
2.3 Monitoring spore germination
The 20 μL A. alternata spore suspensions (1 × 105 spores per mL) were added to an agar plugs (diameter = 5 mm) (in triplicate) and placed onto a glass slides. Then the slides were placed in a glass Petri dish (diameter = 18 cm) with the same size of moist filter paper at the bottom to provide with 90–92% relative humidity. Sterile filter paper discs (diameter = 6 mm) containing BITC (0, 0.039, 0.078, 0.156, 0.312, 0.625, and 1.25 mM) were placed on the surface of the Petri dish and incubated at 28 °C for 2, 4, 6 and 8 hours. The cultures were taken at the corresponding time point, and stained with 20 μL of lactophenol cotton blue for 2 minutes. And 100 spores were counted under a microscope to determine germination rates. Each BITC concentration was assessed in triplicate.
2.4 Mycelial growth assays
The effects of BITC on the mycelial growth of A. alternata were assessed on PDA plates according to the methods described by N. L. Tatsadjieu et al.36 Autoclaved PDA medium which was cooled to about 60 °C was poured into Petri dishes (diameter = 90 mm). Sterile filter paper discs (diameter = 6 mm) containing various concentrations of BITC (0, 0.039, 0.078, 0.156, 0.312, 0.625, and 1.25 mM) were placed on the surface of the Petri dish. A 6 mm plug of mycelial agar was obtained from the edge of 6 day-old cultures of A. alternata and transferred into the center of each dish. Plates were sealed with parafilm and the radial growth of A. alternata was measured after incubation at 28 °C for 3, 5 and 7 day. Each treatment was applied to three replicates and the entire experiment was repeated three.
2.5 In vivo antifungal activity assays
The in vivo assays were performed as previously described with subtle modifications.37–39 Pears lacking physical injury or infections were selected based on size uniformity, surface-disinfected with 1% (v/v) sodium hypochlorite for 2 min, rinsed with tap water, and air-dried prior to use. Pears fruits were wounded (3 mm wide and 3 mm deep) with a sterile dissecting needle at the equator prior to inoculation. Each wound site was inoculated with 20 μL 1 × 105 spores per mL A. alternata and air dried at room temperature. Selected pears were placed in sealed boxes and fumigated with BITC (0, 0.312, 0.625, 1.25 mM) for 12 h (concentrations were selected based on preliminary in vitro experiments), and then stored at room temperature (25 ± 2 °C), 90–92% relative humidity for 9 days. The lesion diameter was measured every 2 days. There were three replicates with 10 fruits in each treatment, and the experiment was repeated three times.
2.6 Determination of membrane integrity
PI staining was performed as previously described with minor modifications.40 A. alternata spores were cultured on PDA for 6 day and fumigated with BITC at 0, 0.156, 0.312 and 0.625 mM. Spores were harvested from PDA and suspended in 20 mL of 100 mM PBS (pH 7.4). After incubation of A. alternata spores for 4 hours, PI was added to a final concentration at 2.5 μg mL−1 and maintained at 28 °C for 10 minutes in the dark. Mixtures were centrifuged at 8000 × g for 2 min at 4 °C and precipitates were washed twice in PBS to remove residual dye. Samples were then resuspended in 500 μL of PBS and then 10 μL suspensions were dropped on slides. Unstained spore suspensions were used as controls. Samples were imaged under a fluorescence microscope (U-LH100HG 19v 100w, Tokyo 163-0914, Japan). The percentages of fluorescent spores in each population were calculated. Each experiment was performed in triplicate and the experiment was repeated three.
2.7 Cytoplasmic leakage assays
The leakage of cytoplasmic contents from A. alternata mycelia were determined according to the methods of J. Tian et al. and D. R. N. A. Cezar et al. with minor modifications.41,42 A total of 6–8 plugs of A. alternata mycelial agar (diameter = 6 mm) fumigated with BITC at 0, 0.312 and 0.625 mM were obtained from the edge of 6 day-old cultures on PDA and transferred into PDB medium at 28 °C on a rotary shaker (150 rpm). After 3 days of incubation, mycelia were harvested onto filter paper (pore diameter 10 μm) and washed with sterile distilled water. Mycelia was resuspended in 50 mL sterile distilled water and incubated on a rotary shaker at 26 °C for 0, 30, 60, 90 and 120 min. Cell membrane permeability was determined using an electric conductivity meter (DDS-307A, Shanghai, China). Nucleic acids levels were measured through the assessment of optical density at 260 nm (OD260). All experiments were performed in triplicate.
2.8 Extraction of mycotoxins
2.8.1 Standards. Certified standards of Alternaria toxins, namely AME, AOH, ALT and TEN were purchased from Shanghai Yuanye Bio-Technology Co., Ltd (China). Solid portions of standards were dissolved in MeCN to prepare 100 μg mL−1 stock solutions, and a portion of solutions were prepared from the stock standard solutions at 10 μg mL−1 in MeCN, which were stored in the dark at −20 °C. The spiking solution (1 μg mL−1) was freshly prepared from the mix solutions, and the working solutions with concentrations of 1–200 ng mL−1 were freshly prepared just before using with the blank matrix.
2.8.2 Sample preparation. The method have been appropriately modified as described by P. López et al.9 The fungus were cultured in PDA for 4 day at 28 °C and fumigated with BITC at 0 and 0.312 mM according to previous experiment. A. alternata mycelium (0.5 g) was grounded in an ice bath and the hyphae were transferred to sterile centrifuge tubes containing 2.5 mL of acetonitrile/water (80
:
20) and 0.3% formic acid. Extracts were sonicated for 60 min and agitated in a shaker agitator for 30 min at 28 °C and 150 rpm. Next, 0.25 g of anhydrous MgSO4 and 0.04 g NaCl were added while shaking for 1 min, and samples were centrifuged at 10
000 rpm for 10 min. Supernatant was passed through a 0.22 μm organic filter to a volume of 1.2 mL for high performance liquid chromatography-tandem mass spectrometry (parameters described in Table 1; standard curve for standard samples in Fig. S1†).
Table 1 Optimized LC-MS/MS instrumental parametersa
Analyte |
Ionization mode |
Retention time (min) |
MRM (m/z, positive) |
Fragmentor |
Collision energy |
MRM: (Q) transition used for quantification and (I) transition employed to confirm the identification. |
Alternariol monomethyl ether (AME) |
ESI− |
2.85 |
271.0 → 228.0 (Q) |
32 |
20 |
271.0 → 256.0 (I) |
Alternariol (AOH) |
ESI− |
2.37 |
257.0 → 147.2 (Q) |
32 |
20 |
257.0 → 213.0 (I) |
Allenuene (ALT) |
ESI+ |
3.33 |
293.1 → 239.1 (Q) |
85 |
15 |
293.1 → 257.2 (I) |
Tentoxin (Ten) |
ESI+ |
3.66 |
415.2 → 189.0 (Q) |
110 |
30 |
415.2 → 312.3 (I) |
2.9 Statistical analysis
Experiments were performed on three independent occasions and each replicate was performed in triplicate. Data was statistically analyzed using an analysis of variance (ANOVA) and expressed as the mean ± standard deviation (SD). Mean separations were analyzed using Duncan's multiple range tests and differences amongst the different treatments were determined at the 5% level (IBM SPSS Statistics 20, USA).
3. Results
3.1 BITC inhibited spore germination and mycelial growth of A. alternata
BITC treatment effectively inhibited spore germination and the mycelial growth of A. alternata in a dose-dependent manner (Fig. 1A and B). Spore germination rates of 0.625 mM BITC fumigated A. alternata decreased by 82.89% compared to that of the control (p < 0.05). When the concentration of BITC was increased to 1.25 mM, spores germination were entirely inhibited (Fig. 1A). Visible hyphae of 0.625 mM BITC treated A. alternata was observed after 2 days of cultivation (Fig. 1B), and the inhibition rate of mycelial growth was up to 41.05% (p < 0.05) 4 days after treatment. BITC at 1.25 mM completely inhibited mycelial growth. Colony morphologies of the treated groups showed a more pronounced and regular round shape (Fig. 1C).
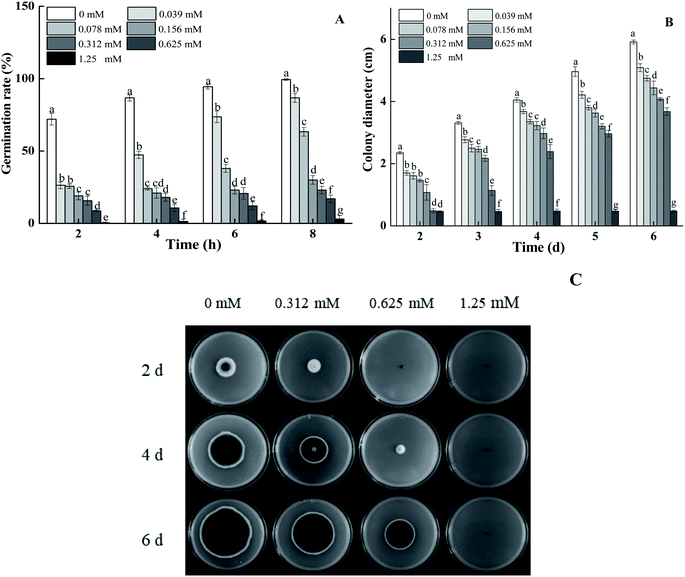 |
| Fig. 1 Effects of BITC on spore germination rates (A), the mycelial diameter (B) and colony morphology (C) of A. alternata after treatment with 0.312, 0.625 and 1.25 mM BITC. Vertical bars represent standard errors. Columns marked by different letters (such as a and b) indicate that there are significant differences in the treatment within the group, and identical letters (such as c and d) indicate no significant difference in treatment within the group according to Duncan's multiple range tests (p < 0.05). | |
3.2 Efficacy of BITC against A. alternata in pears
As shown in Fig. 2, the development of black spots in pears inoculated with A. alternata was effectively inhibited by BITC fumigation. The lesion diameters of the black spots in pears treated with 0.625 and 1.25 mM BITC were reduced by 34.42% and 52.83% (p < 0.05) compared to control pears after 7 days of inoculation, respectively. BITC concentrations of 1.25 mM showed the highest level of disease control (Fig. 2A). Dark brown infected areas were observed on 1.25 mM BITC fumigated pear fruits (Fig. 2B).
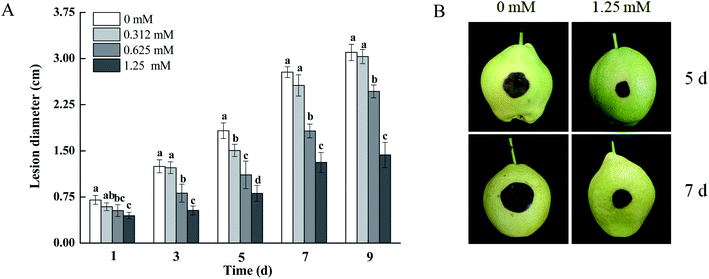 |
| Fig. 2 Harvested pears were treated with BITC at 0.312, 0.625 and 1.25 mM and inoculated with A. alternata and stored at 25 °C. Lesion diameter (A) was measured and imaged (B) every two days post inoculation. Vertical bars represent the standard error of treatment means. Columns marked by differed lowercase letters are significantly different according to Duncan's multiple range tests (p < 0.05). | |
3.3 Cell membrane integrity by PI staining
PI staining was performed for A. alternata cells in the presence and absence of BITC. As shown in Fig. 3A, BITC treatment led to a loss of cell membrane integrity of A. alternata in a concentration dependent manner, evidenced by the increased intensity of PI staining. The percentage of spores with PI fluorescence which impaired membrane integrity was as high as 90% in the presence of 0.625 mM BITC (Fig. 3B).
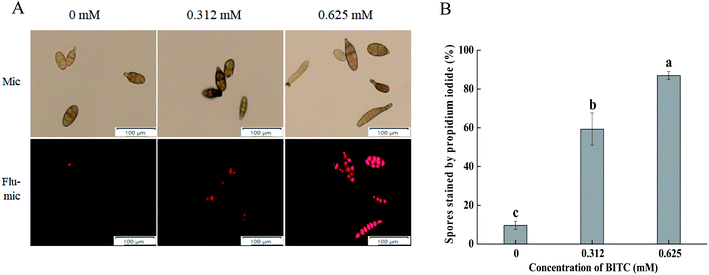 |
| Fig. 3 Effects of BITC treatment on the plasma membrane integrity of A. alternata. Spores incubated with different concentrations of BITC (0, 0.312 and 0.625 mM) were stained with propidium iodide (PI) and imaged under a fluorescence microscope. Spores with damaged plasma membranes showed fluorescence (A), and the percentage of spores with reduced plasma membrane integrity were analyzed (B). Three fields of view were randomly chosen for each treatment. Experiments were repeated on three occasions. Vertical bars represent standard errors. Columns marked by different lowercase letters significantly differed according to Duncan's multiple range tests (p < 0.05). | |
3.4 Cellular leakage of A. alternata following BITC treatment
As shown in Fig. 4A, cell membrane conductivity values of non-treated A. alternata slowly increased slowly within 0–120 min of incubation, whilst the external conductivity of BITC treated A. alternata cells showed a rapid upwards trend. The conductivity values increased with the increasing of BITC concentrations. The conductivity of 0.625 mM BITC treated A. alternata increased and peaked at 60 min (Fig. 4A), which was significantly higher than observed in control samples (37.4 ± 0.063). In addition, significant differences in nucleic acid leakage were detected comparing to the control (Fig. 4B). The nucleic acid content characterized by OD260 in the filtrates of A. alternata treated with BITC significantly increased during the incubation period. After 60 min, the OD260 values in 0.625 mM BITC-treated A. alternata filtrates were 7.2-fold higher than that in the controls (Fig. 4 B).
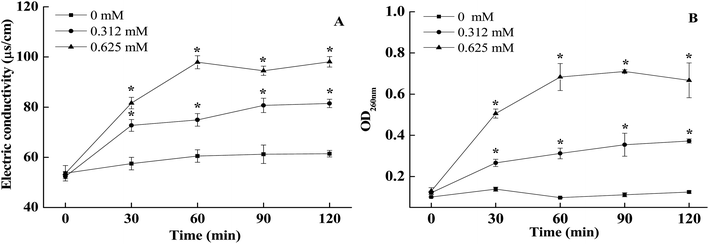 |
| Fig. 4 Effects of BITC on electrical conductivity (A) and nucleic acid leakage (B) of A. alternata. Vertical bars represent standard errors. Asterisk (*) are significantly different according to the Duncan's multiple range test (p < 0.05). | |
3.5 BITC treatment inhibited mycotoxin production in A. alternata
The retention time was determined by the standard curve and four mycotoxins were identified: AME, 2.845 min; AOH, 2.364 min; ALT, 2.333 min; TEN, 2.666 min (Table 1). The mass were 271.0 and 257.0 m/z under negative ionization mode for AME and AOH (Fig. S2A and C†). The quasi-molecular ions of ALT and TEN (m/z 293.1, [M + H]+; m/z 415.2, [M + H]+) suggest a molecular mass of 292 and 414 respectively (Fig. S2E and F†). Samples fumigated with 0.312 mM BITC showed significantly lower levels of mycotoxins (AME, AOH, ALT and TEN) in the A. alternata hyphae, and inhibitory effects varied with each type of mycotoxins. The inhibitory rates of BITC treatment on AME and AOH were 89.36% and 84.57% (p < 0.05), respectively (Fig. 5A and B). The TEN content decreased to 32.60 ± 0.46 μg g−1 compared to the control (141.70 ± 1.73 ng L−1) (Fig. 5D). The inhibitory rates of ALT were greater than 90% (Fig. 5C).
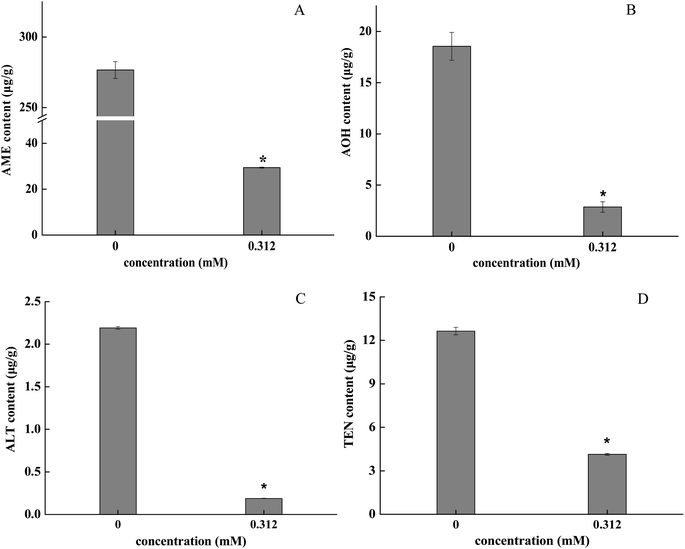 |
| Fig. 5 Effects of 0.312 mM BITC treatment on AME, AOH, ALT and TEN mycotoxins content in A. alternata. Vertical bars represent standard errors. Asterisk (*) indicates significant differences amongst sample groups (P < 0.05). | |
4. Discussion
ITCs is natural compounds with antimicrobial properties.17 Previous studies have shown that 0.1 mg L−1 ITCs reduced the incidence of Botrytis cinerea in strawberries by over 45% without detrimental effects on post-harvest quality.38 Our results showed that the incidence of A. alternata infection in pears fumigated with BITC at 1.25 mM for 12 h was significantly lower after 7 d of storage, in which the fruit showed the smallest infection areas, consistent with the findings of L. Ugolini et al.38 However, BITC concentrations of 1.25 mM led to a complete inhibition of mycelial growth in A. alternata. Thus inhibition of BITC on the growth was proved to be lower in vivo compared with the in vitro trial, these differences may be due to the diverse characteristics of stability through time and volatility in the compounds studied, as well as the diffusive effect of the active substance inside the wound.35 Visible mycelial began to grow after 2 days of treatment with 0.625 mM BITC. However, concentrations of 0.75 mg mL−1 BITC completely inhibited Fusarium culmorum growth in vitro.30 Those differences may be due to variable compound purity or application methods. M. Mari et al. demonstrated the inhibition of conidial germination and mycelial growth of several postharvest fruit pathogens by BITC and other natural ITCs.39,43 Our results were consistent with these studies, as BITC efficiently inhibited spore germination and colony expansion of A. alternata on PDA medium. Spore germination was incompletely inhibited at concentrations of 0.039–1.25 mM for 8 h, with spore germination rates decreasing from 86.67% to 3%. S. G. Harvey reported that the spore germination of Sclerotium rolfsii inhibition of 100% was not recorded even at the highest concentrations of 2-propenyl-isothiocyanate (528.8 mM), indicating that the spores were more resistant than mycelia.44 Our results suggested that BITC efficiently inhibits spore germination rates and colony expansion of A. alternata on PDA medium, and alleviates disease severity in pear fruits.
To further elucidate the mechanisms underlying the antifungal activity of BITC against A. alternata, a loss of membrane integrity and the leakage of cellular constituents of BITC-treated A. alternata were determined. BITC is lipophilic and may destroy membrane integrity.29 PI is a DNA-stain that shows red fluorescence when inserted into double-stranded DNA in cells that have lost membrane integrity.41,45 A higher number of PI stained A. alternata spores were observed in response to BITC fumigation, with the membrane integrity of A. alternata almost completely destroyed at BITC concentration of 0.625 mM. These were similar to the values previously reported by F. Yan et al.46
Lipophilic compound accumulates in the phospholipid bilayer and alters the permeability of the plasma membrane for K+ and H+, resulting in the disruption of ionic homeostasis.47,48 A loss of membrane integrity results in the leakage of small molecules ions and electrolytes from the cells.49,50 Membrane permeability parameters which are commonly used to indicate gross and irreversible damage to the cytoplasmic and plasma membranes included the loss of 260 nm absorbing materials (K+ and Na+ leakage).51,52 The release of electrolytes (Fig. 4A) and nucleic acids (Fig. 4B) in the fungal suspensions visibly increased with increasing BITC concentrations. The conductivity value of A. alternata suspensions treated with 0.312 and 0.625 mM of BITC increased over the initial 60 min, whilst the control group remained relatively stable within 0–120 min. The maximum release of cell constituents was observed in A. alternata suspensions treated with 0.625 mM BITC for 60 min, showing absorbances of 0.683. These findings suggest that the cytoplasmic membranes of A. alternata incurred irreversible damage after BITC fumigation. The results observed were in agreement with previous studies that suggested that the antibacterial activity of ITCs acts through the disturbance of membrane integrity.53,54
I. Clemente et al. observed the effects of BITC on enzymes on the P450 family (Ochratoxins A-synthase).30,55,56 BITC can reduce the biosynthesis of secondary metabolites (Ochratoxins A and B) by affecting the relevant metabolic pathways of Aspergillus ochraceus.57 A. alternata toxins are secondary metabolites produced by the A. alternata genera.58 Some early reports have shown that Alternaria species do not produce the most known and frequent Alternaria toxins (e.g. AOH, AME, TEN),11,59 thus we conduct relevant exploratory experiments. The data presented in this study showed that high levels AME, AOH, ALT and TEN were detected in non-BITC treated A. alternata, similar to the report by T. T. T. Nguyen et al.60 AME and AOH produced a high intensity (271.0 and 257.0 m/z) in negative mode (ESI−), and these results were consistent with the previous studies of A. A. Hildebrand et al. (2015).61 And significant reduction in mycotoxins contents was observed in BITC treated A. alternata (Fig. 5A and B), especially the inhibition rate of AME and ALT in response to BITC fumigation were 89.36% and 91.41%, respectively (p < 0.05). These results showed that BITC hold value foe reducing mycotoxin contamination in A. alternata. Combination with the effect of BITC on the integrity and permeability of A. alternata plasma membrane, mycotoxin of A. alternata may leak out to extracellular medium and interact with BITC after the damage of cell membrane, leading to degradation of mycotoxins. However, its specific mechanism of action governing these effects needs further clarification.
5. Conclusion
BITC could inhibit in vitro spore germination and mycelial growth in A. alternata and reduce the occurrence of black spots in pears. BITC treatment destroyed the morphology of the spores and mycelia of the pathogens increased the leakage of intercellular electrolytes and nucleic acids of A. alternata leading to lethal effects. The mechanisms underlying the antifungal toxic activity of BITC were through membrane disruption and cell growth hindrance. BITC also significantly inhibited the production of Alternaria toxins (AME, AOH, ALT and TEN). BITC may thus offer an economical and environmentally-friendly strategy to control postharvest disease in fruit and vegetables.
Conflicts of interest
There is no conflict of interest.
Acknowledgements
This study was financed by the National Key R&D Plans of China (2018YFD0401302) and the National Natural Science Foundation of China (31860456, 31460534).
References
- N. Estiarte, A. Crespo-Sempere, S. Marín, V. Sanchis and A. J. Ramos, Exploring polyamine metabolism of Alternaria alternata to target new substances to control the fungal infection, Food Microbiol., 2017, 65, 193–204 CrossRef CAS PubMed.
- C. Y. Diao, Y. Bi and Y. C. Li, The inhibition of chitosan on Alternaria alternata in vitro, Chinese Agricultural Science Bulletin, 2010, 26, 91–94 Search PubMed.
- S. M. Sanzani, M. Reverberi and R. Geisen, Mycotoxins in harvested fruits and vegetables: insights in producing fungi, biological role, conductive conditions, and tools to manage postharvest contamination, Postharvest Biol. Technol., 2016, 122, 95–105 CrossRef CAS.
- C. Pane, F. Fratianni, M. Parisi, F. Nazzaro and M. Zaccardelli, Control of Alternaria post-harvest infections on cherry tomato fruits by wild pepper phenolic-rich extracts, Crop Prot., 2016, 84, 81–87 CrossRef CAS.
- M. Greco, A. Patriarca, L. Terminiello, V. F. Pinto and G. Pose, Toxigenic Alternaria species from Argentinean blueberries, Int. J. Food Microbiol., 2012, 154, 187–191 CrossRef CAS PubMed.
- I. Kustrzeba-Wójcicka, E. Siwak, G. Terlecki, A. Wolańczyk-Mędrala and W. Mędrala, Alternaria alternata and its allergens: a comprehensive review, Clin. Rev. Allergy Immunol., 2014, 47, 354–365 CrossRef PubMed.
- Y. C. Li and Y. Bi, Occurrence and infection process of apple pear black spot disease, J. Plant Prot., 2006, 33, 131–135 Search PubMed.
- F. Kassel and C. Lillie, Coordinate control of oxidative stress tolerance, vegetative growth, and fungal pathogenicity via the AP1 pathway in the rough lemon pathotype of Alternaria alternata, Physiol. Mol. Plant Pathol., 2010, 74, 100–110 Search PubMed.
- P. López, D. Venema, H. Mol, M. Spanjer, J. De Stoppelaar and F. Pfeiffer, Alternaria toxins and conjugates in selected foods in the Netherlands, Food Control, 2016, 69, 153–159 CrossRef.
- V. Ostry, Alternaria mycotoxins: an overview of chemical characterization, producers, toxicity, analysis and occurrence in foodstuffs, World Mycotoxin J., 2008, 1, 175–188 CrossRef CAS.
- A. Patriarca, M. P. Azcarate, L. Terminiello and V. F. Pinto, Mycotoxin production by Alternaria strains isolated from Argentinean wheat, Int. J. Food Microbiol., 2007, 119, 219–222 CrossRef CAS PubMed.
- M. Wang, N. Jiang, Y. Wang, D. Jiang and X. Feng, Characterization of phenolic compounds from early and late ripening sweet cherries and their antioxidant and antifungal activities, J. Agric. Food Chem., 2017, 65, 5413–5420 CrossRef CAS PubMed.
- E. M. Soylu and F. Kose, Antifungal activities of essential oils against citrus black rot disease agent Alternaria alternata, J. Essent. Oil-Bear. Plants, 2015, 18, 894–903 CrossRef CAS.
- L. Xu, N. Tao and W. Yang, Cinnamaldehyde damaged the cell membrane of Alternaria alternata and induced the degradation of mycotoxins in vivo, Ind. Crops Prod., 2018, 112, 427–433 CrossRef CAS.
- J. D. S. Gabriel, M. J. Tiera and V. A. D. O. Tiera, Synthesis, characterization, and antifungal activities of amphiphilic derivatives of diethylaminoethyl chitosan against Aspergillus flavus, J. Agric. Food Chem., 2015, 63, 5725–5731 CrossRef CAS PubMed.
- S. K. Srivastava and S. V. Singh, Cell cycle arrest apoptosis induction and inhibition of nuclear factor kappa B activation in anti-proliferative activity of benzyl isothiocyanate against human pancreatic cancer cells, Carcinogenesis, 2004, 25, 1701–1709 CrossRef CAS PubMed.
- E. A. S. Rosa and P. M. F. Rodrigues, Towards a more sustainable agriculture system: the effect of glucosinolates on the control of soil-borne diseases, J. Hortic. Sci. Biotechnol., 1999, 74, 667–674 CrossRef CAS.
- D. D. Archbold, T. R. Hamilton-Kemp and M. M. Barth, Identifying natural volatile compounds that control gray mold (Botrytis cinerea) during postharvest storage of strawberry, blackberry, and grape, J. Agric. Food Chem., 1997, 45, 4032–4037 CrossRef CAS.
- J. A. Kirkegaard and M. Sarwar, Biofumigation potential of brassicas–I. Variation in glucosinolate profiles of diverse field-grown brassicas, Plant Soil, 1998, 201, 71–89 CrossRef CAS.
- U. Smolinska, M. J. Morra, G. R. Knudsen and R. L. James, Isothiocyanates produced by Brassicaceae species as inhibitors of Fusarium oxysporum, Plant Dis., 2003, 87, 407–412 CrossRef CAS PubMed.
- O. D. Dhingra, M. L. N. Costa and G. J. Silva, Potential of allyl isothiocyanate to control Rhizoctonia solani seedling damping off and seedling blight in transplant production, J. Phytopathol., 2004, 152, 352–357 CrossRef CAS.
- M. V. Galan, A. A. Kishan and A. L. Silverman, Oral broccoli sprouts for the treatment of Helicobacter pylori infection: a preliminary report, Dig. Dis. Sci., 2004, 49, 1088–1090 CrossRef PubMed.
- R. Kermanshai, B. E. McCarry, J. Rosenfeld, P. S. Summers, E. A. Weretilnyk and G. J. Sorger, Benzyl isothiocyanate is the chief or sole anthelmintic in papaya seed extracts, Phytochemistry, 2001, 57, 427–435 CrossRef CAS PubMed.
- I. A. Zasada and H. Ferris, Sensitivity of Meloidogyne javanica and Tylenchulus semipenetrans to isothiocyanates in laboratory assays, Phytopathology, 2003, 93, 747–750 CrossRef CAS PubMed.
- R. F. Mithen, Glucosinolates and their degradation products, Adv. Bot. Res., 2001, 35, 213–262 CAS.
- J. W. Fahey, A. T. Zalcmann and P. Talalay, The chemical diversity and distribution of glucosinolates and isothiocyanates among plants, Phytochemistry, 2001, 56, 5–51 CrossRef CAS PubMed.
- R. Troncoso, C. Espinoza, A. Sánchez–Estrada, M. E. Tiznado and H. S. Garcia, Analysis of the isothiocyanates present in cabbage leaves extract and their potential application to control Alternaria rot in bell peppers, Food Res. Int., 2005, 38, 701–708 CrossRef CAS.
- L. M. Manici, L. Lazzeri and S. Palmieri, In vitro fungitoxic activity of some glucosinolates and their enzyme-derived products toward plant pathogenic fungi, J. Agric. Food Chem., 1997, 45, 2768–2773 CrossRef CAS.
- R. Troncoso-Rojas, A. Sánchez-Estrada, C. Ruelas, H. S. García and M. E. Tiznado-Hernández, Effect of benzyl isothiocyanate on tomato fruit infection development by Alternaria alternata, J. Sci. Food Agric., 2005, 85, 1427–1434 CrossRef CAS.
- M. E. Tiznado-Hernández and R. Troncoso-Rojas, Control of fungal diseases with isothiocyanates, Stewart Postharvest Review, 2006, 2(1), 1–14 Search PubMed.
- Y. Wang, Q. Q. Zhou and R. L. Wan, Inhibition of two gram-positive pathogenic bacteria by isothiocyanate spices, Food Sci., 2017, 21, 22–27 Search PubMed.
- J. Sikkema, J. A. de Bont and B. Poolman, Mechanisms of membrane toxicity of hydrocarbons, Microbiol. Rev., 1995, 59, 201–222 CrossRef CAS PubMed.
- C. Hontanaya, G. Meca, F. B. Luciano, J. Manes and G. Font, Inhibition of the Aflatoxins B1, B2, G1, and G2, production by Aspergillus parasiticus in nuts using yellow and oriental mustard flours, Food Control, 2015, 47, 154–160 CrossRef CAS.
- I. Clemente, M. Aznar and C. Nerín, Effect of an active label based on benzyl isothiocyanate on the morphology and ochratoxins production of Aspergillus ochraceus, Food Res. Int., 2017, 101, 61–72 CrossRef CAS PubMed.
- M. Mari, R. Iori, O. Leoni and A. Marchi, Bioassay of glucosinolate-derived isothiocyanates against post-harvest pest pathogens, Plant Pathol., 1996, 45, 753–760 CrossRef CAS.
- N. L. Tatsadjieu, P. M. J. Dongmo, M. B. Ngassoum, F. X. Etoa and C. M. F. Mbofung, Investigations on the essential oil of Lippia rugosa from Cameroon for its potential use as antifungal agent against Aspergillus flavus Link ex. Fries, Food Control, 2009, 20(2), 161–166 CrossRef CAS.
- H. Li, Z. Q. Zhang, C. He, G. Z. Qin and S. P. Tian, Comparative proteomics reveals the potential targets of BcNoxR, a putative regulatory subunit of NADPH oxidase of Botrytis cinerea, Mol. Plant-Microbe Interact., 2016, 29, 990–1003 CrossRef CAS PubMed.
- L. Ugolini, C. Martini, L. Lazzeri, L. D'Avino and M. Mari, Control of postharvest grey mould (Botrytis cinerea Per.: Fr.) on strawberries by glucosinolate-derived allyl-isothiocyanate treatments, Postharvest Biol. Technol., 2014, 90, 34–39 CrossRef CAS.
- M. Mari, O. Leoni, R. Bernardi, F. Neri and S. Palmieri, Control of brown rot on stonefruit by synthetic and glucosinolate-derived isothiocyanates, Postharvest Biol. Technol., 2007, 47, 61–67 CrossRef.
- F. Wang, F. Y. Wei, C. X. Song, Y. X. Huang and Y. X. Li, Dodartia orientalis L. essential oil exerts antibacterial activity by mechanisms of disrupting cell structure and resisting biofilm, Ind. Crops Prod., 2017, 109, 358–366 CrossRef CAS.
- J. Tian, Y. Wang, H. Zeng, Z. Li, P. Zhang, A. Tessema and X. Peng, Efficacy and possible mechanisms of perillaldehyde in control of Aspergillus niger causing grape decay, Int. J. Food Microbiol., 2015, 202, 27–34 CrossRef CAS PubMed.
- D. R. N. A. Cezar, M. Maraschin and R. M. Di Piero, Antifungal activity of salicylic acid against Penicillium expansum and its possible mechanisms of action, Int. J. Food Microbiol., 2015, 215, 64–70 CrossRef PubMed.
- H. Wu, X. Zhang, G. Zhang, S. Zeng and K. Kin, Antifungal vapour-phase activity of a combination of allyl isothiocyanate and ethyl isothiocyanate against Botrytis cinerea and Penicillium expansum infection on apples, J. Phytopathol., 2011, 159, 4050–4455 Search PubMed.
- S. G. Harvey, H. N. Hannahan and C. E. Sams, Indian mustard and allyl-isothiocyanate inhibit Sclerotium rolfsii, J. Am. Soc. Hortic. Sci., 2002, 127(1), 27–31 CAS.
- D. J. Novo, N. G. Perlmutter, R. H. Hunt and H. M. Shapiro, Multiparameter flow cytometric analysis of antibiotic effects on membrane potential, membrane permeability, and bacterial counts of staphylococcus aureus and micrococcus luteus, Antimicrob. Agents Chemother., 2000, 44(4), 827–834 CrossRef CAS PubMed.
- F. Yan, Q. Dang, C. Liu, J. Yan, T. Wang and B. Fan, 3,6-O-[N-(2-Aminoethyl)-acetamide-yl]-chitosan exerts antibacterial activity by a membrane damage mechanism, Carbohydr. Polym., 2016, 149, 102–111 CrossRef CAS PubMed.
- L. da Cruz Cabral, V. F. Pinto and A. Patriarca, Application of plant derived compounds to control fungal spoilage and mycotoxin production in foods, Int. J. Food Microbiol., 2013, 166, 1–14 CrossRef CAS PubMed.
- D. G. Yun and D. G. Lee, Silymarin exerts antifungal effects via membrane-targeted mode of action by increasing permeability and inducing oxidative stress, Biochim. Biophys. Acta, Biomembr., 2017, 1859, 467–474 CrossRef CAS PubMed.
- V. K. Bajpai, A. Sharma and K. H. Baek, Antibacterial mode of action of Cudrania tricuspidata fruit essential oil, affecting membrane permeability and surface characteristics of food-borne pathogens, Food Control, 2013, 32, 582–590 CrossRef CAS.
- J. Liu, Y. Y. Zong, G. Z. Qin, B. Q. Li and S. P. Tian, Plasma membrane damage contributes to antifungal activity of silicon against Penicillium digitatum, Curr. Microbiol., 2010, 61, 274–279 CrossRef CAS PubMed.
- S. Paul, R. C. Dubey, D. K. Maheswari and S. C. Kang, Trachyspermum ammi (L.) fruit essential oil influencing on membrane permeability and surface characteristics in inhibiting food-borne pathogens, Food Control, 2011, 22, 725–731 CrossRef CAS.
- M. Turgis, J. Han, S. Caillet and M. Lacroix, Antimicrobial activity of mustard essential oil against Escherichia coli O157: H7 and Salmonella typhi, Food Control, 2009, 20, 1073–1079 CrossRef CAS.
- C. M. Lin, J. F. Preston and C. I. Wei, Antibacterial mechanism of allyl isothiocyanate, J. Food Prot., 2000, 63, 727–734 CrossRef CAS PubMed.
- A. C. Chan, D. Ager and I. P. Thompson, Resolving the mechanism of bacterial inhibition by plant secondary metabolites employing a combination of whole-cell biosensors, J. Microbiol. Methods, 2013, 93, 209–217 CrossRef CAS PubMed.
- I. Clemente, M. Aznar and C. Nerín, Raman imaging spectroscopy as a tool to investigate
the cell damage on Aspergillus ochraceus caused by an antimicrobial packaging containing Benzyl isothiocyanate, Anal. Chem., 2016, 88, 4772–4779 CrossRef CAS PubMed.
- T. C. Goosen, D. E. Mills and P. F. Hollenberg, Effects of benzyl isothiocyanate on rat and human cytochromes P450: Identification of metabolites formed by P4502B1, J. Pharmacol. Exp. Ther., 2001, 296, 198–206 CAS.
- A. Gallo, K. S. Bruno, M. Solfrizzo, G. Perrone, G. Mule, A. Visconti and S. E. Baker, New insight into the Ochratoxin A biosynthetic pathway through deletion of a nonribosomal peptide synthetase gene in aspergillus carbonarius, Appl. Environ. Microbiol., 2012, 78, 8208–8218 CrossRef CAS PubMed.
- M. Wang, N. Jiang, H. Xian, D. Wei and X. Feng, A single-step solid phase extraction for the simultaneous determination of 8 mycotoxins in fruits by ultra-high performance liquid chromatography tandem mass spectrometry, J. Chromatogr. A, 2015, 1429, 22–29 CrossRef PubMed.
- B. Andersen, E. Krøger and R. G. Roberts, Chemical and morphological segregation of Alternaria arborescens, A. infectoria and A. tenuissima species-groups, Mycol. Res., 2002, 106, 170–182 CrossRef CAS.
- T. T. T. Nguyen, J. Kim, S. J. Jeon, C. W. Lee, N. Magan and H. B. Lee, Mycotoxin production of, Alternaria, strains isolated from Korean barley grains determined by LC-MS/MS, Int. J. Food Microbiol., 2018, 268, 44–52 CrossRef CAS PubMed.
- A. A. Hildebrand, B. N. Kohn, E. Pfeiffer, D. Wefers and M. Bunzel, Conjugation of the mycotoxins alternariol and alternariol monomethyl ether in tobacco suspension cells, J. Agric. Food Chem., 2015, 63, 4728–4736 CrossRef CAS PubMed.
Footnote |
† Electronic supplementary information (ESI) available. See DOI: 10.1039/c9ra09225k |
|
This journal is © The Royal Society of Chemistry 2020 |