DOI:
10.1039/C9RA07891F
(Paper)
RSC Adv., 2020,
10, 5234-5240
Facile and rapid synthesis of a novel spindle-like heterojunction BiVO4 showing enhanced visible-light-driven photoactivity
Received
29th September 2019
, Accepted 27th December 2019
First published on 31st January 2020
Abstract
A spindle-like monoclinic–tetragonal heterojunction BiVO4 was successfully synthesized by a pressure-controllable microwave method. The as-prepared BiVO4 samples were characterized by X-ray diffraction (XRD), scanning electron microscopy (SEM), transmission electron microscopy (TEM), X-ray photoelectron spectroscopy (XPS), UV-vis diffuse reflectance spectroscopy (DRS), photoluminescence (PL) spectroscopy, transient photocurrent responses and electrochemical impedance spectroscopy (EIS). The visible-light-driven photocatalytic activity of the BiVO4 samples was evaluated for the degradation of Rhodamine B (RhB) and tetracycline (TC). The synthesis process needs microwave irradiation for only 10 min without the addition of any auxiliary reagent, pH adjustment, and calcination. The as-prepared spindle-like monoclinic–tetragonal heterojunction BiVO4 exhibits excellent photocatalytic activity for the degradation of both RhB and TC. The photocatalytic degradation rates of RhB and TC over spindle-like BiVO4 are 1.77 and 1.64 times higher, respectively, than that measured over monoclinic BiVO4. The enhanced photocatalytic activity is mainly attributed to the fact that the existence of a heterojunction effectively promotes the separation of photo-generated carriers and extends the visible-light absorption of BiVO4.
Introduction
Over the past decades, photocatalysts have attracted considerable attention in the field of energy and environment.1–5 Due to its high stability, low cost, non-toxicity and strong response to ultraviolet light, semiconductor oxide TiO2 has been considered as an effective photocatalyst. However, the broad applications of TiO2 are restricted because it can only utilize the ultraviolet (3–4%) fraction of the solar energy.6,7 As a result, many researchers have been concentrating on investigating photocatalysts with visible-light-driven activity. BiVO4 with a narrow band gap exhibits strong absorption of visible light and has been recognized as a promising visible-light-driven photocatalyst for the degradation of pollutants and water splitting.8,9 As is known, BiVO4 has three crystal types: tetragonal zircon type, tetragonal scheelite type, and monoclinic scheelite type. Of these, tetragonal zircon and monoclinic scheelite types are considered to possess photocatalytic properties. Tetragonal zircon type bismuth vanadate (t-BiVO4) shows lower photocatalytic activity, while monoclinic bismuth vanadate (m-BiVO4) exhibits higher activity. Nevertheless, poor adsorption performance and rapid recombination of photo-induced electrons and holes inhibit the efficient activity of m-BiVO4. Therefore, various methods have been developed to improve the photocatalytic properties of BiVO4. Morphological control has been proven to be an effective approach to enhance photocatalytic activity. Up to now, researchers have prepared many kinds of BiVO4 with specific morphologies, such as tubular,10 one-dimensional band,11 rod-like,12 two-dimensional sheet-shaped,13 three-dimensional spherical,14 multi-layer flower-like,15 sandwich-like,16 pumpkin-like,17 and elliptical.18 In addition, the construction of a monoclinic–tetragonal heterojunction is an ideal means to increase the photocatalytic activity.19–21 At present, BiVO4 materials with specific morphologies or monoclinic–tetragonal heterojunctions have been generally synthesized by the hydrothermal method that requires a very long time of more than 10 hours. Moreover, complicated processes, such as the use of solvents or auxiliary reagents, pH adjustment, calcination, and annealing treatment, are involved in hydrothermal preparations to obtain specific morphologies.
Microwave-assisted synthesis has been widely applied in synthesizing nanoscale inorganic materials due to homogeneous nucleation and rapid crystallization. The microwave-assisted preparation of the BiVO4 photocatalyst has been reported by a few researchers.22,23 Nevertheless, the microwave-assisted synthesis at normal pressure requires several hours. Recently, it has been reported that BiVO4 can be successfully synthesized by a high-pressure microwave method.24,25 The microwave-assisted synthesis of BiVO4 under a high pressure can save reaction time and control morphology.
Herein, we report pressure-controllable microwave synthesis of a spindle-like monoclinic–tetragonal heterojunction BiVO4. This synthesis method is facile and fast, only taking about 10 minutes of reaction time, without the addition of organic solvents and surfactants, adjustment of pH and calcination.
Experimental section
Preparation of BiVO4
Here, 1.456 g of Bi(NO3)3·5H2O was dissolved in 30 mL of deionized water and sonicated for 10 min to obtain solution A. Then, 0.36 g of NaVO3 was dissolved in 20 mL of deionized water and sonicated for 10 min to obtain solution B. The solution B was added dropwise to the solution A under stirring. The obtained orange-yellow suspension was stirred at room temperature for 30 min and then transferred to a polytetrafluoroethylene digestion tank that was placed in a pressure-controllable microwave digestion apparatus for irradiation for 10 min. During the microwave irradiation, the microwave power, reactor pressure and reaction time were changed according to a pre-designed procedure (stage 1: microwave power 600 W, reactor pressure 5 kg cm−1,2 reaction time 1.5 min; stage 2: microwave power 700 W, reactor pressure 10 kg cm−2, reaction time 2 min; stage 3: microwave power 900 W, reactor pressure 15 kg cm−2, reaction time 3.5 min; stage 4: microwave power 900 W, the reactor pressure 20 kg cm−2, reaction time 3.5 min). The yellow products obtained after stage 2, stage 3 and stage 4 were washed with absolute ethanol and deionized water, dried at 80 °C, and marked as MB-2, MB-3, and MB-4, respectively.
For comparison, a single-phase m-BiVO4 sample was prepared by the hydrothermal method and marked as HB. The preparation method of HB was as follows: 9.7 g of Bi(NO3)3·5H2O was dissolved in 20 mL of 4 mol L−1 HNO3 solution to form a transparent solution A. Then, 2.34 g of NH4VO3 was dissolved in 20 mL of 2 mol L−1 NaOH solution to obtain solution B. The solution B was added dropwise to the solution A under stirring and was further stirred for 2 hours at room temperature. The pH of the mixture was adjusted to 7 with NaOH to give an orange-yellow solution. The orange-yellow solution was added to a 100 mL Teflon reactor and heated at 180 °C for 24 h. The product was naturally cooled to room temperature, washed with water and ethanol several times, and dried at 80 °C to obtain a BiVO4 sample HB.
Characterization of BiVO4
The XRD patterns of the samples were recorded on a D8 Advance X-ray diffractometer (Bruker, Germany) using Cu-Kα radiation (λ = 1.5406 Å). The SEM and TEM images of the samples were observed on an S-4800 field emission scanning electron microscope (Hitachi, Japan) and a JEM-2100 transmission electron microscope (Japan Electronics, Japan), respectively. UV-vis diffuse reflectance spectra were obtained on a UV-2550 UV-vis spectrophotometer (Shimadzu, Japan) using BaSO4 as a reflectance standard. The XPS spectra were collected on an ESCALAB-250i X-ray photoelectron spectroscope (Thermo Fisher Scientific, USA). PL spectra were recorded on an F4500 fluorescence spectrometer. Transient photocurrent responses and EIS of the sample were measured on a CHI 660D electrochemical workstation (Chenhua Instrument, China) with an FTO electrode deposited with a catalyst as the working electrode using Na2SO4 aqueous solution of 0.1 mol L−1 as an electrolyte under visible-light illumination.
Photocatalytic activity test
The photocatalytic activity was evaluated for the degradation of Rhodamine B (RhB) and tetracycline (TC) under visible-light irradiation. Typically, 100 mg of BiVO4 was added to 100 mL of RhB or TC solution with a concentration of 5 mg L−1 and stirred for 30 min in the dark for adsorption–desorption equilibrium. Then, the solution was irradiated by a 300 W xenon lamp with a 420 nm cut-off filter. Three mL of the solution was sampled at 15 min intervals. The concentrations of RhB and TC in the solution were analysed on a UV-Vis spectrophotometer at the wavelengths of 554 and 357 nm, respectively.
Results and discussion
XRD analysis
Fig. 1 shows the XRD patterns of the samples. The diffraction peaks of HB are completely consistent with those expected for m-BiVO4 (standard card number JCPDS No. 14-0688), indicating that BiVO4 prepared by the hydrothermal method is a pure phase of m-BiVO4. In the XRD patterns of MB-2, MB-3 and MB-4, not only the diffraction peaks at 18.5°, 28.8°, and 30.5°, but also the peaks at 24.5°, 32.6°, and 39.5° appeared. The peaks at 18.5°, 28.8°, and 30.5° belong to the (110), (121), and (040) crystal planes of m-BiVO4, respectively. In contrast, the diffraction peaks at 24.5°, 32.6°, and 39.5° correspond to the (200), (112), and (301) crystal planes of t-BiVO4 (standard card number JCPDS No. 14-0133). It is suggested that MB-2, MB-3 and MB-4 prepared at different reaction stages are the composites of m-BiVO4 and t-BiVO4. Moreover, compared with the diffraction peak intensities of HB, MB-2, and MB-3, the diffraction peak intensity of MB-4 at 18.5°, 28.8°, and 30.5° weakened with the broadening of the peaks. According to formula (1),26 the proportions of tetragonal BiVO4 in the MB-2, MB-3, and MB-4 composites were estimated to be 6.3%, 3.5%, and 23%, respectively. |
ηtetra = Itetra(200)/(Itetra(200) + Imono(121))
| (1) |
Here, ηtetra represents the proportion of the tetragonal phase. Itetra(200) and Imono(121) are the relative intensities of the diffraction peaks of the tetragonal phase (200) and monoclinic phase (121), respectively.
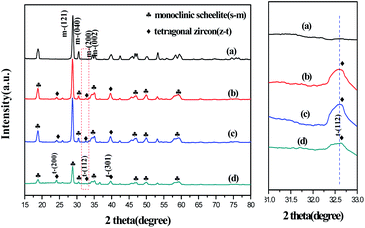 |
| Fig. 1 XRD patterns of HB (a), MB-2 (b), MB-3 (c) and MB-4 (d). | |
Thus, the XRD results indicated that BiVO4 was obtained only within 3.5 min under microwave irradiation with the microwave reaction pressure increasing from 5 kg cm−2 to 10 kg cm−2. This is due to the acceleration of the precipitation and crystallization of BiVO4 under high-pressure microwave conditions. Furthermore, microwave pressure plays a significant role in the phase transition of BiVO4. By increasing the reaction pressure and time, the transformation from m-BiVO4 to t-BiVO4 was achieved. The probable reason is that m-BiVO4 was thermodynamically more stable under the condition of 5 kg cm−2 to 10 kg cm−2 pressure; thus, MB-2 obtained in stage 2 mainly consists of m-BiVO4. In stage 3 and 4, increased pressure resulted in partial dissolution–recrystallization of m-BiVO4 formed in stage 2 to form a composite of m-BiVO4 and t-BiVO4.
SEM and TEM
The morphology of the samples was investigated using SEM and TEM (Fig. 2). The SEM image in Fig. 2(a) indicates that m-BiVO4 prepared by the hydrothermal method is in the form of flakes with some agglomeration and size of about 1 μm.
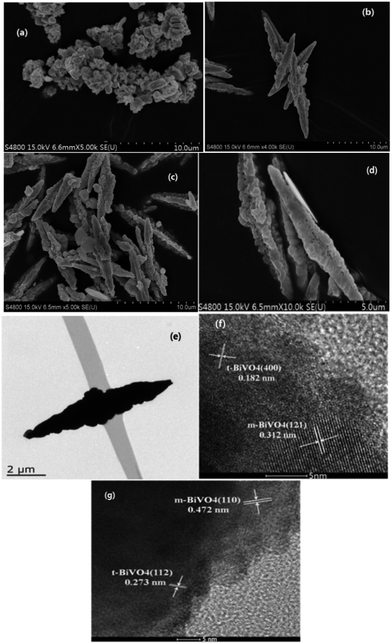 |
| Fig. 2 SEM images of HB (a) and MB-2 (b), MB-2 (c), MB-4 (d). TEM image of MB-4 (e) and HRTEM images of MB-4 (f and g). | |
Fig. 2(b–d) reveal that the BiVO4 samples prepared by the microwave method appear as spindle-like particles with a rough surface and length of about 10 μm, which are constructed by the assembly of many sheet-shaped particles of uniform diameter. It was indicated that the spindle-like morphology was formed at stage 2 with a microwave power of 700 W and a reactor pressure of 10 kg cm−2. Nevertheless, the BiVO4 sample MB-4 obtained in stage 4 had more regular and perfect spindle-like morphology. The TEM image of MB-4 (Fig. 2(e)) confirms the solid construction of spindle-like BiVO4. Fig. 2(f) and (g) show the high-resolution TEM images of MB-4. In Fig. 2(f), the crystal planes with the lattice fringe spacings of 0.312 nm and 0.182 nm belong to the (121) plane of m-BiVO4 and the (400) plane of t-BiVO4, respectively. The (121) plane of m-BiVO4 is evidently in close contact with the (400) plane of t-BiVO4. Fig. 2(e) shows that the (110) plane of m-BiVO4 is in close contact with the (112) plane of t-BiVO4. Therefore, it is concluded that the heterojunction is formed between m-BiVO4 and t-BiVO4.
X-ray photoelectron spectroscopy (XPS) analysis
X-ray photoelectron spectroscopy (XPS) was conducted to investigate the chemical state and surface composition of the sample. The XPS spectra of MB-4 based on C1s are presented in Fig. 3.
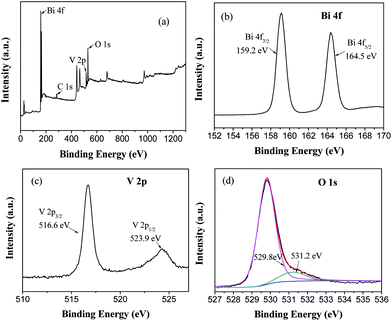 |
| Fig. 3 XPS survey spectra of MB-4 (a) and high-resolution XPS spectra of Bi 4f (b), V 2p (c), O 1s (d). | |
It is observed from the XPS survey spectrum (Fig. 3(a)) that MB-4 consists of Bi, V and O. In the high-resolution spectrum of Bi (Fig. 3(b)), the peaks at 159.2 eV and 164.5 eV are assigned to Bi 4f7/2 and Bi 4f5/2, respectively, which indicates that the bismuth element in the spindle-like BiVO4 exists as Bi3+.27 The peaks at 523.9 eV and 516.6 eV in Fig. 3(c) are characteristic spin–orbit splitting signals of V 2p1/2 and V 2p3/2, respectively, suggesting that all the vanadium species in the BiVO4 sample are present in the pentavalent state.28 The O1s spectra (Fig. 3(d)) can be differentiated into two peaks with the binding energies of 529.8 eV and 531.2 eV, which are attributed to the lattice oxygen of BiVO4 and adsorbed oxygen such as surface-adsorbed ˙OH and H2O, respectively. It is universally known that higher adsorbed oxygen means more oxygen vacancies, which will result in the enhancement in the photocatalytic activity.29
BET surface area
The N2 adsorption–desorption isotherm and the Barrett–Joyner–Halenda plot for the pore size distribution of MB-4 are shown in Fig. 4.
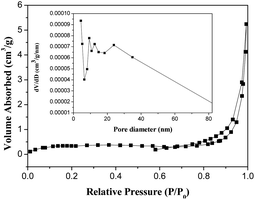 |
| Fig. 4 Nitrogen adsorption–desorption isotherm and pore size distribution plot of MB-4. | |
As seen in Fig. 4, the pores have a diameter of 10–80 nm; the average pore diameter is 32.204 nm. Based on the Brunauer–Emmett–Teller (BET) model, the surface area of MB-4 is estimated to be 1.596 m2 g−1. The small specific surface area is perhaps due to the fact that MB-4 is a large micron-sized particle, which is tightly assembled by a large number of nanosized sheet-shaped BiVO4.
UV-vis diffuse reflectance spectroscopy (DRS)
The optical absorption property of a semiconductor is considered as a crucial factor for its photocatalytic performance. UV-vis diffuse reflectance spectroscopy was used to detect the optical response characteristics of the sample. Fig. 5(a) shows the UV-vis diffuse reflectance spectra of HB and MB-4. As seen from Fig. 5(a), both the samples display strong absorption in the visible-light region, which is due to the migration of an electron from the Bi 6s and O 2p orbitals to the V 3d orbitals.30 The absorption edge of HB is around 520 nm, while the absorption edge of MB-4 shifts to 550 nm. It is concluded that the spindle-like BiVO4 heterojunction synthesized by the microwave method has superior visible-light response characteristics to those of m-BiVO4.
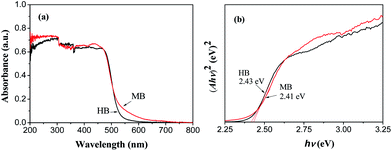 |
| Fig. 5 UV-vis diffuse reflectance spectra of HB and MB-4. | |
The band gap values of a semiconductor can be estimated by the Tauc formula αhν = A(hν − Eg)n/2, where α is the absorption coefficient, h is the Planck constant, and ν is the optical frequency; A is a constant and Eg is the band gap energy of the semiconductor.31 For BiVO4, a direct bandgap semiconductor, the value of n is 1.32 According to the Tauc formula, a plot of (αhν)2 versus (hν) is obtained and shown in Fig. 5(b). The Eg values of m-BiVO4 and spindle-like BiVO4, obtained from the intercept of the tangent of the curve of (αhν)2 versus (hν) on the X-axis, are 2.43 eV and 2.41 eV, respectively. The band gap of spindle-like BiVO4 is less than that of m-BiVO4.
The conduction and valence band potentials of a semiconductor can be determined by following eqn (2) and (3):21,24
Here,
EVB and
ECB are the conduction and valence band potentials, respectively.
Eg is the band-gap energy of the semiconductor,
Ee is the energy of the free electrons on the hydrogen scale (about 4.5 eV), and
X is the electronegativity of the semiconductor. The
X value for BiVO
4 is 6.04 eV. According to
eqn (2) and
(3), the conduction and valence band potentials of m-BiVO
4 are obtained as 0.32 eV and 2.75 eV, respectively.
Photocatalytic property
The photocatalytic property of the as-prepared BiVO4 was evaluated for the degradation of RhB and tetracycline (TC) under the irradiation of visible light. Fig. 6(a) shows that the degradation rate of RhB over MB-4 is high and up to 90.8% after 180 min of irradiation. However, the degradation rate of RhB over HB is 70.9%. Fig. 6(b) reveals that the degradation rate of TC over MB-4 is 78.9% within 180 min, while the degradation rate of TC over HB is 61.9%. For the degradation of both RhB and TC, the photocatalytic activity of the spindle-like heterojunction BiVO4 (MB-4) is significantly higher than that of single-phase m-BiVO4 (HB). Hence, the spindle-like heterojunction BiVO4 exhibits excellent photocatalytic activity for the degradation of RhB and TC.
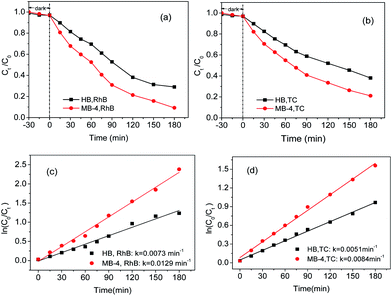 |
| Fig. 6 (a) Photodegradation of RhB, (b) photodegradation of TC under visible light, (c) kinetics of degradation of RhB and (d) kinetics of degradation of TC. | |
It is well-known that the photocatalytic degradation of organic pollutants follows apparent first-order kinetics expressed as eqn (4).33
Here,
C0 and
Ct represent the concentrations of RhB at the initial time and
t time, respectively;
k is the apparent reaction constant. As can be seen from
Fig. 6(c), the
k value of RhB over MB-4 is 0.0129 min
−1, which is 1.77 times higher than that of RhB over HB.
Fig. 6(d) indicates that the
k value of TC over MB-4 is 0.0084 min
−1, which is 1.64 times higher than that of TC over HB. Compared with the photocatalytic activity of HB, the enhanced photocatalytic activity of MB-4 is probably due to the surface-phase junctions formed between m-BiVO
4 and t-BiVO
4 and the red shift in the absorption edge of MB-4.
To evaluate the stability of spindle-like BiVO4, cyclic degradation experiments were carried out and the results are shown in Fig. 7. It can be found that the photocatalytic activity of the spindle-like BiVO4 does not decrease even after four cycling runs, confirming its excellent stability and reusability under visible light.
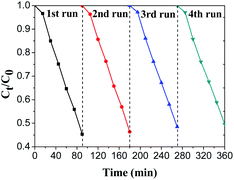 |
| Fig. 7 Recycling runs of the spindle-like BiVO4 for photocatalytic degradation of RhB. | |
Photoluminescence (PL) spectra
To probe a possible reason for the higher photocatalytic activity of MB-4, PL spectra were obtained to investigate the recombination probability of electron–hole pairs. Fig. 8 gives the PL spectra of HB and MB-4. As shown in Fig. 8, the PL spectra of MB-4 and HB are substantially similar, and a strong emission peak is observed at about 708 nm. However, compared with the observation for HB, the PL emission intensity of MB-4 weakened, suggesting that the recombination of electrons and holes in the spindle-like BiVO4 was effectively inhibited.
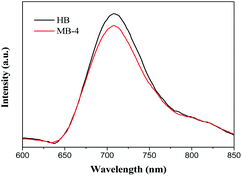 |
| Fig. 8 Photoluminescence spectra of HB and MB-4. | |
Transient photocurrent responses and EIS
In order to further investigate the separation and transfer of photo-induced charges, transient photocurrent responses and EIS studies were conducted. Fig. 9(a) gives the transient photocurrent response of MB-4 and HB. MB-4 exhibits remarkably higher photocurrent than that shown by HB, indicating more efficient separation of electron–hole pairs in spindle-like BiVO4.34 Fig. 9(b) shows the EIS results of MB-4 and HB. It is found that MB-4 has an obviously smaller radius than HB, further confirming that the charge transfer in spindle-like BiVO4 is significantly faster than that in pure m-BiVO4. It is because the interface of tetragonal–monoclinic heterojunction can promote the separation of photo-generated electron–hole pairs.35,36 Thus, the relatively high photocatalytic activity of MB-4 is mainly attributed to a smaller recombination rate of photo-induced carriers and its better optical absorption capacity.
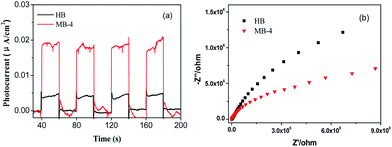 |
| Fig. 9 (a) Transient photocurrent response and (b) EIS of MB-4 and HB. | |
Detection of active species
To further explore the probable mechanism of charge carriers transferring in MB-4, the active species produced during the photocatalytic degradation of RhB over MB-4 were identified by a free radical trapping test. EDTA-2Na, isopropanol, and p-benzoquinone were selected as scavengers to be added into the RhB solution to remove the holes (h+), hydroxyl radicals (˙OH) and superoxide radicals (˙O2−), respectively. The photocatalytic activity of RhB in the presence of scavengers is given in Fig. 10. As can be seen, the addition of EDTA-2Na results in a significant decrease in the photocatalytic degradation of RhB. In the presence of p-benzoquinone, the photocatalytic activity of the MB-4 sample is slightly inhibited. However, the degradation of RhB has no obvious decrease when isopropanol is used as a scavenger. Therefore, it is indicated that the holes mainly account for the degradation of RhB.
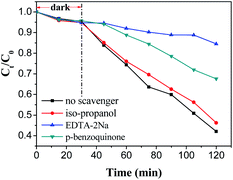 |
| Fig. 10 Photodegradation of RhB over MB-4 in the presence of different scavengers. | |
Probable mechanism
According to our experimental results, ECB and EVB of m-BiVO4 are 0.32 eV and 2.75 eV, respectively. In contrast, ECB and EVB of t-BiVO4 have been reported in a reference to be 0.24 eV and 2.84 eV, respectively.21 Based on the results about the conduction band and valence band potentials, transient photocurrent responses and active species, the possible charge migration during the photocatalytic degradation of RhB is speculated and shown in Fig. 11. Both m-BiVO4 and t-BiVO4 are excited by visible light to generate electrons and holes. The photo-generated electrons on the conduction band of t-BiVO4 migrate to the conduction band of m-BiVO4 because the conduction band of m-BiVO4 is below the conduction band of t-BiVO4. At the same time, the photo-induced holes on the valence band of t-BiVO4 can be easily transferred to the valence band of m-BiVO4. Consequently, the photo-generated electron–hole pairs are effectively separated. The holes with significantly positive potential on the surface can directly oxidize RhB.
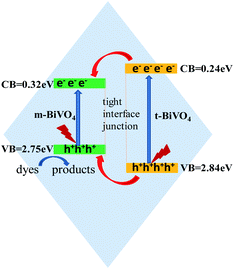 |
| Fig. 11 Illustration of probable charge transfer on the interface junction of m-BiVO4 and t-BiVO4. | |
Conclusions
A spindle-like BiVO4 was successfully synthesized using a pressure-controllable microwave method. The synthesis process was facile and rapid without the addition of any auxiliary reagent, adjustment of pH and annealing. It is found that the as-prepared spindle-like BiVO4 is a heterojunction composed of monoclinic scheelite BiVO4 and tetragonal zircon BiVO4. The existence of a heterojunction effectively promotes the separation of photo-generated carriers, which enhances the activity of spindle-like BiVO4 compared to that of monoclinic phase BiVO4. The photocatalytic degradation rates of RhB and TC over spindle-like BiVO4 are 1.77 and 1.64 times higher, respectively, than that over monoclinic BiVO4. Under visible-light illumination, the photo-induced electrons from the conduction band of t-BiVO4 migrate to the conduction band of m-BiVO4, while the photo-generated holes from the valence band of t-BiVO4 are transferred to the valence band of m-BiVO4. In this way, the photo-generated electron–hole pairs are effectively separated. The holes on the surface directly degrade RhB and TC.
Conflicts of interest
There are no conflicts of interest to declare.
Acknowledgements
This work is supported by the Postgraduate Research & Practice Innovation Program of Jiangsu Province (China) (SJKY19_2658, SJKY19_2629).
References
- S. Adhikari and D. Sarkar, Mater. Res. Bull., 2015, 72, 220–228 CrossRef CAS.
- M. Y. Li, Y. B. Tang, W. L. Shi, F. Y. Chen, Y. Shi and H. C. Gu, Inorg. Chem. Front., 2018, 5, 3148–3154 RSC.
- J. Xu, B. F. Luo, W. Gu, Y. P. Jian, F. L. Wu, Y. B. Tang and H. Shen, New J. Chem., 2018, 42, 5052–5058 RSC.
- F. P. Cai, Y. B. Tang, F. Y. Chen, Y. Yan and W. D. Shi, RSC Adv., 2015, 5, 21290–21296 RSC.
- Y. X. Wang, H. Wang, F. Y. Chen, F. Cao, X. H. Zhao, S. G. Meng and Y. J. Cui, Appl. Catal., B, 2017, 206, 417–425 CrossRef CAS.
- G. Jiang, X. Wang, Z. Wei and X. Li, J. Mater. Chem. A, 2013, 1, 2406–2410 RSC.
- G. Jiang, Z. Wei, H. Chen and X. X. Du, RSC Adv., 2015, 5, 30433–30437 RSC.
- G. Xi and J. Ye, Chem. Commun., 2010, 46, 1893–1895 RSC.
- Y. Wang, J. Huang, G. Tan, J. Huang and L. Zhang, Nano, 2015, 10, 78–86 Search PubMed.
- C. Lv, J. Sun, G. Chen, Y. Zhou and D. Li, Appl. Catal., B, 2017, 208, 14–21 CrossRef CAS.
- F. Wang, M. Shao, C. Liang, J. Hua and X. Wei, Mater. Res. Bull., 2009, 44, 1687–1691 CrossRef CAS.
- B. Liu, X. Yan, H. Yan, Y. Yao, Y. Cai, J. Wei, S. Chen, X. Xu and L. Li, Materials, 2017, 10, 976–986 CrossRef PubMed.
- D. Wang, H. Jiang, X. Zong, Q. Xu, Y. Ma, G. Li and C. Li, Chemistry, 2011, 17, 1275–1282 CrossRef CAS PubMed.
- H. Q. Jiang, H. Endo, H. Natori, M. Nagai and K. Kobayashi, J. Eur. Ceram. Soc., 2008, 28, 2955–2962 CrossRef CAS.
- H. B. Li, J. Zhang, G. Y. Huang, S. H. Fu, C. Ma, B. Y. Wang, Q. R. Huang and H. W. Liao, Trans. Nonferrous Met. Soc. China, 2017, 27, 868–875 CrossRef CAS.
- S. Liu, H. Tang, H. Zhou, G. Dai and W. Wang, Appl. Surf. Sci., 2016, 391, 542–547 CrossRef.
- L. Ye, L. Li, L. Guo, H. Peng and K. Chen, Mater. Lett., 2017, 221, 171–174 Search PubMed.
- Y. Lu, H. Shang, F. Shi, C. Chao, X. Zhang and B. Zhang, J. Phys. Chem. Solids, 2015, 85, 44–50 CrossRef CAS.
- D. T. Nguyen and S. S. Hong, Top. Catal., 2017, 60, 782–788 CrossRef CAS.
- N. D. Phu, L. H. Hoang, P. K. Vu, M. H. Kong, X. B. Chen, H. C. Wen and W. C. Chou, J. Mater. Sci.: Mater. Electron., 2016, 27, 6452–6456 CrossRef CAS.
- W. Li, X. Wang, Z. Wang, Y. Meng, X. Sun, T. Yan, J. You and D. Kong, Mater. Res. Bull., 2016, 83, 259–267 CrossRef CAS.
- W. Shi, Y. Yan and X. Yan, Chem. Eng. J., 2013, 215–216, 740–746 CrossRef CAS.
- S. Moscow and K. Jothivenkatachalam, J. Mater. Sci.: Mater. Electron., 2015, 27, 1433–1443 CrossRef.
- T. S. Dabodiya, P. Selvarasu and A. V. Murugan, Inorg. Chem., 2019, 58, 5096–5110 CrossRef CAS PubMed.
- Y. Wang, F. Liu, Y. Hua, C. Wang, X. Zhao, X. Liu and H. Li, J. Colloid Interface Sci., 2016, 483, 307–313 CrossRef CAS PubMed.
- A. K. Bhattacharya, K. K. Mallick and A. Hartridge, Mater. Lett., 1997, 30, 7–13 CrossRef CAS.
- B. Zhou, J. Qu, Z. Xu and H. Liu, J. Environ. Sci., 2011, 23, 151–159 CrossRef CAS.
- J. Su, X. X. Zou, G. D. Li, X. Wei, C. Yan, Y. N. Wang, J. Zhao, L. Zhou and J. Chen, J. Phys. Chem. C, 2011, 115, 8064–8071 CrossRef CAS.
- M. Wang, Y. S. Che, C. Niu, M. Dang and D. Dong, J. Rare Earths, 2013, 31, 878–884 CrossRef CAS.
- K. Sayama, A. Nomura, Z. Zou, R. Abe, Y. Abe and H. Arakawa, Chem. Commun., 2004, 2908–2909 Search PubMed.
- Z. Zhu, J. Du, J. Li, Y. Zhang and D. Liu, Ceram. Int., 2012, 38, 4827–4834 CrossRef CAS.
- Z. Lin, W. Wang, S. Liu, L. Zhang, H. Xu and W. Zhu, J. Mol. Catal. A: Chem., 2006, 252, 120–124 CrossRef.
- W. Y. Jung and S. S. Hong, J. Ind. Eng. Chem., 2013, 19, 157–160 CrossRef CAS.
- Z. H. Wei, Y. F. Wang, Y. Y. Li, L. Zhang, H. C. Yao and Z. J. Li, J. CO2 Util., 2018, 28, 15–25 CrossRef CAS.
- N. D. Phu, L. H. Hoang, P. K. Vu, M. H. Kong, X. B. Chen, H. C. Wen and W. C. Chou, J. Mater. Sci.: Mater. Electron., 2016, 27, 6452–6456 CrossRef CAS.
- X. Gao, H. B. Wu, L. Zheng, Y. Zhong, Y. Hu and X. W. Lou, Angew. Chem., Int. Ed., 2013, 53, 5917–5921 CrossRef PubMed.
|
This journal is © The Royal Society of Chemistry 2020 |
Click here to see how this site uses Cookies. View our privacy policy here.