DOI:
10.1039/C9QO01318K
(Research Article)
Org. Chem. Front., 2020,
7, 329-339
Diversity-oriented approach to functional thiophene dyes by Suzuki coupling-lithiation one-pot sequences†
Received
29th October 2019
, Accepted 3rd December 2019
First published on 4th December 2019
Abstract
Functional thiophenes, e.g. for organic metal-free dye sensitized solar cells (DSSC), are accessible efficiently via a divergent and diversity-oriented synthetic strategy. Here, we present two straightforward, modular and comparative one-pot syntheses of diversely functionalized thiophenes with high yields starting from simple molecules, namely Suzuki-Lithiation–Formylation-Knoevenagel (SLiForK) sequence and Suzuki-Lithiation–Borylation-Suzuki (SLiBS) sequence. These methods open new avenues to interesting thiophene structure motives with potential applications as pharmaceutical active agents or in molecular electronics, which we have elucidated by a substance library of 21 diversely, mostly unsymmetrically substituted thiophenes. Finally, three novel DSSC dyes were synthesized according to the developed one-pot protocols. For illustration, DSSC devices sensitized by the selected dyes revealed promising solar cell performances, which were rationalized by photophysical and electrochemical characterization and DFT calculations.
Introduction
The efficient synthesis of functional π-systems1 has garnered considerable attention due to the growing interest in molecular electronics. Many applications of these molecule based technologies, which become increasingly significant for everyday life, like organic photovoltaics (OPV) and organic dye-sensitized solar cells (DSSC),2–7 organic field-effect transistors (OFET),8–10 or organic light emitting diodes (OLED),11–13 constantly demand new structures. Thiophenes are one of the most important heterocyclic building blocks in functional molecules due to their outstanding intrinsic electronic characteristics, such as favorable charge transport or tunable redox potentials.14 Highly polarizable, electron-rich, polycyclic scaffolds with tailored electrochemical and photophysical properties were designed based on thiophene moieties.15,16 Thiophenes and in particular oligothiophenes are found amongst many other applications in materials science,17 in particular in OLEDs,18–20 OFETs,21,22 OPV,23,24 and DSSC.25–30 DSSC have been discussed to be a more sustainable alternative to conventional silicon-based solar cells, but for a fast amortization of the devices a low-cost fabrication is crucial. Furthermore, by establishing systematic structure–property relationships, which demands large substance libraries, the solar cell performances can be optimized and rationalized.25–30 Multicomponent reactions (MCR) are a valuable tool for the rapid, efficient and sustainable synthesis of structurally and functionally diverse substance libraries of DSSC dyes.31 Moreover, diverse other functional molecules are accessible by MCR.1 Syntheses of for instance quinoxalines, pyrazoles or indolones, functional heterocyclic π-systems, have been developed by MCR-approaches guaranteeing tailored photophysical properties.32–34 Also manifold de novo syntheses of thiophenes can be achieved by MCR.14,35 Surprisingly, noticeably fewer direct functionalizations of thiophenes in a one-pot fashion have been reported.31,36–38 The unsymmetrical one-pot functionalization of thiophenes turns out to be challenging but, nevertheless, important facing their applications.25–30,39 As a consequence, the careful design of MCR, opening a new access to functional thiophenes and especially donor–acceptor conjugates, becomes a demanding task. Suzuki coupling offers mild and versatile conditions for the (hetero)aryl functionalization of thiophenes,40,41 for instance with electron-donating groups, whereas formylation and Knoevenagel condensation reactions set the stage for a complementary acceptor substitution.3,31,42 Aiming for a MCR-approach, we assumed by the retrosynthetic analysis of thiophene donor–acceptor conjugates (Scheme 1), that in situ lithiation is the key step for concatenation of consecutive donor–acceptor functionalization of thiophenes in the sense of a one-pot Suzuki-Lithiation–Formylation-Knoevenagel (SLiForK) sequence.
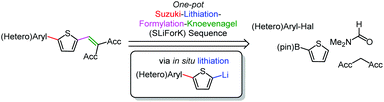 |
| Scheme 1 Consecutive four-step four-component synthesis of thiophene donor–acceptor conjugates by Suzuki-Lithiation–Formylation-Knoevenagel (SLiForK) sequence. | |
Here, we report first one-pot syntheses of unsymmetrically functionalized thiophenes, including novel DSSC dyes, by concatenation of Suzuki coupling and lithiation-electrophilic trapping reactions. The photophysical and electrochemical properties of chromophores and DSSC characteristics are assessed as well as the TDDFT calculated electronic structure.
Results and discussion
Synthesis
First, we set out to develop a Suzuki-Lithiation–Formylation-Knoevenagel (SLiForK) sequence as a rapid access to donor-thiophene–acceptor conjugates, which can be considered as a consecutive four-component process in a one-pot fashion (Table 1).
Table 1 Optimization of the four-component SLiForK synthesis of thiophene based donor–acceptor conjugates 1
Other than typical formylations under Lewis acidic conditions the formylation step was initiated by an in situ lithiation in non protic solvent systems. DMF is used in a twofold manner: As C1-building block in the formylation as well as precursor of dimethyl amine as previously reported for a formylation-Wittig-olefination sequence.43 Therefore, adding an organo catalyst for the Knoevenagel condensation was expendable. Due to the base sensitivity of activated Michael systems and ester functionalities, such as cyanoacrylate 1a, employing weakly nucleophilic bases for the Suzuki coupling step was crucial. In addition, we therefore identified cesium fluoride as a base, Pd(dba)2/HPtBu3BF4
44 as a catalyst and THF as the solvent of choice. Indeed, the coupling conditions were perfectly compatible with the subsequent lithiation step and the Knoevenagel condensation in the same reaction vessel. With the SLiForK sequence in hand five thiophene donor–acceptor conjugates 1 bearing various acceptor and donor substituents could be synthesized in moderate to very high yields up to 88% (Table 1).
The average single-step yields range from 57 to 96%, which further underlines the high bond forming efficiency of this one-pot sequence. The corresponding aldehyde 5-(4-methoxyphenyl)thiophene-2-carbaldehyde (see the ESI chapter 2†) preceding product 1a could be isolated in a yield of 45%, which is significantly lower than the yield of 1a (Table 1, entry 1) as a consequence of workup and chromatographic purification. This furthermore underlines the clear superiority of MCR over a sequence of one step transformations. Starting from Suzuki coupling of 2-thienyl dioxaborolane (2a) with the donor halide 3, the coupling product is lithiated with BuLi followed by formylation with DMF to finally react after buffering with acetic acid with methylene active compound 4, which are most commonly implemented as sensitizers for DSSC applications.25–29
Using thienyl dibromides 5 and phenyl dioxaborolane (2b) the order of reactants can be inversed and additional thiophene donor–acceptor conjugates 6 are obtained in high yields, expanding the SLiForK sequence to a S2LiForK (pseudo)five-component reaction (Table 2).
Table 2 Pseudo five-component S2LiForK synthesis of thiophene based donor–acceptor conjugates 6
The modular nature of the (pseudo)five-component S2LiForK synthesis furthermore allows the implementation of structure motives, that are found in sensitizers for highly efficient metal-free organic dye-sensitized solar cells (DSSC).3,42 Thereby substance libraries of novel promising DSSC dyes are readily accessible for structure–property relationships in a diversity-oriented one-pot fashion. For illustration three dyes 7a–7c were obtained in moderate overall yields with the S2LiForK sequence after an additional saponification step with lithium hydroxide in a THF–water mixture and purification (Scheme 2). The free cyano acrylic acid is necessary as an anchoring group for DSSC experiments (vide infra).
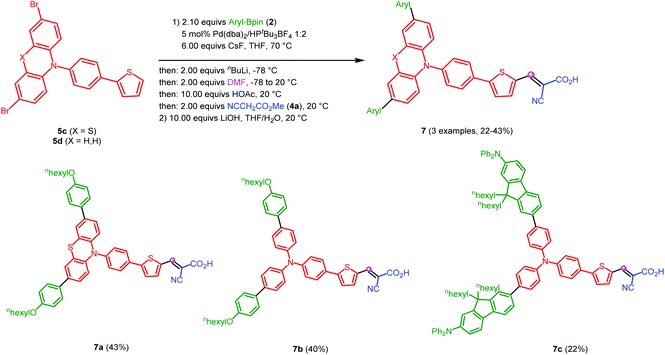 |
| Scheme 2 Synthesis of DSSC dyes 7a–7cvia (pseudo)five-component S2LiForK synthesis and saponification (yields after two steps and after purification by flash chromatography). | |
Finally, we reasoned that instead of formylation a borylation could follow directly leading into a terminating Suzuki coupling with the in situ generated boronate complex, i.e. a Suzuki-Lithiation-Borylation-Suzuki (SLiBS) sequence was envisioned. Starting from 2-thienyl dioxaborolane (2a) after Suzuki coupling with aryl halides 3 the obtained 2-arylated thiophene was lithiated with BuLi in the same reaction vessel and after borylation with trimethylborate the sequence was concluded by Suzuki coupling of different (hetero)aryl halides 3 to furnish unsymmetrically substituted diaryl thiophenes 8 in moderate to excellent yields (Table 3) in the sense of a consecutive three-component synthesis. In some cases, the yields could be optimized by a variation of the catalyst (Table 3, entry 2).
Table 3 Three-component SLiBS synthesis of various diaryl thiophenes 8
Aryl bromides and iodides 3 can be easily employed in the three-component SLiBS synthesis. In particular, electronically unsymmetrical substitution patterns of electron-deficient as well as electron-rich substituents can be introduced. Due to the overall robustness of donor aryl substituents against lithiation conditions, they are employed in the first Suzuki step whereas the second Suzuki coupling is perfectly suitable to implement acceptor substituents, such as cyano, nitro, and ester groups (Table 3, entries 2, 4–6, 11, and 12). Even thienyl cyanoacrylate 3k could be implemented with moderate yield (Table 3, entry 11), giving rise to access potential DSSC dyes. Hence, the SLiBS sequence is complementary to the SLiForK sequence and can be employed for the synthesis of π-expanded dyes. The required building-block 3k was synthesized via a consecutive three-component Lithiation–Formylation-Knoevenagel-condensation sequence (LiForK) with an excellent yield of 90% (Scheme 3), which is a shortened variant of the SLiForK sequence.
 |
| Scheme 3 Synthesis of acceptor substituted thiophene building-block 3kvia lithiation–formylation-Knoevenagel-condensation sequence (LiForK). | |
It is noteworthy to mention that some of the targeted compounds have been employed in medicinal chemistry (compounds 8a, 8b, 8e and 8h)45,46 and in materials science application (compound 8f),47 but have been synthesized in less efficient multistep approaches. Besides the overall advantage of one-pot methodology reducing the number of purification steps it must be emphasized that the novel SLiBS sequence is in addition highly catalyst economical. The initially employed catalyst source is still active in the concluding Suzuki step, i.e. no additional catalyst loading is required to achieve full conversion. With this the SLiBS sequence is another valuable example of sequentially Pd-catalyzed processes, where Pd-catalyzed steps can be intercepted by various organic transformations in one-pot processes.34
Electronic properties of dyes 7a–7c
The electronic properties of the dyes 7a–7c were assessed experimentally by cyclic voltammetry and absorption and emission spectroscopy (Table 4). First, the ground state properties of the dyes 7a–7c were examined by cyclovoltammetric measurements in dichloromethane solution, showing that all compounds possess two electrochemically reversible one-electron oxidations (for details see chapter 4 in the ESI†). The oxidation potential of phenothiazine derivative 7a is cathodically shifted by about 200 mV compared to the potentials of the triphenylamine derivatives 7b and 7c, in agreement with phenothiazines’ propensity to furnish highly stabilized radical cations with low oxidation potentials.48 The smaller cathodic shift of 7c (67 mV) cathodically compared to compound 7b rationalizes by the stronger codonor and more expanded π-system of compound 7c.
Table 4 Selected photophysical and electrochemical properties of dyes 7a–7c
Dye |
λ
abs (ε)a [nm] ([L mol−1 cm−1]) |
λ
em b [nm] |
E
0–0 c [eV] |
Δ d [cm−1] |
E
S/S+ evs. NHE (vs. Me10Fc) [V] |
E
S+/S* fvs. NHE [V] |
Recorded in CH2Cl2 at T = 298 K, c0(7) = 10−5 M.
Recorded in CH2Cl2 at T = 298 K, c0(7) = 10−6 M.
E
0–0 was determined from the cross section of absorption and emission spectra.
Δ = 1/λabs − 1/λem.
Recorded in 0.1 M [Bu4N][PF6], v = 100 mV s−1, Pt working, Ag/AgCl reference and Pt counter electrode, [Me10Fc]/[Me10Fc]+ as an internal standard,49ES/S+vs. NHE = E00/+1vs. Me10Fc + 0.209 V.
E
S+/S*
vs. NHE = ES+/Svs. NHE − E0–0.
Shoulder.
|
7a
|
465 (11 400)g |
626 |
2.31 |
5500 |
0.87 (0.66) |
−1.44 |
390 (32 600) |
284 (61 100) |
7b
|
499 (30 400) |
754 |
2.12 |
6800 |
1.07 (0.86) |
−1.05 |
338 (41 000) |
262 (30 400) |
7c
|
500 (29 100) |
708 |
2.16 |
5900 |
1.00 (0.79) |
−1.16 |
380 (89 600) |
312 (36 500) |
The photophysical properties of the dyes 7a–7c were examined by absorption and emission spectroscopy in a dichloromethane solution (Fig. 1). Phenothiazine dye 7a shows a bathochromically shifted absorption band in the UV/vis region at 465 nm with an extinction coefficient ε of 11
370 L mol−1 cm−1, whereas the triphenyl amine dyes 7b and 7c display broad stronger bathochromically shifted absorption bands at 500 nm with ε values of around 30
000 L mol−1 cm−1 (Fig. 1).
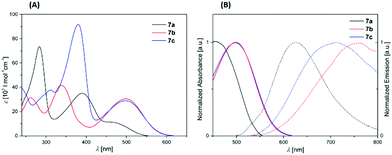 |
| Fig. 1 (A) UV/vis absorption spectra of the dyes 7 (c0(7) = 10−5 M, CH2Cl2, T = 298 K). (B) Normalized UV/vis absorption (solid lines, c0(7) = 10−6 M) and emission spectra (dashed lines, c0(7) = 10−6 M, λexc(7a) = 460 nm, λexc(7b, 7c) = 500 nm) of the dyes 7a–7c (recorded in CH2Cl2 at T = 298 K). | |
Additionally, all three dyes 7a–c possess two more major absorption bands at higher energies (Table 4). Since the three dyes 7a–c only differ in their donor unit, the observed absorption behavior can be directly correlated with the donor strength each. In this series phenothiazine acts as the weakest donor according to the hypsochromically shifted absorption band of 7a. All dyes 7a–7c fluoresce weakly (Fig. 1). Moreover, the emission of compounds 7b and 7c (λem = ∼754 nm) is very similar and more bathochromically shifted than the emission of compound 7a (λem = 626 nm). All Stokes shifts Δ
are relatively large and fall in a range from 5500 cm−1 (7a) to 6780 cm−1 (7b, 7c) indicating a significant change in the electronic structure upon photonic excitation from the electronic ground state to the vibrationally relaxed excited state (Table 4).
In combination with the optical band gap E0–0, derived from spectroscopic data, the excited state oxidation potentials ES+/S* can be estimated from the ground state oxidation potentials ES+/S (Table 4). A comparison of the ground and excited state oxidation potentials with the potentials of the conduction band (cb) of TiO2 (E0(cb TiO2) = −0.5 V vs. NHE) and an iodide/triiodide electrolyte (E0(I3−/I−) = 0.54 V vs. NHE)50 reveals that all dyes 7a–7c are well suited as sensitizers for DSSC devices based on a TiO2-photoanode and a iodide/triiodide electrolyte (Fig. 2). Both the electron transfer from the dyes’ excited states into the conduction band of TiO2 and the regeneration of the oxidized dyes by the electrolyte are thermodynamically allowed.
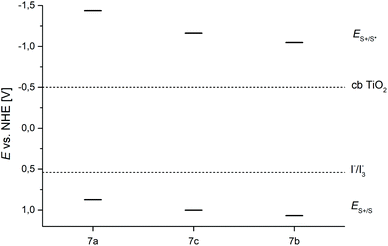 |
| Fig. 2 Schematic energy level diagram for the dyes 7a–7c. E0(cb TiO2) = −0.5 V vs. NHE; E0(I3−/I−) = 0.54 V vs. NHE. | |
Electronic structure of the dyes 7a–7c
The electronic structure of the dyes 7a–7c was examined via quantum chemical calculations on DFT level of theory for assigning the dominant longest wavelength absorption bands. The ground state geometries were optimized using the Gaussian09 program package,51 the PBEh1PBE functional52 and the 6-31G(d,p) basis set53 and the optimized geometries were confirmed as minima by analytical frequency analyses. Excitation energies were calculated with TDDFT54 methods as implemented in the Gaussian09 program package using the same functional and basis set. The polarizable continuum model (PCM) with dichloromethane as a solvent was additionally employed.55 The 6-31G(d,p) basis set was chosen to ensure reasonable computational cost. Likewise, hexyl groups were truncated to methyl groups in order to save computation time as alkyl side chains do not change the electronic structure of the π-system very much.
The computed excitation energies and oscillator strengths can be brought to coincidence with the experimental data satisfactorily, although the excitation energies are underestimated (Table 5). Other tested functionals, such as B3LYP51 or cam-B3LYP51 either deviated from with the experimental data or demanded considerable computational effort for calculating structure 7c (for details see chapter 5 in the ESI†). The longest wavelength absorption bands originate from dominant HOMO–LUMO based transitions, whereas the two strongly hyperchromically shifted absorption bands observed for 7a (λ = 284 nm, ε = 61
100 L mol−1 cm−1) and 7c (λ = 380 nm, ε = 89
640 L mol−1 cm−1) originate from phenothiazine and fluorene centered π–π* transitions, respectively.
Table 5 TDDFT calculations on the UV/vis-absorption spectra of the dyes 7a–7c (PBEh1PBE/6-31G(d,p), PCM CH2Cl2)
Dye |
λ
abs (ε)a [nm] ([L mol−1 cm−1]) |
λ
abs,calcd (oscillatory strength) [nm] |
Most dominant contributions |
Recorded in CH2Cl2, c = 10−5 M, T = 293 K.
|
7a
|
465 (11 400) |
494 (1.1231) |
HOMO → LUMO (98%) |
390 (32 600) |
379 (0.2233) |
HOMO−2 → LUMO (91%) |
284 (61 100) |
279 (0.9111) |
HOMO−1 → LUMO+1 (73%) |
7b
|
499 (30 400) |
558 (0.9228) |
HOMO → LUMO (99%) |
338 (41 000) |
390 (0.4298) |
HOMO−2 → LUMO (90%) |
262 (30 400) |
342 (0.7619) |
HOMO → LUMO+1 (97%) |
7c
|
500 (29 100) |
577 (0.7094) |
HOMO → LUMO (89%) |
380 (89 600) |
393 (1.9932) |
HOMO → LUMO+1 (79%) |
312 (36 500) |
379 (0.9619) |
HOMO−3 → LUMO (35%) |
The distribution of the coefficient densities in the Kohn–Sham frontier molecular orbitals (Fig. 3) accounts for a large charge-transfer character of the longest wavelength absorption bands. The HOMO coefficient densities are rather localized on the donor parts of the dyes 7a–7c, whereas the LUMO coefficient densities are dominant in the anchored acceptor units. For favorable and efficient generation of a photo current in a DSSC device a strong charge separation is demanded for obtaining high overall efficiency η.25–29
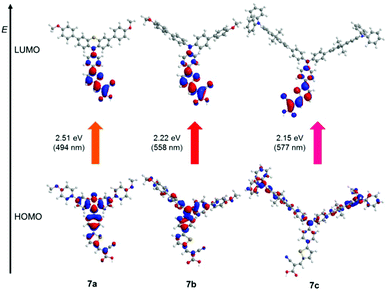 |
| Fig. 3 Selected DFT-computed (PBEh1PBE/6-31G(d,p), PCM CH2Cl2, isosurface value at 0.03 a.u.) Kohn–Sham FMOs of dyes 7a–c and representing contributions of the TDDFT-computed longest wavelength Franck–Condon absorption bands. | |
The large difference in Stokes shifts between phenothiazine 7a and triaryl amines 7b and 7c (Table 4) presumably originates from the different changes in geometry after excitation. This can be visualized consulting the ground state geometries (Fig. 3). Phenothiazines are known to planarize upon photoexcitation,56 which explains their inherently large Stokes shifts, but the triaryl amine moiety includes more degrees of freedom. Their twisted and less rigid ground state structure allows larger structural changes. The favored ground state geometry of phenothiazine 7a is the extra-conformation (Fig. 3), in which the phenothiazine is twisted in plane, compensating between steric hindrance and conjugation to the acceptor unit. This also rationalizes the weaker impact on the longest wavelength absorption band of the phenothiazine donor in 7a in comparison to triphenyl amine 7b, although the experimental oxidation potentials identify 7a as the dye bearing the most electron rich donor of the series (Table 4).
Solar cell performances of dyes 7a–7c
The solar cell performances of DSSC devices sensitized by the dyes 7a–7c were determined. The photoanodes consisted of nanocrystalline TiO2, the electrolyte was based on iodide/triiodide. For the preparation of the devices and the measurements we followed a previously published procedure.50 We referenced the measurements to the commercially available standard N3 (cis-bis(isothiocyanato)bis(2,2′-bipyridyl-4,4′-dicarboxylato)-ruthenium(II)), which was measured under the same conditions. We obtained reasonable fill factors FF within our DSSC measurements (Table 6). The overall efficiencies η of the DSSC devices sensitized with 7a (η = 2.87%, 0.70 of N3) and 7c (η = 3.15%, 0.77 of N3) are high referenced to N3 (η = 4.10%) and the overall efficiency of 7b (η = 3.86%, 0.94 of N3) is the highest in the series and almost reaches the overall efficiency of N3.
Table 6 Photovoltaic performance of DSSCs sensitized by the dyes 7a–7c
a
Dye |
j
sc [mA cm−2] |
V
OC [mV] |
FF |
η [%] |
η/ηN3 |
Measured under irradiation with AM 1.5 G simulated solar light (100 mW cm−2).
|
7a
|
8.18 |
747 |
0.47 |
2.87 |
0.70 |
7b
|
10.51 |
772 |
0.48 |
3.86 |
0.94 |
7c
|
9.45 |
753 |
0.44 |
3.15 |
0.77 |
N3
|
12.03 |
769 |
0.44 |
4.10 |
1.00 |
The incident photon-to-current conversion efficiency (IPCE) of DSSCs sensitized by the dyes 7b and 7c (Fig. 4) is in line with the absorption spectra recorded in dichloromethane solution. In the region of the longest wavelength absorption bands the IPCE of 7b is 42% (λ = 499 nm) and 38% (λ = 500 nm) for 7c. This slight decrease can be explained with the lower extinction coefficient of 7c (Table 4). Similarly, the maximum IPCE of 7c (80%, λ = 365 nm) exceeds the value of 7b (75%, λ = 350 nm) in the hypsochromic region due to the intense fluorene centered π–π* transitions for 7c. The IPCE curve of phenothiazine 7a is narrowed in comparison to dyes 7b and 7c as expected from the hypsochromically shifted longest wavelength absorption band. But the IPCE of 7a is higher than expected from the absorption spectra in solution (Fig. 1) and even exceeds the maximum IPCE of 7c in the region of the phenothiazine centered π–π* transitions with a maximum IPCE value of 90% (λ = 364 nm). This finding can be rationalized by the electrochemical properties of 7a, representing the largest driving force for the electron injection into the TiO2 conduction band (Table 4, Fig. 4), thereby compensating the weaker absorption. It is noteworthy that the IPCE-spectrum of 7a is shifted more bathochromically than the absorption spectra in dichloromethane solution (Fig. 1). This might indicate the formation of J-aggregates57 or broadened absorption bands50 on the TiO2 film.
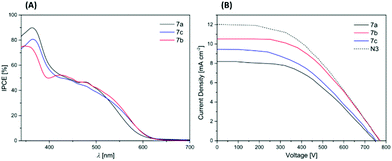 |
| Fig. 4 (A) Incident photon-to-current conversion efficiency (IPCE) of DSSCs sensitized by the dyes 7a–7c (iodine/triiodide electrolyte). (B) Photocurrent density vs. voltage curves of DSSCs sensitized by the dyes 7a–7c and N3 (iodine/triiodide electrolyte, under irradiation of AM 1.5 simulated sunlight, 100 mW cm−2). | |
A DSSC device based on 7b generated the top short circuit photocurrent density of jSC = 10.51 mA cm−2. The open circuit voltages VOC of the dyes 7a–7c (Fig. 4, Table 6) are in the order of N3. Compound 7b (VOC = 772 mV) even exceeds the value of N3. However, charge recombination reactions in the DSSC device can decrease the open circuit voltage. Bulky substituents shielding the photoanode from the electrolyte have been shown to reduce this recombination.42,57,58 Therefore, triaryl amine dyes 7b and 7c generate higher open circuit voltages in DSSC experiments than the phenothiazine 7a. Surprisingly, the open circuit voltage of 7c bearing bulky fluorene codonors is lower than open circuit voltage of 7b. The widely planar, conjugated π-system expanded by the fluorenes presumably favors the charge recombination as previously reported for similar systems.57,59,60
Experimental
Experimental details, full characterization and 1H and 13C NMR spectra of compounds 1, 3e, 3k and 5–8, DFT computed XYZ-coordinates, energies, and absorption spectra as well as cyclic voltammograms of compounds 7a–7c are compiled in the ESI.†
Typical procedure for the consecutive four-component synthesis of 1a via the SLiForK sequence
In a flame-dried Schlenk vessel with magnetic stir bar under nitrogen atmosphere were filled 4,4,5,5-tetramethyl-2-(thiophen-2-yl)-1,3,2-dioxaborolane (2a) (105 mg, 0.50 mmol), 4-iodoanisole (3a) (124 mg, 0.53 mmol), cesium fluoride (228 mg, 1.50 mmol), bis(dibenzylideneacetone)palladium(0) (14 mg, 25 μmol), and tri-tert-butyl phosphonium tetrafluoro-borate (14 mg, 50 μmol, 10 mol%) and dissolved in dry THF (8 mL). The reaction mixture was stirred at 70 °C for 1 h. Then, the reaction mixture was slowly cooled to ambient temperature and then to −78 °C (isopropanol/dry ice). To the cooled, viscous reaction mixture was added dropwise n-butyllithium (0.63 mL, 1.00 mmol, 1.6 M in hexane). Vigorous stirring was continued for 2 h at −78 °C. Then dry DMF (0.08 mL, 1.00 mmol) was added dropwise and stirring was continued for another 90 min at −78 °C. Then the reaction mixture was stirred for 30 min at ambient temperature, before acetic acid (0.29 mL, 5.00 mmol) was added, followed by stirring at ambient temperature for another 15 min. Then methyl cyanoacetate (4a) (0.09 mL, 1.00 mmol) was added and the reaction mixture was stirred at ambient temperature overnight (14 h). Then volatiles were removed by evaporation and the crude product was purified by flash chromatography on silica gel (n-hexane/ethyl acetate 5
:
1) to give 131 mg (0.47 mmol, 88%) of 1a as a yellow solid, Mp.: 145–150 °C. Rf (n-hexane/ethyl acetate 3
:
1) = 0.30. 1H NMR (300 MHz, acetone-d6): δ 3.78 (s, 3H), 3.88 (s, 3H), 7.03–7.10 (m, 2H), 7.59 (d, 3JHH = 4.06 Hz, 1H), 7.73–7.80 (m, 2H), 7.69 (dd, 3JHH = 4.08 Hz, 4JHH = 0.59 Hz, 1H), 8.43 (d, 4JHH = 0.52 Hz, 1H). 13C NMR (150 MHz, acetone-d6): δ 53.3 (CH3), 55.9 (CH3), 97.7 (Cquat), 115.7 (CH), 116.7 (Cquat), 124.6 (CH), 126.3 (Cquat), 128.8 (CH), 134.8 (Cquat), 142.0 (CH), 147.6 (CH), 155.4 (Cquat), 162.1 (Cquat), 163.9 (Cquat). MS(EI) m/z: 300 ([M + H]+, 18), 299 ([M]+, 100), 284 ([M − CH3]+, 32), 278 (12), 277 (29), 268 ([M − CH3O+], 10), 262 (18), 240 ([M − C2H3O2]+, 9), 196 (16), 192 ([M − C7H7O]+, 9), 183 (18), 149 (14). IR:
[cm−1] = 3038 (w), 3007 (w), 2953 (w), 2837 (w), 2216 (w), 1709 (s), 1607 (w), 1578 (s), 1557 (w), 1526 (w), 1495 (m), 1427 (s), 1393 (w), 1368 (w), 1337 (w), 1280 (m), 1252 (s), 1211 (s), 1204 (s), 1180 (s), 1117 (m), 1080 (m), 1063 (s), 1024 (s), 1009 (m), 955 (m), 930 (w), 799 (s), 758 (s), 719 (w), 692 (m), 662 (m), 629 (m). Anal. calcd for C16H13NO3S (299.3): C 64.20, H 4.38, N 4.68, S 10.71; found: C 64.23, H 4.51, N 4.68, S 10.41.
Typical procedure for the consecutive three-component synthesis of 8kvia the SLiBS sequence
In a flame-dried Schlenk vessel with magnetic stir bar under nitrogen atmosphere were filled 4,4,5,5-tetramethyl-2-(thiophen-2-yl)-1,3,2-dioxaborolane (2a) (94 mg, 0.45 mmol), 1-bromo-2,4-bis(hexyloxy)benzene (3e) (92 mg, 0.53 mmol), cesium fluoride (205 mg, 1.35 mmol), bis(dibenzylidene-acetone)palladium(0) (13 mg, 23 μmol) and tri-tert-butyl-phosphonium tetrafluoroborate (13 mg, 46 μmol) and dissolved in dry THF (8 mL). The reaction mixture was stirred at 70 °C for 2 h. Then, the reaction mixture was slowly cooled to ambient temperature and then to −78 °C (isopropanol/dry ice). To the cooled, viscous reaction mixture was added dropwise n-butyllithium (0.56 mL, 0.90 mmol, 1.6 M in hexane). Vigorous stirring was continued for 2 h at −78 °C. Then trimethyl borate (0.10 mL, 0.90 mmol) was added and the stirring was continued for 30 min at ambient temperature. Then methyl 4-iodobenzoate (3i) (142 mg, 0.54 mmol) and a second portion of cesium fluoride (205 mg, 1.35 mmol) were added. The reaction mixture was stirred for 20.5 h at 70 °C. The volatiles were removed by evaporation and the crude product was purified by flash chromatography on silica gel (n-hexane/ethyl acetate 20
:
1) to give 176 mg (0.36 mmol, 79%) of 8k as a light yellow solid, Mp.: 104 °C. Rf (n-hexane/ethyl acetate 20
:
1): 0.28. 1H NMR (300 MHz, CDCl3): δ 0.85–0.97 (m, 6H), 1.33–1.54 (m, 10H), 1.44–1.52 (m, 2H), 1.77–1.83 (m, 2H), 1.87–1.93 (m, 2H), 3.93 (s, 3H), 3.99 (t, 3JHH = 6.56 Hz, 3JHH = 6.56 Hz, 2H), 4.08 (t, 3JHH = 6.43 Hz, 3JHH = 6.43 Hz, 2H), 6.50–6.57 (m, 2H), 7.39 (q, 3JHH = 3.95 Hz, 2H), 7.55–7.60 (m, 1H), 7.65–7.71 (m, 2H), 8.00–8.06 (m, 2H). 13C NMR (125 MHz, CDCl3): δ 13.9 (CH3), 14.0 (CH3), 22.4 (CH2), 22.5 (CH2), 25.6 (CH2), 25.9 (CH2), 29.1 (CH2), 29.2 (CH2), 31.4 (CH2), 31.5 (CH2), 51.9 (CH3), 68.2 (CH2), 68.9 (CH2), 100.1 (CH), 105.8 (CH), 115.9 (Cquat), 124.2 (CH), 124.9 (CH), 125.0 (CH), 128.1 (Cquat), 128.7 (CH), 130.1 (CH), 139.2 (Cquat), 140.8 (Cquat), 141.2 (Cquat), 156.3 (Cquat), 159.9 (Cquat), 166.7 (Cquat). MS(EI) m/z (%): 495 ([M + H]+, 30), 494 ([M]+, 100), 410 ([M − C6H13]+, 16), 409 ([M − C6H13]+, 6), 327 ([M − C12H26]+, 12), 326 ([M − C12H26]+, 58), 325 ([M − C12H26]+, 11), 55 (10). IR:
[cm−1] = 2949 (w), 2926 (w), 2870 (w), 1712 (s), 1600 (m), 1573 (w), 1558 (w), 1537 (w), 1492 (w), 1467 (w), 1435 (m), 1303 (w), 1276 (s), 1255 (m), 1222 (w), 1182 (s), 1130 (m), 1107 (s), 1024 (m), 997 (w), 964 (w), 894 (w), 848 (w), 835 (m), 823 (w), 798 (m), 767 (s), 727. Anal. calcd for C30H38O4S (522.7): C 72.84, H 7.74, S 6.48; found: C 72.98, H 7.47, S 6.77.
Conclusions
A rapid, straightforward, divergent, and diversity-oriented access to functionalized thiophenes has been developed based upon one-pot Suzuki-Lithiation (SLi) sequences, namely Suzuki-Lithiation–Formylation-Knoevenagel (SLiForK) and Suzuki-Lithiation–Borylation-Suzuki (SLiBS) consecutive multicomponent reactions. Key step in this highly practical methodology is the concatenation of Suzuki coupling with in situ lithiation of aryl thiophenes. For electrophilic trapping DMF as C1-building block for the formylations and precursor of dimethyl amine, enables direct concatenation of a concluding Knoevenagel condensation in a one-pot fashion. Electrophilic borylation, in turn, directly furnishes the organometallic intermediate for a second Suzuki coupling in the sense of a sequentially Pd-catalyzed process. These thiophene donor–acceptor conjugates and unsymmetrically substituted diaryl thiophenes are of particular interest both due to their potential biological or electronic properties. Moreover, three DSSC dyes were prepared and their electronic properties and structure were investigated with experimental (absorption and emission spectroscopy, cyclovoltammetry) and theoretical methods (DFT and TDDFT calculations). DSSC devices sensitized with these dyes featured high overall efficiencies and even reached the value of the standard N3 under the similar conditions. The variation of codonor substituents, which were introduced by the one-pot synthesis, has a substantial impact on the solar cell performance of the dyes. Large substance libraries for further elucidating structure–property relationships of DSSC dyes can be easily realized. Studies directed to employing the highly modular one-pot synthesis for assessing various classes of OPV dyes are the subject of ongoing studies and are currently underway.
Conflicts of interest
There are no conflicts to declare.
Acknowledgements
The authors cordially thank the Fonds der Chemischen Industrie for financial support (scholarship of LM).
Notes and references
- L. Levi and T. J. J. Müller, Multicomponent syntheses of functional chromophores, Chem. Soc. Rev., 2016, 45, 2825–2846 RSC.
- M. Grätzel, Photoelectrochemical cells Nature, Nature, 2001, 414, 338–344 CrossRef PubMed.
- A. Mishra, M. K. Fischer and P. Bäuerle, Metal-free organic dyes for dye-sensitized solar cells: from structure: property relationships to design rules, Angew. Chem., Int. Ed., 2009, 48, 2474–2499 CrossRef CAS.
- Y.-W. Su, S.-C. Lan and K.-H. Wei, Organic photovoltaics, Mater. Today, 2012, 15, 554–562 CrossRef CAS.
- T. Ameri, N. Li and C. J. Brabec, Highly efficient organic tandem solar cells: a follow up review, Energy Environ. Sci., 2013, 6, 2390–2413 RSC.
-
V. W. W. Yam, M. M.-Y. Chan and C.-H. Tao, WOLEDs and Organic Photovoltaics, Springer-Verlag, Berlin, 2010 Search PubMed.
-
Organic Photovoltaics, ed. C. Brabec, V. Dyakonov and U. Scherf, Wiley-VCH, Weinheim, 2008 Search PubMed.
- L. Torsi, M. Magliulo, L. Manoli and G. Palazzo, Organic field-effect transistor sensors: a tutorial review, Chem. Soc. Rev., 2013, 42, 8612–8628 RSC.
- C.-H. Kim, Y. Bonnassieux and G. Horowitz, Compact DC Modeling of Organic Field-Effect Transistors: Review and Perspectives IEEE, Trans. Electron Devices, 2014, 61, 278–287 CAS.
-
I. Kymissis, Organic Field Effect Transistors Theory, Fabrication and Characterization, Springer, New York, 2009 Search PubMed.
-
Organic Light-Emitting Materials and Devices, ed. Z. R. Li, CRC Press, 2nd edn, 2015 Search PubMed.
- N. Thejo Kalyani and S. J. Dhoble, Organic light emitting diodes: Energy saving lighting technology-A review, Renewable Sustainable Energy Rev., 2012, 16, 2696–2723 CrossRef CAS.
-
Organic Light Emitting Devices: Synthesis, Properties and Applications, ed. K. Müllen and U. Scherf, Wiley VCH, Weinheim, 2006 Search PubMed.
- K. Schaper and T. J. J. Müller, Thiophene Syntheses by Ring Forming Multicomponent Reactions, Top. Curr. Chem., 2018, 376, 38 CrossRef PubMed.
- A. P. W. Schneeweis, S. T. Hauer, G. J. Reiss and T. J. J. Müller, Bis[1]benzothieno[1,4]thiazines: Planarity, Enhanced Redox Activity and Luminescence by Thieno-Expansion of Phenothiazine, Chem. – Eur. J., 2019, 25, 3582–3590 CrossRef CAS PubMed.
- C. Dostert, C. Wansrath, W. Frank and T. J. J. Müller, 4H-Dithieno[2,3-b:30,20-e][1,4]thiazines – synthesis and electronic properties of a novel class of electron rich redox system, Chem. Commun., 2012, 48, 7271–7273 RSC.
- G. Barbarella, M. Melucci and G. Sotgiu, The versatile thiophene: an overview of recent research on thiophene-based materials, Adv. Mater., 2005, 17, 1581–1593 CrossRef CAS.
- D. Fichou, Structural order in conjugated oligothiophenes and its implications on opto-electronic devices, J. Mater. Chem., 2000, 10, 571–588 RSC.
- C. M. Zhon, C. H. Duan, F. Huang, H. B. Wu and Y. Cao, Materials and devices toward fully solution processable organic light-emitting diodes, Chem. Mater., 2011, 23, 326–340 CrossRef.
- F. Geiger, M. Stoldt, H. Schweizer, P. Bäuerle and E. Umbach, Electroluminescence from oligothiophene- based light-emitting devices, Adv. Mater., 1993, 5, 922–925 CrossRef CAS.
- B. S. Ong, Y. L. Wu, P. Liu and S. Gardner, High-performance semiconducting polythiophenes for organic thin-film transistors, J. Am. Chem. Soc., 2004, 126, 3378–3379 CrossRef CAS PubMed.
- C. L. Wang, H. L. Dong, W. P. Hu, Y. Q. Liu and D. B. Zhu, Semiconducting pi-conjugated systems in field-effect transistors: a material odyssey of organic electronics, Chem. Rev., 2012, 112, 2208–2267 CrossRef CAS PubMed.
- K. Schulze, C. Uhrich, R. Schuppel, K. Leo, M. Pfeiffer, E. Brier, E. Reinold and P. Bäuerle, Efficient vacuum-deposited organic solar cells based on a new low-bandgap oligothiophene and fullerene C-60, Adv. Mater., 2006, 18, 2872–2875 CrossRef CAS.
- H. J. Son, F. He, B. Carsten and L. P. Yu, Are we there yet? Design of better conjugated polymers for polymer solar cells, J. Mater. Chem., 2011, 21, 18934–18945 RSC.
- A. Carella, F. Borbone and R. Centore, Research Progress on Photosensitizers for DSSC, Front. Chem., 2018, 11, 481 CrossRef PubMed.
- A. Hagfeldt, G. Boschloo, L. Sun, L. Kloo and H. Pettersson, Dye-Sensitized Solar Cells, Chem. Rev., 2010, 110, 6595–6663 CrossRef CAS PubMed.
- A. Shahzada, E. Guillén, L. Kavan, M. Grätzel and M. K. Nazeeruddin, Metal free sensitizer and catalyst for dye sensitized solar cells, Energy Environ. Sci., 2013, 6, 3439–3466 RSC.
- M. K. Nazeeruddin, E. Baranoff and M. Grätzel, Dye-sensitized solar cells: A brief overview, Sol. Energy, 2011, 85, 1172–1178 CrossRef CAS.
- B. O'Regan and M. Grätzel, A low-cost, high-efficiency solar cell based on dye-sensitized colloidal TiO2 films, Nature, 1991, 353, 737–740 CrossRef.
- P. P. Kumavat, P. Sonar and D. S. Dalal, An overview on basics of organic and dye sensitized solar cells, their mechanism and recent improvements, Renewable Sustainable Energy Rev., 2017, 78, 1262–1287 CrossRef.
- S. Fuse, S. Sugiyama, M. M. Maitani, Y. Wada, Y. Ogomi, S. Hayase, R. Katoh, T. Kaiho and T. Takahashi, Elucidating the Structure–Property Relationships of Donor–p- Acceptor Dyes for Dye-Sensitized Solar Cells (DSSCs) through Rapid Library Synthesis by a One-Pot Procedure, Chem. – Eur. J., 2014, 20, 10685–10694 CrossRef CAS PubMed.
- T. J. J. Müller, Multicomponent reactions in the synthesis of heterocycles (Editorial), Chem. Heterocycl. Compd., 2017, 53, 381 CrossRef.
- L. Levi and T. J. J. Müller, Multicomponent Syntheses of Fluorophores Initiated by Metal Catalysis, Eur. J. Org. Chem., 2016, 2907–2918 Search PubMed.
- T. Lessing and T. J. J. Müller, Sequentially Palladium-Catalyzed Processes in One-Pot Syntheses of Heterocycles, Appl. Sci., 2015, 5, 1803–1836 CrossRef CAS.
- O. Grotkopp and T. J. J. Müller, Synthesis of Bi- and Terthiophenes Initiated by Microwave-assisted Coupling-Isomerization Reaction, Chem. Heterocycl. Compd., 2017, 53, 66–71 CrossRef CAS; D. Urselmann, K. Deilhof, B. Mayer and T. J. J. Müller, Thiophene-Forming One-pot Synthesis of Three Thienyl-bridged Oligophenothiazines and Their Electronic Properties Beilstein, Beilstein J. Org. Chem., 2016, 12, 2055–2064 CrossRef PubMed.
- C. Muschelknautz, M. Sailer and T. J. J. Müller, Sequential Electrophilic Trapping Reactions for the Desymmetrization of Dilithio(hetero)arenes, Synlett, 2008, 845–848 CAS.
- K. M. Saini, R. K. Saunthwal and A. K. Verma, Pd-Catalyzed one-pot sequential cross-coupling reactions of tetrabromothiophene, Org. Biomol. Chem., 2017, 15, 10289–10298 RSC.
- C. Kieffer, V. Babin, M. Jouanne, I. Slimani, Y. Berhault, R. Legay, J. S. O. Santos, S. Rault and A. S. Voisin-Chiret, Sequential one pot double C-H heteroarylation of thiophene using bromopyridines to synthesize unsymmetrical 2,5-bipyridylthiophenes, Tetrahedron, 2017, 73, 5509–5516 CrossRef CAS.
- Y. Liu, A. Kumar, S. Depauw, R. Nhili, M.-H. David-Cordonnier, M. P. Lee, M. A. Ismail, A. A. Farahat, M. Say, S. Chackal-Catoen, A. Batista-Parra, S. Neidle, D. W. Boykin and W. D. Wilson, Water-Mediated Binding of Agents that Target the DNA Minor Groove, J. Am. Chem. Soc., 2011, 133, 10171–10183 CrossRef CAS PubMed.
- S. Kotha, K. Lahiri and D. Kashinath, Recent applications of the Suzuki–Miyaura cross-coupling reaction in organic synthesis, Tetrahedron, 2002, 58, 9633–9695 CrossRef CAS.
- B. Liégault, D. Lapointe, L. Caron, A. Vlassova and K. Fagnou, Establishment of Broadly Applicable Reaction Conditions for the Palladium-Catalyzed Direct Arylation of Heteroatom-Containing Aromatic Compounds, J. Org. Chem., 2009, 74, 1826–1834 CrossRef PubMed.
- D. P. Hagberg, J.-H. Yum, H. J. Lee, F. De Angelis, T. Marinado, K. M. Karlsson, R. Humphry-Baker, L. Sun, A. Hagfeldt, M. Grätzel and M. K. Nazeeruddin, Molecular Engineering of Organic Sensitizers for Dye-Sensitized Solar Cell Applications, J. Am. Chem. Soc., 2008, 130, 6259–6266 CrossRef CAS PubMed.
- For the twofold use of DMF in a formylation-Wittig olefination sequence, see: Q. Wang, H. Wie and M. Schlosser, The Simultaneous In-Situ Generation of Aldehydes and Phosphorus Ylides: A Convenient Multi-Step One-Pot Olefination Protocol, Eur. J. Org. Chem., 1999, 3263–3268 CrossRef CAS.
- A. F. Littke, C. Dai and G. C. Fu, Versatile Catalysts for the Suzuki Cross-Coupling of Arylboronic Acids with Aryl and Vinyl Halides and Triflates under Mild Conditions, J. Am. Chem. Soc., 2000, 122, 4020–4028 CrossRef CAS.
- R. Chandra, M. Kunga and H. F. Kung, Design, synthesis, and structure–activity relationship of novel thiophene derivatives for b-amyloid plaque imaging, Bioorg. Med. Chem. Lett., 2006, 16, 1350–1352 CrossRef CAS PubMed.
- M. A. Ismail, M. M. Youssef, R. K. Arafa, S. S. Al-Shihry and W. M. El-Sayed, Synthesis and antiproliferative activity of monocationic arylthiophene derivatives, Eur. J. Med. Chem., 2017, 126, 789–798 CrossRef CAS PubMed.
- G. Kirsch, D. Prim, F. Leising and G. Mignani, New thiophene derivatives as potential materials for non linear optics, Heterocycl. Chem., 1994, 31, 1005–1010 CrossRef CAS.
- D. Pan and D. L. Phillips, Raman and Density Functional Study of the S0 State of Phenothiazine and the Radical Cation of Phenothiazine, J. Phys. Chem. A, 1999, 103, 4737–4743 CrossRef CAS.
- I. Noviandri, K. N. Brown, D. S. Fleming, P. T. Gulyas, P. A. Lay, A. F. Masters and L. Phillips, The Decamethylferrocenium/Decamethylferrocene Redox Couple: A Superior Redox Standard to the Ferrocenium/Ferrocene Redox Couple for Studying Solvent Effects on the Thermodynamics of Electron Transfer, J. Phys. Chem. B, 1999, 103, 6713–6722 CrossRef CAS.
- T. Meyer, D. Ogermann, A. Pankrath, K. Kleinermanns and T. J. J. Müller, Phenothiazinyl Rhodanylidene Merocyanines for Dye-Sensitized Solar Cells, J. Org. Chem., 2012, 77, 3704–3715 CrossRef CAS PubMed.
-
M. J. Frisch, G. W. Trucks, H. B. Schlegel, G. E. Scuseria, M. A. Robb, J. R. Cheeseman, G. Scalmani, V. Barone, B. Mennucci, G. A. Petersson, H. Nakatsuji, M. Caricato, X. Li, H. P. Hratchian, A. F. Izmaylov, J. Bloino, G. Zheng, J. L. Sonnenberg, M. Hada, M. Ehara, K. Toyota, R. Fukuda, J. Hasegawa, M. Ishida, T. Nakajima, Y. Honda, O. Kitao, H. Nakai, T. Vreven, J. A. Montgomery Jr., J. E. Peralta, F. Ogliaro, M. Bearpark, J. J. Heyd, E. Brothers, K. N. Kudin, V. N. Staroverov, R. Kobayashi, J. Normand, K. Raghavachari, A. Rendell, J. C. Burant, S. S. Iyengar, J. Tomasi, M. Cossi, N. Rega, J. M. Millam, M. Klene, J. E. Knox, J. B. Cross, V. Bakken, C. Adamo, J. Jaramillo, R. Gomperts, R. E. Stratmann, O. Yazyev, A. J. Austin, R. Cammi, C. Pomelli, J. W. Ochterski, R. L. Martin, K. Morokuma, V. G. Zakrzewski, G. A. Voth, P. Salvador, J. J. Dannenberg, S. Dapprich, A. D. Daniels, O. Farkas, J. B. Foresman, J. V. Ortiz, J. Cioslowski and D. J. Fox, Gaussian 09, Revision A.02, Gaussian, Inc., Wallingford CT, 2009 Search PubMed.
- C. Lee, W. Yang and R. G. Parr, Development of the Colle-Salvetti correlation-energy formula into a functional of the electron density, Phys. Rev. B: Condens. Matter Mater. Phys., 1988, 37, 785–789 CrossRef CAS PubMed; A. D. Becke, A new mixing of Hartree–Fock and local density-functional theories, J. Chem. Phys., 1993, 98, 1372–1377 CrossRef; A. D. Becke, Density-functional thermochemistry. III. The role of exact exchange, J. Chem. Phys., 1993, 98, 5648–5652 CrossRef; K. Kim and K. D. Jordan, Comparison of Density Functional and MP2 Calculations on the Water Monomer and Dimer, J. Phys. Chem., 1994, 98, 10089–10094 CrossRef; P. J. Stephens, F. J. Devlin, C. F. Chabalowski and M. J. Frisch, Ab Initio Calculation of Vibrational Absorption and Circular Dichroism Spectra Using Density Functional Force Fields, J. Phys. Chem., 1994, 98, 11623–11627 CrossRef; T. Yanai, D. Tew and N. Handy, A new hybrid exchange-correlation functional using the Coulomb-attenuating method (CAM-B3LYP), Chem. Phys. Lett., 2004, 393, 51–57 CrossRef.
- W. J. Hehre, R. Ditchfeld and J. A. Pople, Self-Consistent Molecular Orbital Methods. XII. Further Extensions of Gaussian-Type Basis Sets for Use in Molecular Orbital Studies of Organic Molecules, J. Chem. Phys., 1972, 56, 2257 CrossRef CAS.
- R. Bauernschmitt and R. Ahlrichs, Treatment of electronic excitations within the adiabatic approximation of time dependent density functional theory, Chem. Phys. Lett., 1996, 256, 454–464 CrossRef CAS; M. E. Casida, C. Jamorski, K. C. Casida and D. R. Salahub, Molecular excitation energies to high-lying bound states from time-dependent density-functional response theory: Characterization and correction of the time-dependent local density approximation ionization threshold, J. Chem. Phys., 1998, 108, 4439–4449 CrossRef; R. E. Stratmann, G. E. Scuseria and M. J. Frisch, An efficient implementation of time-dependent density-functional theory for the calculation of excitation energies of large molecules, J. Chem. Phys., 1998, 109, 8218–8224 CrossRef.
- G. Scalmani and M. J. Frisch, Continuous surface charge polarizable continuum models of solvation. I. General formalism, J. Chem. Phys., 2010, 132, 114110–114115 CrossRef PubMed.
- L. Yang, J.-K. Feng and A.-M. Ren, Theoretical Study on Electronic Structure and Optical Properties of Phenothiazine-Containing Conjugated Oligomers and Polymers, J. Org. Chem., 2005, 70, 5987–5996 CrossRef CAS PubMed.
- H. Zhang, J. Fan, Z. Iqbal, D.-B. Kuang, L. Wang, D. Cao and H. Meier, Anti-recombination organic dyes containing dendritic triphenylamine moieties for high open-circuit voltage of DSSCs, Dyes Pigm., 2013, 99, 74–81 CrossRef CAS.
- B. C. O'Regan and J. R. Durrant, Kinetic and energetic paradigms for dye-sensitized solar cells: moving from the ideal to the real, Acc. Chem. Res., 2009, 42, 1799–1808 CrossRef PubMed.
- Z.-S. Wang, N. Koumura, Y. Cui, M. Takahashi, H. Sekiguchi, A. Mori, T. Kubo, A. Furube and K. Hara, Hexylthiophene- functionalized carbazole dyes for efficient molecular photovoltaics: tuning of solar-cell performance by structural modification, Chem. Mater., 2008, 20, 3993–4003 CrossRef CAS.
- K. M. Karlsson, X. Jiang, S. K. Eriksson, E. Gabrielsson, H. Rensmo, A. Hagfeldt and L. Sun, Phenoxazine dyes for dye-sensitized solar cells: relationship between molecular structure and electron lifetime, Chem. – Eur. J., 2011, 17, 6415–6424 CrossRef CAS PubMed.
Footnote |
† Electronic supplementary information (ESI) available. See DOI: 10.1039/c9qo01318k |
|
This journal is © the Partner Organisations 2020 |