DOI:
10.1039/C9RA07847A
(Paper)
RSC Adv., 2020,
10, 317-322
Elucidating π–π interaction-induced extension effect in sandwich phthalocyaninato compounds†
Received
27th September 2019
, Accepted 18th December 2019
First published on 2nd January 2020
Abstract
Electrochemical and theoretical investigations over triple-quadruple-, quintuple-, and sextuple-decker sandwich-type compounds {[(Pc*)Sm][(Pc*)Cdn(Pc*)n][Sm(Pc*)]} (n = 0–3) elucidate successive π–π interaction-linked extension in the perpendicular direction of the phthalocyanine plane along with increasing the stacked tetrapyrrole number, significantly improving the nonlinear optical properties including effective imaginary third order molecular hyperpolarizability and optical limiting threshold.
Introduction
Large conjugated organic molecules have attracted lots of academic interest in the field of electronics and photonics.1 In this direction, fusing monomers with conjugated electronic structures such as acene,2 pentarylenebis(dicarboximide),3 porphyrin,4 and polycyclic aromatic hydrocarbons5 through covalent bonds provides an efficient approach to access various extended systems.2–5 In addition to the extension in the monomer plane, expanding systems are also able to be realized along the perpendicular direction to the monomer plane via π–π interactions. This has been clearly demonstrated by metallocene sandwich compounds, μ-oxo bridged phthalocyanine compounds and the sandwich-type tetrapyrrole metal compounds.6 The latter species were fabricated by the complexation of large metal ion(s) with tetrapyrrole macrocycles, exhibiting effective inter-ring π–π interaction. The lack of multiple-decker sandwich-type tetrapyrrole compounds with more than three layer stacking structure leads to the rare investigation over their expanding effect originated from intramolecular π–π interactions. Recently, tetrakis-, pentakis-, and hexakis(tetrapyrrole) rare earth-cadmium compounds with quadruple-, quintuple-, and sextuple-decker molecular configuration just prepared by the smart use of divalent cadmium ions.7
In the present case, it is a good opportunity to systematically explore the π–π interaction-linked extension effect of sandwich-type compounds. To this end, a series of sandwich compounds {[(Pc*)Sm][(Pc*)Cdn(Pc*)n][Sm(Pc*)]} (n = 0–3) (3–6) have been devised and prepared, Scheme 1. Comparative electrochemical and theoretical studies clearly reveal the effective π–π interaction-induced electronic structure change in the expanding system for 3–6 along with increasing the stacked tetrapyrrole number in the direction perpendicular to phthalocyanine chromophore, which in turn improves their nonlinear optical properties as demonstrated by the nonlinear absorption coefficient and optical limiting threshold.
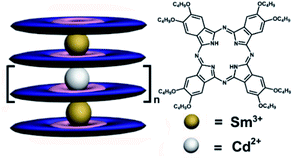 |
| Scheme 1 Schematic molecular structures of tris-to hexakis(phthalocyaninato) metal complexes {[(Pc*)Sm][(Pc*)Cdn(Pc*)n][Sm(Pc*)]} (n = 0–3) (3–6). | |
Results and discussion
Synthesis of 3–6
Homometallic tris[2,3,9,10,16,17,23,24-octa(butyloxy)phthalocyaninato] samarium(III) triple-decker complex (Pc*)Sm(Pc*) Sm(Pc*) (3) was isolated in a yield of 50% by monomeric phthalocyanine, 2,3,9,10,16,17,23,24-octa(butyloxy)phthalocyanine (Pc*), reacting with samarium(III) ions in 1,2-4-trichlorobenzene (TCB), Fig. S1 (ESI†). For 4–6, these compounds were able to be generated form the reaction between Sm(Pc*)2 and Cd(OAc)2 in TCB at 220 °C due to the pyrolysis of double-decker compound into monomeric phthalocyanine, leading to the complicated assembly between monomeric compound, double-decker and divalent cadmium ion, Fig. S2 (ESI†). In this process, some unstable species with multiple-decker configuration with more than six-deck structure was monitored, however, were too unable to be separated. For 5 and 6, they are known compound, and their synthesis, characterization and purity were reported before.7a,7b
Electronic absorption spectra of 3–6
The electronic absorption spectra of 3–6 recorded in toluene are shown in Fig. 1. For the triple-decker compound 3, the absorption at 362 nm with a shoulder band at 338 nm is assigned to the phthalocyanine Soret band, and a main band at 646 with two shoulder bands at 592 and 688 nm belongs to the phthalocyanine Q bands. Increasing the number of stacking phthalocyanine via a bridging divalent cadmium ion leads to the slight red-shift of Soret band (354 nm) as well as a blue-shift of main Q band (634 nm) in quadruple-decker species 4 in comparison with that of 3. The similar molar absorbance coefficients for corresponding bands between 3 and 4 indicate that the addition of a phthalocyanine ligand and one divalent cadmium ion gives a new expanding system. Further introducing phthalocyanine on the multiple-decker sandwich-type complexes, the Soret and Q bands (346 and around 640 nm, respectively) of 5 and 6 were significantly broadened, and their molar absorbance coefficients were seriously reduced due mostly to the twist angle difference originating from the complicated inter-ring π–π interactions in these compounds.5b In addition, these results indicate that all phthalocyanine ligands in multiple-decker sandwich-type complexes are effectively integrated into a molecular system.
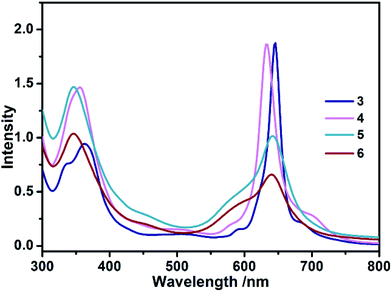 |
| Fig. 1 UV-vis spectra of 3–6 with the concentration of 5.0 × 10−6 mol L−1 in toluene. | |
Electrochemical behaviors of 3–6
The electrochemical behaviors of 3–6 were determined by cyclic voltammetry in CH2Cl2. As detailed in Fig. S3 and Table S1 (ESI†), these compounds exhibits three/four ligand-associated oxidation potentials together with two reduction potentials. The first oxidation potential (corresponding to the HOMO energy level) gradually decreases from 0.33 V for 3, 0.22 V for 4, 0.12 V for 5, to 0.06 V for 6, while the first reduction potential (corresponding to the LUMO energy level) similarly diminishes from −0.85 V for 3, −0.95 V for 4, −1.05 V for 5, to −1.09 V for 6, revealing the increase of the respective HOMO and LUMO energy level along with the increase in the stacked phthalocyanine-deck number. These results clearly disclose the presence of the perpendicular extension within the sandwich-type compounds in the direction to the tetrapyrrole plane following the increased stacking number of phthalocyanine. As a consequence, the HOMO–LUMO gap deduced from the difference of the first oxidation and first reduction potentials does not change significantly along with the increase of the stacked phthalocyanine-deck number most probably associated with the effective π–π interaction-induced extension effect,1a which only very slightly decreases from 1.18 V for 3, 1.17 V for 4, 1.17 V for 5, to 1.15 V for 6, Table S4 (ESI†). In addition, on the basis of the plots of the first oxidation and first reduction potentials versus the reciprocal of the conjugated stacked phthalocyanine number N for the series of {[(Pc*)Sm][(Pc*)Cdn(Pc*)n][Sm(Pc*)]} (n = 0–3) (3–6), Fig. 2, the first oxidation and reduction potentials (−0.20 and −1.34 V, respectively) of the extended sandwich-type species with “infinite” stacked-deck number were estimated from the intercept values of the fitting plots.4a The deduced HOMO–LUMO gap of 1.14 V indicates the presence of saturation effect for HOMO–LUMO gap of 5.
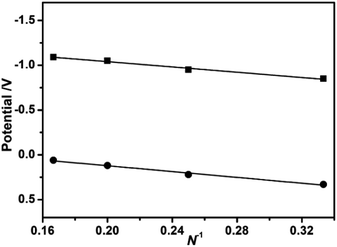 |
| Fig. 2 Plots of the first oxidation and reduction potentials [vs. SCE] as a function of the reciprocal of the stacked phthalocyanine-deck number for 3–6. | |
Theoretical calculations
To further confirm the π–π interaction-induced extension effect of 3–6 along with the increase of the phthalocyanine-deck number, density functional theory (DFT) calculations were carried out on the series of sandwich-type derivatives {[(Pc)La(Pc)Cdn(Pc)n][La(Pc)]} (n = 0–2) (a–c) at the level of B3LYP/LanL2DZ (ESI†).8 The frontier orbital couplings of the intramolecular phthalocyanines and corresponding energy levels for the series of sandwich-type complexes with triple-, quadruple-, and quintuple-decker molecular structure are shown in Fig. S4–S7 (ESI†). As can be seen, the frontier coupling orbitals of these multiple-decker compounds are originated from the HOMO/LUMO(x)/LUMO(y) of the monomeric phthalocyanine rings with doping very tiny metal component, leading to the delocalized configuration over the sandwich system for the multi(phthalocyaninato) metal triple-, quadruple-, and quintuple-decker complexes.9 According to the calculation, the HOMO energy level increases from −4.94 eV for (Pc)La(Pc)La(Pc) (a), −4.78 eV for (Pc)La(Pc)Cd(Pc)La(Pc) (b), −4.68 eV for (Pc)La(Pc)Cd(Pc)Cd(Pc)La(Pc) (c), and the LUMO energy level similarly raises from −3.34 eV for a, −3.30 eV for b, −3.27 eV for c, resulting in a diminished HOMO–LUMO gap from 1.60 eV for a, 1.48 eV for b, to 1.39 eV for c. These results well explain experimental findings about the decrease of HOMO–LUMO gap from 3 to 5. Herein, it is worth noting that the trial for calculation over the sandwich-type hexakis(phthalocyaninato) lanthanum(III)–cadmium(II) sextuple-decker compound (Pc)La(Pc)Cd(Pc)Cd(Pc)Cd(Pc)La(Pc) (C192H96Cd3La2N48) were failed due to the limited calculating ability for the present system containing 341 atoms.
Nonlinear optical properties of 3–6
The third order nonlinear optical properties of the series of sandwich-type complexes 3–6 in toluene were comparatively studied by employing Z-scan technique with 532 nm Nd-YAG laser as light source with 5 ns pulse width, and the results are shown in Table 1. Concentration-dependent reverse saturation absorption curves have been observed for the quintuple-decker compound 5, as a representative of 3–6. The third order molecular hyperpolarizability of 5 is increased following the concentration from 1.0 × 10−5, 2.0 × 10−5 to 1.2 × 10−4 mol L−1 in toluene under an open-aperture configuration, Fig. 3a. This is also true for the other three sandwich-type complexes. The effective imaginary third order molecular hyperpolarizability (Im{χ(3)}) as the nonlinear refractive component of 3–6 was deduced by using the popular formula.10 The plot of the (Im{χ(3)}) at the concentration of 1.2 × 10−4 mol L−1 as a function of the stacked phthalocyanine-deck number, N, in the multi(phthalocyaninato) samarium–cadmium compounds {[(Pc*)Sm][(Pc*)Cdn(Pc*)n][Sm(Pc*)]} (n = 0–3) (3–6) clearly shows a linear relationship with the fitting equation of Im{χ(3)} = 9.37 × 10−12N + 1.16 × 10−12 where R = 0.999, Fig. 3b, revealing the effect of the π–π interaction-induced expansion in the perpendicular direction of the phthalocyanine chromophore on the third order nonlinear optical properties of the series of sandwich-type complexes. This result is in line with that revealed for the one-dimensional covalently-linked extended systems with coplanar structure.11
Table 1 The effective imaginary third order molecular hyperpolarizability (Im{χ(3)}) data for the series of sandwich-type complexes 3–6
Concentration |
(Im{χ(3)})/esu |
3 |
4 |
5 |
6 |
1.0 × 10−5 mol L−1 |
5.31 × 10−12 |
5.39 × 10−12 |
5.84 × 10−12 |
5.98 × 10−12 |
2.0 × 10−5 mol L−1 |
5.76 × 10−12 |
5.86 × 10−12 |
6.88 × 10−12 |
1.04 × 10−11 |
1.2 × 10−4 mol L−1 |
1.29 × 10−11 |
1.41 × 10−11 |
1.61 × 10−11 |
1.91 × 10−11 |
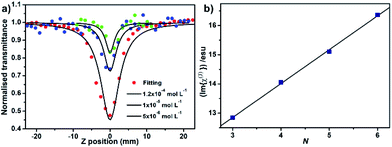 |
| Fig. 3 (a) NLO absorptive property of 5 in toluene solution under an open-aperture configuration. (b) Plot of (Im{χ(3)}) for at the concentration of 1.2 × 10−4 mol L−1 in toluene, as a function of the stacked phthalocyanine-deck number, N, in {[(Pc*)Sm][(Pc*)Cdn(Pc*)n][Sm(Pc*)]} (n = 0–3) (3–6). | |
Optical limiting properties of 3–6
For the purpose of further confirming the effect of the π–π interaction-induced expansion on the nonlinear optical properties of the sandwich-type complexes, the optical limiting (OL) properties of 3–6 in toluene with the initial transmittance of 0.7 at 532 nm have also been systematically probed with In(Pc*)Cl as reference. Similar to the reference compound, the whole series of sandwich-type compounds {[(Pc*)Sm][(Pc*)Cdn(Pc*)n][Sm(Pc*)]} (n = 0–3) (3–6) exhibit characteristic transmittance profile of OL materials with the linear transmittance at 0.7 before ca. 100 mJ cm−2 laser input fluence, Fig. 4, which however gets decreased along with the further increase of the input fluence. The OL threshold, denoted as the incident energy at which the transmittance is half of the initial linear transmittance,12 was revealed to be ca. 0.40, 0.32, 0.28, and 0.37 mJ cm−2 for 3–6, respectively. The OL threshold of the sandwich-type compounds gets decrease along with the increase in the phthalocyanine-deck number in the order from 3, 4, to 5 due to the fast-cooling and recombination in photo-induced electron–hole gas mechanism.13 The slightly weaker OL performance of sextuple-decker 6 relative to that of the quintuple-decker 5 is not clear at this stage but most probably associated with the factors including concentration difference, twist angle, and odd–even difference in the stacked phthalocyanine deck number of the sandwich-type complexes. However, it is worth noting that the OL threshold for the series of sandwich-type multi(phthalocyaninato) metal complexes, in particular the quintuple-decker 5 is comparable with that for the excellent optical material In(Pc*)Cl, 0.27 mJ cm−2, obtained under the same experimental condition, clearly revealing the potential of these multi(phthalocyaninato) metal sandwich-type complexes as effective OL materials along with increasing the stacked phthalocyanine-deck number.
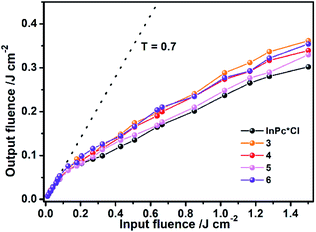 |
| Fig. 4 OL properties of 3–6 in toluene with the initial transmittance (T) of 0.7. | |
Experimental
Note: All the reagents and solvents were used as received. The compounds H2Pc* was prepared according to the published procedure.5m
Physical measurement
1H NMR spectra were recorded on a Bruker DPX 400 spectrometer in CDCl3. Spectrum was referenced internally using the residual solvent resonances (δ = 7.26 for 1H NMR). Electronic absorption spectra were recorded on a Hitachi U-4100 spectrophotometer. MALDI-TOF mass spectra were taken on a Bruker BIFLEX III ultra high resolution Fourier transform ion cyclotron resonance (FT-ICR) mass spectrometer with alpha-cyano-4-hydroxycinnamic acid as matrix. Elemental analysis was performed on an Elementar Vario El III. The open aperture Z-scan measurements and optical limiting property were carried out using a nanosecond laser at 532 nm.
Synthesis of Sm[Pc*]2
A mixture of 4,5-dibutoxyphthalonitrile (300 mg, 0.28 mmol), Sm(acac)3·xH2O (80 mg), n-pentanol (5 mL), and DBU (50 μL) was heated at 150 °C under nitrogen for 8 h. After being cooled to room temperature, the organic solvent was removed under reduced pressure. The residue was subjected to chromatography on a silica-gel column using CHCl3 as eluent. Following the green fraction containing H2Pc*, dark green band containing double-decker Sm[Pc*]2 was obtained. The crude product was purified by repeated chromatography followed by recrystallization from CHCl3/MeOH in a yield of 15%. Due to the sample containing two kinds of oxidation states, the NMR spectrum was not collected.
Synthesis of [(Pc*)Sm(Pc*)Sm(Pc*)] (3)
A mixture of metal-free 2,3,9,10,16,17,23,24-octa(butyloxy)phthalocyanine (30 mg, 0.028 mmol) and Sm(acac)3·xH2O (12 mg) in dry 1,2-4-trichlorobenzene (TCB) (2 mL) was heated to reflux under nitrogen for 5.0 h. After being cooled to room temperature, the volatiles were removed under reduced pressure. The residue was chromatographed on a silica gel column using CHCl3 as the eluent to give a green band firstly, which contained the unreacted H2Pc* and double-decker compound Sm(Pc*)2. Further elution with CHCl3/CH3OH (95
:
5) gave a blue band containing the triple-decker complex 3. Further repeated biobead column chromatography followed by recrystallization from chloroform and methanol gave the pure target heterometallic tetrakis[2,3,9,10,16,17,23,24-octa(butyloxy)phthalocyaninato] samarium complex in the yield of 50%. δ 7.37 (s, 16H, Hα for outer Pc*), 6.53 (s, 8H, Hα for center Pc*), 4.27 (m, 16H, –OCH2– for center Pc*), 4.10 (m, 6.3 Hz, 32H, –OCH2– for outer Pc*), 2.08 (m, 16H, –OCH2CH2– for center Pc*), 1.95 (m, 32H, –OCH2CH2– for center Pc*), 1.80 (m, 16H, –O(CH2)2CH2– for center Pc*), 1.63 (m, 32H, –O(CH2)2CH2– for outer Pc*), 1.22 (t, 24H, –O(CH2)3CH3 for center Pc*), 1.09 (t, 48H, –O(CH2)3CH3 for outer Pc*). MALDI-TOF MS: an isotopic cluster peaking at m/z 3568.90, calcd for C132H114N12, [M]+ 3568.83. Anal. calcd for C192H240N24O24Sm2%: C 64.62; H 6.78; N 9.42. Found: C 64.12; H 6.42; N 9.68. λmax nm (log
ε) in toluene: 338(5.18), 362(5.28), 592(4.49), 646(5.57) and 688(4.61).
Synthesis of [(Pc*)Sm(Pc*)Cd(Pc*)Sm(Pc*)] (4)
A mixture of Cd(OAc)2·2H2O (5.3 mg, 0.02 mmol) and neutral bis[2,3,9,10,16,17,23,24-octa(butyloxy)phthalocyaninato] samrium double-decker compound Sm(Pc*)2 (0.04 mmol) in dry TCB (3 mL) was heated to reflux under nitrogen for 2.0 h. After being cooled to room temperature, the volatiles were removed under reduced pressure. The residue was chromatographed on a silica gel column using CHCl3 as the eluent to give a green band, which contained the unreacted Sm(Pc*)2. Further elution with CHCl3/CH3OH (95
:
5) gave a blue band containing the quadruple-decker complex {[(Pc*)Sm][(Pc*)Cd(Pc*)n][Sm(Pc*)]} (4). Further repeated biobead column chromatography followed by recrystallization from chloroform and methanol gave the pure target heterometallic tetrakis[2,3,9,10,16,17,23,24-octa(butyloxy)phthalocyaninato] samarium–cadmium quadruple-decker complex 4 in the yield of 26%. Some species including 5, 6, and other unknown multiple-deckers will also be generated due to the pyrolysis of double-decker compound Sm(Pc*)2. 5 and 6 were separated by using biobead column chromatography and the recrystallization from chloroform and methanol with the yield of 10 and 6%, respectively. 1H NMR (CDCl3, 400 MHz): δ 7.13 (s, 16H, Hα for outer Pc*), 6.82 (s, 16H, Hα for center Pc*), 4.22–3.98 (m, 64H, –OCH2– for center and outer Pc*), 2.17 (m, 32H, –OCH2CH2– for center Pc*), 1.92 (m, 64H, –OCH2CH2– for outer Pc* and –O(CH2)2CH2– for center Pc*), 1.61 (m, 32H, –O(CH2)2CH2– for outer Pc*), 1.31 (t, 48H, –O(CH2)3CH3 for center Pc*), 1.07 (t, 48H, –O(CH2)3CH3 for outer Pc*). MALDI-TOF MS: an isotopic cluster peaking at m/z 4770.68, calcd for C132H114N12, [M]+ 4770.61. Anal. calcd for C256H320CdN32O32Sm2·CHCl3: C 63.12; H 6.61; N 9.16. Found: C 63.35; H 6.90; N 9.18. λmax nm (log
ε) in toluene: 354(5.46), 580(4.59), 634(5.57) and 702(4.74).
Synthesis of [(Pc*)Sm(Pc*)Cd(Pc*)Cd(Pc*)Sm(Pc*)] (5) and [(Pc*)Sm(Pc*)Cd(Pc*)Cd(Pc*)Cd(Pc*)Sm(Pc*)] (6)
These two compounds were prepared according to the published procedures.7a,7b For 5, λmax nm (log
ε) in toluene: 346(5.42) and 642(5.24). For 6, λmax nm (log
ε) in toluene: 346(5.32) and 640(5.12).
Computational details
Density functional theory (DFT) of hybrid B3LYP functional with Becke exchange8a and Lee–Yang–Parr correlation8b has been employed to calculate the molecular orbitals of a series of multi-deckers. In all cases, the LanL2DZ basis set, which applies Dunning–Huzinaga full double-ξ (D95) basis functions on first-row elements and Los Alamos effective core potentials plus DZ functions on all other atoms, was used.8c–8e For the reason of time efficiency, Sm(III) ion was replaced by La(III) ion, meanwhile the substitute groups were also deleted in the sandwich-type stacked tetrapyrrole metal complexes 3–5. All the calculations were carried out using the Gaussian 03 program.14
Conclusions
In a summary, perpendicular π–π interaction-induced extension within a series of sandwich-type tetrapyrrole complexes have been clearly confirmed by electrochemical and theoretical investigations. Such effective extension plays an important role in the improvement of their nonlinear optical properties. These results not only introduce unique π–π interaction-induced extension effect in influencing the electronic structures of sandwich-type tetrapyrrole compounds, but also evidence such extension effect on the molecular functions, which will be helpful for the design, of novel π–π interaction-involving compounds/materials towards the practical applications.
Conflicts of interest
There are no conflicts to declare.
Acknowledgements
Financial support from the Natural Science Foundation of China (No. 21631003, 21805005, and 21671017), the Fundamental Research Funds for the Central Universities (No. FRF-BD-17-016A and FRF-BR-18-009B), the joint research project between University of Science and Technology Beijing and National Taipei University of Technology (TW2019008), and University of Science and Technology Beijing is gratefully acknowledged. This work is also supported by the projects from NTUT-USTB-108-12 between National Taipei University of Technology and University of Science and Technology Beijing and the project of MOST 107-2113-M-027-005-MY3.
Notes and references
-
(a) R. E. Martin and F. Diederich, Angew. Chem., Int. Ed., 1999, 38, 1350 CrossRef;
(b) F. Chen and N. J. Tao, Acc. Chem. Res., 2009, 42, 429 CrossRef CAS PubMed;
(c) J. Wu, W. Pisula and K. Müllen, Chem. Rev., 2007, 107, 718 CrossRef CAS PubMed;
(d) J. E. Anthony, Chem. Rev., 2006, 106, 5028 CrossRef CAS PubMed;
(e) D. Kim and A. Osuka, Acc. Chem. Res., 2004, 37, 735 CrossRef CAS PubMed.
-
(a) J. Xiao, H. M. Duong, Y. Liu, W. Shi, L. Ji, G. Li, S. Li, X.-W. Liu, J. Ma, F. Wudl and Q. Zhang, Angew. Chem., Int. Ed., 2012, 51, 6094 CrossRef CAS PubMed;
(b) Y. Wang, S. Qiu, S. Xie, L. Zhou, Y. Hong, J. Chang, J. Wu and Z. Zeng, J. Am. Chem. Soc., 2019, 141, 2169 CrossRef CAS PubMed;
(c) B. Purushothaman, M. Bruzek, S. R. Parkin, A.-F. Miller and J. E. Anthony, Angew. Chem., Int. Ed., 2011, 50, 7013 CrossRef CAS PubMed;
(d) Z. Liu, J. S. A. Ishibashi, C. Darrigan, A. Dargelos, A. Chrostowska, B. Li, M. Vasiliu, D. A. Dixon and S.-Y. Liu, J. Am. Chem. Soc., 2017, 139, 6082 CrossRef CAS PubMed.
-
(a) Arramel, X. Yin, Q. Wang, Y. J. Zheng, Z. Song, M. H. b. Hassan, D. Qi, J. Wu, A. Rusydi and A. T. S. Wee, ACS Appl. Mater. Interfaces, 2017, 9, 5566 CrossRef CAS PubMed;
(b) H. Qian, F. Negri, C. Wang and Z. Wang, J. Am. Chem. Soc., 2010, 130, 17970 CrossRef PubMed;
(c) W. Jiang, C. Xiao, L. Hao, Z. Wang, H. Ceyamann, C. Lambert, S. D. Motta and F. Negri, Chem.–Eur. J., 2012, 18, 6764 CrossRef CAS PubMed;
(d) L. Yang, P. Langer, E. S. Davies, M. Baldoni, K. Wickham, N. A. Besley, E. Besley and N. R. Champness, Chem. Sci., 2019, 10, 3723 RSC.
-
(a) A. Tsuda and A. Osuka, Science, 2001, 293, 79 CrossRef CAS PubMed;
(b) H.-X. Wang, Q. Wan, K. Wu, K.-H. Low, C. Yang, C.-Y. Zhou, J.-S. Huang and C.-M. Che, J. Am. Chem. Soc., 2019, 141, 9027 CrossRef CAS PubMed;
(c) Y. Nakamura, S. Y. Jang, T. Tanaka, N. Aratani, J. M. Lim, K. S. Kim, D. Kim and A. Osuka, Chem.–Eur. J., 2008, 14, 8279 CrossRef CAS PubMed;
(d) K. Kato, K. Furukawa and A. Osuka, Angew. Chem., Int. Ed., 2018, 57, 9491 CrossRef CAS PubMed;
(e) R. Liu, C. Malotki, L. Arnold, N. Koshino, H. Higashimura, M. Baumgarten and K. Müllen, J. Am. Chem. Soc., 2011, 133, 10372 CrossRef CAS PubMed;
(f) M. J. Crossley and P. L. Burn, J. Chem. Soc., Chem. Commun., 1991, 1569 RSC;
(g) X. Yang, X. Dou, A. Rouhanipour, L. Zhi, H. J. Räder and K. Müllen, J. Am. Chem. Soc., 2008, 130, 4216 CrossRef CAS PubMed;
(h) J. I. Urgel, S. Mishra, H. Hayashi, J. Wilhelm, C. A. Pignedoli, M. Di Giovannantonio, R. Widmer, M. Yamashita, N. Hieda, P. Ruffieux, H. Yamada and R. Fasel, Nat. Commun., 2019, 10, 861 CrossRef PubMed;
(i) M. Kastler, J. Schmidt, W. Pisula, D. Sebastiani and K. Müllen, J. Am. Chem. Soc., 2006, 128, 9526 CrossRef CAS PubMed;
(j) Q. Jiang, T. Tao, H. Phan, Y. Han, T. Y. Gopalakrishna, T. S. Herng, G. Li, L. Yuan, J. Ding and C. Chi, Angew. Chem., Int. Ed., 2018, 57, 16737 CrossRef CAS PubMed;
(k) J. Liu, B.-W. Li, Y.-Z. Tan, A. Giannakopoulos, C. Sanchez-Sanchez, D. Beljonne, P. Ruffieux, R. Fasel, X. Feng and K. Müllen, J. Am. Chem. Soc., 2015, 137, 6097 CrossRef CAS PubMed.
-
(a) D. Pun, E. Lobkovsky and P. J. Chirik, J. Am. Chem. Soc., 2008, 130, 6047 CrossRef CAS PubMed;
(b) A. N. Cammidge, F. Nekelson, M. Helliwell, M. J. Heeney and M. J. Cook, J. Am. Chem. Soc., 2005, 127, 16382 CrossRef CAS PubMed;
(c) J. Jiang and D. K. P. Ng, Acc. Chem. Res., 2009, 42, 79 CrossRef CAS PubMed;
(d) J. Jiang, M. Bao, L. Rintoul and D. P. Arnold, Coord. Chem. Rev., 2006, 250, 424 CrossRef CAS;
(e) J. Jiang, K. Kasuga and D. P. Arnold, Supramolecular Photo-Sensitive and Electroactive Materials ed. H. S. Nalwa, Academic Press, New York, 2001, pp. 113–210 Search PubMed;
(f) D. K. P. Ng and J. Jiang, Chem. Soc. Rev., 1997, 26, 433 RSC;
(g) J. Buchler and D. K. P. Ng, The Porphyrin Handbook ed. K. M. Kadish, K. M. Smith, and R. Guilard, Academic Press, New York, 2000, pp. 245–290 Search PubMed;
(h) Z. Liu, A. A. Yasseri, J. S. Lindsey and D. F. Bocian, Science, 2003, 302, 1543 CrossRef CAS PubMed;
(i) J. S. Lindsey and D. F. Bocian, Acc. Chem. Res., 2011, 8, 638 CrossRef PubMed;
(j) G. De La Torre, P. Vazquez, F. Agullo-Lopez and T. Torres, Chem. Rev., 2004, 104, 3723 CrossRef CAS PubMed;
(k) H. Wang, B.-W. Wang, Y. Bian, S. Gao and J. Jiang, Coord. Chem. Rev., 2016, 306, 195 CrossRef CAS;
(l) V. E. Pushkarev, L. G. Tomilova and V. N. Nemykin, Coord. Chem. Rev., 2016, 319, 110 CrossRef CAS;
(m) Y. Horii, K. Katoh, N. Yasuda, B. K. Breedlove and M. Yamashita, Inorg. Chem., 2015, 54, 3297 CrossRef CAS PubMed.
-
(a) P. Zhu, N. Pan, R. Li, J. Dou, Y. Zhang, D. Y. Y. Cheng, D. Wang, D. K. P. Ng and J. Jiang, Chem.–Eur. J., 2005, 11, 1425 CrossRef CAS PubMed;
(b) N. Ishikawa, S. Otsuka and Y. Kaizu, Angew. Chem., Int. Ed., 2005, 44, 731 CrossRef CAS PubMed;
(c) W. Liu, H. Pan, Z. Wang, K. Wang, D. Qi and J. Jiang, Chem. Commun., 2017, 53, 3765 RSC;
(d) Y. Bian, J. Jiang, Y. Tao, M. T. M. Choi, R. Li, A. C. H. Ng, P. Zhu, N. Pan, X. Sun, D. P. Arnold, Z. Zhou, H.-W. Li, T. C. W. Mak and D. K. P. Ng, J. Am. Chem. Soc., 2003, 125, 12257 CrossRef CAS PubMed;
(e) W. Cao, C. Gao, Y.-Q. Zhang, D. Qi, T. Liu, K. Wang, C. Duan, S. Gao and J. Jiang, Chem. Sci., 2015, 6, 5947 RSC;
(f) R. Wang, R. Li, Y. Li, X. Zhang, P. Zhu, P.-C. Lo, D. K. P. Ng, N. Pan, C. Ma, N. Kobayashi and J. Jiang, Chem.–Eur. J., 2006, 12, 1475 CrossRef CAS PubMed;
(g) X. Zhang, A. Muranaka, W. Lv, Y. Zhang, Y. Bian, J. Jiang and N. Kobayashi, Chem.–Eur. J., 2008, 14, 4667 CrossRef CAS PubMed;
(h) J. Kan, Y. Chen, D. Qi, Y. Liu and J. Jiang, Adv. Mater., 2012, 24, 1755 CrossRef CAS PubMed;
(i) K.-H. Schweikart, V. L. Malinovskii, J. R. Diers, A. A. Yasseri, D. F. Bocian, W. G. Kuhr and J. S. Lindsey, J. Mater. Chem., 2002, 12, 808 RSC;
(j) Y. Horii, K. Katoh, B. K. Breedlove and M. Yamashita, Chem. Commun., 2017, 53, 8561 RSC.
-
(a) H. Wang, N. Kobayashi and J. Jiang, Chem.–Eur. J., 2012, 18, 1047 CrossRef CAS PubMed;
(b) H. Wang, D. Qi, Z. Xie, W. Cao, K. Wang, H. Shang and J. Jiang, Chem. Commun., 2013, 49, 889 RSC;
(c) H. Wang, K. Wang, Y. Bian, J. Jiang and N. Kobayashi, Chem. Commun., 2011, 47, 6879 RSC;
(d) H. Wang, K. Qian, K. Wang, Y. Bian, J. Jiang and S. Gao, Chem. Commun., 2011, 9624 RSC;
(e) Y. Horii, K. Katoh, K. Sugimoto, R. Nakanishi, B. K. Breedlove and M. Yamashita, Chem.–Eur. J., 2019, 25, 3098 CAS;
(f) T. Fukuda, T. Biyajima and N. Kobayashi, J. Am. Chem. Soc., 2010, 132, 6278 CrossRef CAS PubMed;
(g) K. Katoh, T. Morita, N. Yasuda, W. Wernsdorfer, Y. Kitagawa, B. K. Breedlove and M. Yamashita, Chem.–Eur. J., 2018, 24, 15522 CrossRef CAS PubMed.
-
(a) A. D. Becke, J. Chem. Phys., 1993, 98, 5648 CrossRef CAS;
(b) C. Lee, W. R. Yang and G. Parr, Phys. Rev., 1988, B37, 785 CrossRef PubMed;
(c) P. J. Hay and W. R. Wadt, J. Chem. Phys., 1985, 82, 270 CrossRef CAS;
(d) W. R. Wadt and P. J. Hay, J. Chem. Phys., 1985, 82, 284 CrossRef CAS;
(e) P. J. Hay and W. R. Wadt, J. Chem. Phys., 1985, 82, 299 CrossRef CAS;
(f) Y. Zhang, X. Cai, D. Qi, P. Yao, Y. Bian and J. Jiang, ChemPhysChem, 2008, 9, 781 CrossRef CAS PubMed.
-
(a) Y. Zhang, X. Cai, P. Yao, H. Xu, Y. Bian and J. Jiang, Chem.–Eur. J., 2008, 9, 781 CAS;
(b) Y. Zhang, X. Cai, Y. Zhou, X. Zhang, H. Xu, Z. Liu, X. Li and J. Jiang, J. Phys. Chem. A, 2007, 111, 392 CrossRef CAS PubMed;
(c) D. Qi, L. Zhang, L. Wan, Y. Zhang, Y. Bian and J. Jiang, Phys. Chem. Chem. Phys., 2011, 13, 13277 RSC.
- M. Sheik-Bahae, A. A. Said, T.-H. Wei, D. J. Hagan and E. W. Van Stryland, IEEE J. Quantum Electron., 1990, 26, 760 CrossRef CAS.
-
(a) M. Rumi, G. Zerbi, K. Müllen, G. Müller and M. Rehahn, J. Chem. Phys., 1997, 106, 24 CrossRef CAS;
(b) D. Dini, M. J. F. Calvete and M. Hanack, Chem. Rev., 2016, 116, 13043 CrossRef CAS PubMed.
-
(a) Y. Li, T. M. Pritchett, J. Huang, M. Ke, P. Shao and W. Sun, J. Phys. Chem. A, 2008, 112, 7200 CrossRef CAS PubMed;
(b) Y. Chen, M. Hanack, Y. Arakic and O. Ito, Chem. Soc. Rev., 2005, 34, 517 RSC.
- G.-K. Lim, Z.-L. Chen, J. Clark, R. G. S. Goh, W.-H. Ng, H.-W. Tan, R. H. Friend, P. K. H. Ho and L.-L. Chua, Nat. Photonics, 2011, 5, 554 CrossRef CAS.
- M. J. Frisch, G. W. Trucks, H. B. Schlegel, G. E. Scuseria, M. A. Robb, J. R. Cheeseman Jr, J. A. Montgomery, T. Vreven, K. N. Kudin, J. C. Burant, J. M. Millam, S. S. Iyengar, J. Tomasi, V. Barone, B. Mennucci, M. Cossi, G. Scalmani, N. Rega, G. A. Petersson, H. Nakatsuji, M. Hada, M. Ehara, K. Toyota, R. Fukuda, J. Hasegawa, M. Ishida, T. Nakajima, Y. Honda, O. Kitao, H. Nakai, M. Klene, X. Li, J. E. Knox, H. P. Hratchian, J. B. Cross, V. Bakken, C. Adamo, J. Jaramillo, R. Gomperts, R. E. Stratmann, O. Yazyev, A. J. Austin, R. Cammi, C. Pomelli, J. W. Ochterski, P. Y. Ayala, K. Morokuma, G. A. Voth, P. Salvador, J. J. Dannenberg, V. G. Zakrzewski, S. Dapprich, A. D. Daniels, M. C. Strain, O. Farkas, D. K. Malick, A. D. Rabuck, K. Raghavachari, J. B. Foresman, J. V. Ortiz, Q. Cui, A. G. Baboul, S. Clifford, J. Cioslowski, B. B. Stefanov, G. Liu, A. Liashenko, P. Piskorz, I. Komaromi, R. L. Martin, D. J. Fox, T. Keith, M. A. Al-Laham, C. Y. Peng, A. Nanayakkara, M. Challacombe, P. M. W. Gill, B. Johnson, W. Chen, M. W. Wong, C. Gonzalez and J. A. Pople, Gaussian03, Revision B.05, Gaussian, Inc., Pittsburgh, PA, 2003 Search PubMed.
Footnotes |
† Electronic supplementary information (ESI) available: 1H NMR spectrum of 4 and open aperture Z-scan results. See DOI: 10.1039/c9ra07847a |
‡ These two authors contributed equally to this study. |
|
This journal is © The Royal Society of Chemistry 2020 |