DOI:
10.1039/D0QM00469C
(Research Article)
Mater. Chem. Front., 2020,
4, 3074-3085
Nanosilver-enhanced AIE photosensitizer for simultaneous bioimaging and photodynamic therapy†
Received
9th July 2020
, Accepted 10th August 2020
First published on 10th August 2020
Abstract
Theranostic photosensitizers which enable both disease diagnosis and effective treatment have recently received much attention towards personalized medicine. Herein, we report a multifunctional nanohybrid system using silver nanoparticles (AgNPs) to enhance the singlet oxygen generation (SOG) and fluorescence properties of a unique photosensitizer with aggregation-induced emission (AIE-PS) for simultaneous bioimaging and photodynamic therapy. To study the metal-enhancement effects, 4-mercaptobenzoic acid-capped AgNPs with well-controlled size (14, 40, and 80 nm) were synthesized to form nanohybrids with a specially designed red-emissive AIE-PS via simple electrostatic interactions. The careful control of the Ag nanoparticle concentration and the unique design of 80 nm AgNP@red-emissive AIE in this study resulted in a 10-fold enhancement in SOG, which is higher than other reported ME-SOG systems using similar plasmonic enhancers. Furthermore, the as-developed AgNP@AIE-PS nanohybrid exhibited improved photostability with negligible fluorescence quenching (5%), which is important for cell tracking. In addition, cytotoxity tests showed that these nanohybrids are biocompatibile with normal NIH-3T3 cells under dark conditions. Thus, they were employed for simultaneous imaging and photodynamic ablation of HeLa cancer cells. The results show that this brightly fluorescent AgNP@AIE-PS enabled about 4 times higher efficacy in PDT as compared to the control sample (i.e., 85% vs. 20% cell death) under low intensity white light irradiation (40 mW cm−2) for only 10 minutes, demonstrating its promising potential for advanced theranostic treatment in future nanomedicine.
1. Introduction
Developing multifunctional hybrid nanomaterials for simultaneous diagnosis and effective cancer treatment via fluorescence imaging and photodynamic therapy has received much interest in recent years.1–7 Photodynamic therapy (PDT) is an emerging non-invasive technique based on the use of light, oxygen, and photosensitizing molecules to produce reactive oxygen species (ROS) for killing cancer and/or bacterial cells.8–12 In particular, efficient PDT relies on the generation of sufficient ROS, especially singlet oxygen (1O2), to be able to disrupt the ‘stubborn’ microbial and cancerous cells.13–15 Although some of the conventional photosensitizers are also fluorescent molecules, most of them are not soluble in water, making them easily aggregate at high concentrations in the biological medium due to π–π stacking.16 This often leads to reduced singlet oxygen generation abilities and also reduced fluorescence due to the self-quenching effect.3,17–19 Therefore, there is a pressing need to develop new generation PDT agents that could overcome the drawbacks of traditional photosensitizers for effective theranostic treatment.
Nowadays, aggregation-induced emission photosensitizers (AIE-PSs) have received much attention in the biomedicine communities owing to their intrinsic fluorescence which could be “turned on” in the aggregate state for disease monitoring while generating singlet oxygen species for PDT treatment.17,20–22 For biological applications,23 water-soluble AIE molecules or AIE-PSs can be easily designed by introducing charged moieties onto the AIE-cores, such as tetraphenylethene (TPE) and tetraphenylsilole (TPS). As such, the aggregation-induced properties of AIE-PSs can also be enhanced by interacting with oppositely charged molecules or metal nanoparticles to form an ‘enhanced’ hybrid system for effective theranostic treatment. Furthermore, as most AIE-PSs have greater photostability and resistance to chemical degradation as compared to the conventional fluorophores or photosensitizers, they are more suitable for use in long term cancer cell tracking and treatment when they are irradiated under white light for an extended period of time. All these unique features of AIE-PSs make them a promising candidate for use in next generation PDT.24–27
Other than exploring new designs of photosensitizers, a plasmonic enhancement strategy has been exploited to improve their singlet oxygen generation efficiency,28,29 termed metal-enhanced singlet oxygen generation (ME-SOG). Similar to the theory of metal-enhanced fluorescence (MEF)30–32 and surface-enhanced Raman scattering,33–35 there are many factors affecting ME-SOG, including the size36,37 and shape34,38 of the metal nanoparticles (NPs) as well as the distance between the photosensitizer (PS) and the metal NPs.37–39 Typically, the photosensitizer can be either directly in contact with the metal surface29,36,40–42 or separated by a dielectric spacer38,39 to balance the competition between the enhanced excitation rate and non-radiative energy transfer from the excited PS* molecule to the metal surface. However, to the best of our knowledge, metal-enhanced singlet oxygen generation of AIE-PSs has not been systematically studied or designed for theranostic applications.
It is well known that photosensitization and fluorescence are competing processes for any photosensitizer, which makes it very difficult to enhance the photosensitization without affecting the fluorescence. Herein, we report a multifunctional silver nanoparticle-AIE photosensitizer (AgNP@AIE-PS) hybrid system to enhance the singlet oxygen generation efficiency of the AIE-PS without affecting its fluorescence brightness via systematic plasmonic engineering. Specifically, negatively charged 4-mercaptobenzoic acid-capped AgNPs and positively charged red-emissive AIE-PSs were used to form AgNP@AIE-PS through a simple electrostatic-induced aggregation approach. The SOG efficiency of the hybrid system was examined by a chemical trapping method while the photophysical properties were characterized using various techniques such as UV-visible (UV-vis) and photoluminescence spectroscopy, transmission electron microscopy (TEM) and time-resolved fluorescence spectroscopy. The plasmonic enhancement effects in SOG were assessed by comparing it with a control sample made from AIE-PS loaded silica nanoparticles (SNP@AIE-PS). The results show that a maximum 10-fold enhancement in singlet oxygen generation with minimum fluorescence quenching (∼5%) could be achieved for the 80 nm AgNP@AIE-PS. It was also found that the as-developed 80 nm AgNP@AIE-PS possesses high photostability in the hybrid system with good biocompatibility, and it has been applied successfully for simultaneous bioimaging and photodynamic therapy in cancer cell ablation as demonstrated in this study.
2. Results and discussion
2.1. Metal-enhanced singlet oxygen generation of the AIE-PS
The theranostic PS used in this study was synthesized from an AIE molecule with a tetraphenylethylene backbone modified with vinyl pyridinium43 (Fig. 1a). The as-synthesized AIE-PS is red-emissive when aggregated in a weak solvent, e.g., phosphate buffer solution. It shows a maximum absorption peak at 400 nm from the UV-vis absorption spectrum and an emission peak at 625 nm (Fig. 1b). The fluorescence quantum yield of the aggregated AIE-PS was measured to be 1.8% using 4-(dicyanomethylene)-2-methyl-6-(p-dimethylaminostyryl)-4H-pyran (DCM) as a reference.43 The singlet oxygen (1O2) generation property of the red-emissive AIE-PS was studied by a chemical trapping method using 9,10-anthracenediyl-bis(methylene)dimalonic acid (ABDA) as the probe, where a decrease in the absorption peak of ABDA corresponds to the amount of 1O2 reacted. As shown in Fig. 1c, the as-synthesized AIE-PS also possesses good 1O2 generation as evidenced by the rapid decrease of the ABDA characteristic peaks (i.e., about 65% of ABDA was degraded in 20 min upon white light irradiation). The 1O2 quantum yield of the red-emissive AIE-PS was estimated to be 78.5% as compared to the conventional photosensitizer Rose Bengal (75%) using ABDA as the 1O2 trapping agent (Fig. S1, ESI†). The good photosensitization ability of the AIE-PS is due to the introduction of electron donor and electron acceptor groups onto the TPE molecule, which decreases the singlet–triplet energy (ΔST), leading to an increase in the intersystem crossing (ISC) rate and better singlet oxygen generation efficiency relative to that of TPE.44
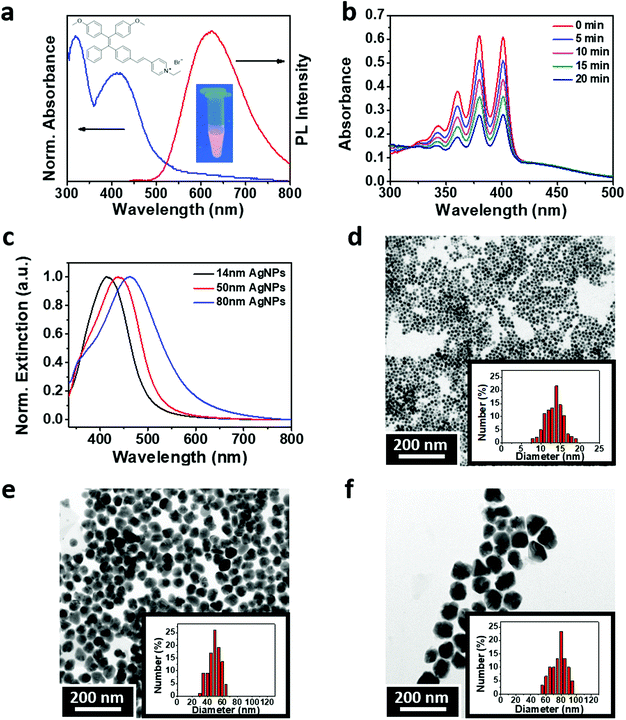 |
| Fig. 1 (a) UV-vis (blue line, left axis) and fluorescence spectra (red line, right axis) of the AIE-PS. The insets show the molecular structure and fluorescence image of the AIE-PS molecule in PBS buffer under UV light. (b) Time profile showing the absorption spectra of ABDA (50 μM) in the presence of the AIE-PS (9 μM) under 40 mW cm−2 white light irradiation. (c) Normalized extinction spectra, and TEM images of 4-MBA-capped AgNPs with three distinct sizes: (d) 14 nm AgNPs (14 ± 2.7 nm), (e) 50 nm AgNPs (50 ± 8.9 nm), and (f) 80 nm AgNPs (80 ± 12.7 nm). The insets show the size distribution of the obtained AgNPs. | |
For the study of metal-enhanced singlet oxygen generation, three distinct sizes of silver nanoparticles (AgNPs) capped with 4-mercaptobenzoic acid were synthesized (Fig. 1c). The size and zeta potential of the as-prepared 4-MBA-capped AgNPs were measured using transmission electron microscopy (TEM) and a Zetasizer, respectively. As shown in Fig. 1d–f, three samples of the 4-MBA-capped AgNPs showed a uniform distribution of particle sizes with a mean diameter of 14 ± 3 (14 nm AgNPs), 40 ± 9 nm (40 nm AgNPs) and 80 ± 13 nm (80 nm AgNPs), respectively. These negatively charged AgNPs (see the respective zeta potential values in Fig. S2, ESI†) were then mixed with the positively charged AIE-PS in aqueous solution (pH = 4) to prepare the AgNP@AIE-PS hybrid systems via electrostatic attraction forces (see the Experimental section for details).
The singlet oxygen generation rate of AgNP@AIE-PS could be monitored using ABDA as the 1O2 trapping probe, and was determined by the first-order kinetic constant (KABDA) of ABDA's degradation rate. That is, the larger KABDA, the faster the 1O2 generation of the sample. Fig. 2a shows the 1O2 generation of AgNP@AIE-PS prepared with the AgNPs of different sizes (i.e., 14 nm, 50 nm, and 80 nm) at varied concentrations. It was observed that the 1O2 generation rate of AgNP@AIE-PS increases as more AgNPs were introduced until it reaches a maximum value at its optimum concentration, beyond which the 1O2 generation rate decreases. The optimum concentrations of AgNPs to achieve maximum 1O2 generation as indicated by the first-order kinetic constant were 3 pM for 14 nm AgNPs (KABDA = 0.0682 min−1), 2 pM for 50 nm AgNPs (KABDA = 0.0721 min−1) and 0.22 pM for 80 nm AgNPs (KABDA = 0.1831 min−1). Interestingly, the addition of much fewer 80 nm AgNPs led to the highest 1O2 generation among the tested AgNPs of different sizes. This could be attributed to the relationship between the plasmonic effect of the Ag NPs and their surface coverage by the AIE-PS molecules. Hence, the maximum 1O2 generation rate is expected to be observed for the samples with maximum surface coverage, where a further increase in the concentration of AgNPs could lead to a lesser number of AIE-PS molecules deposited on the surface of the AgNPs. To examine this hypothesis, the amounts of adsorbed AIE-PS molecules on the three samples of AgNP@AIE-PS with maximum 1O2 generation rate at their optimum concentration (i.e., 3, 2, and 0.22 pM for 14 nm AgNPs, 50 nm AgNPs, and 80 nm AgNPs, respectively) were determined experimentally. Specifically, AgNP@AIE-PS (before addition of PBS) was centrifuged to obtain the supernatant, where its absorbance intensity was measured and compared with that in the initial AIE-PS solution (9 μM).
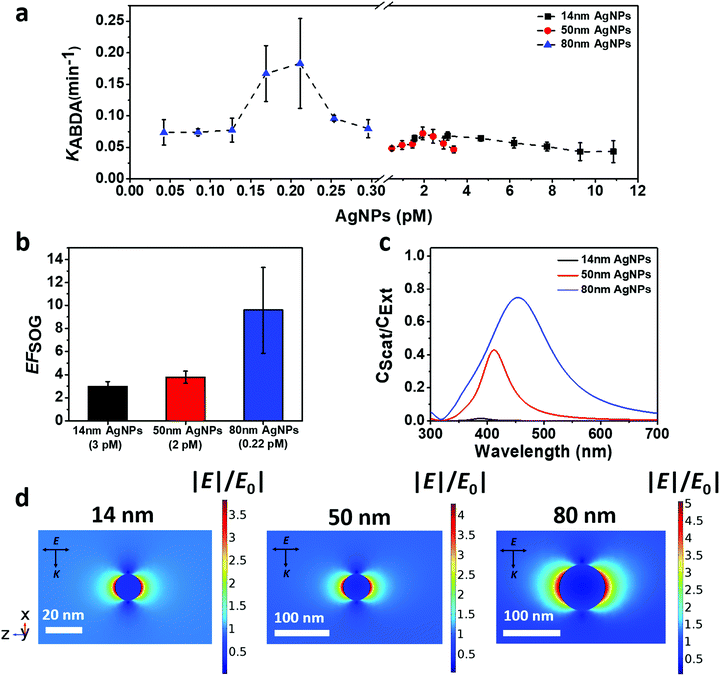 |
| Fig. 2 (a) Plot of the ABDA kinetic constant (KABDA) vs. different concentrations of AgNPs showing the plasmonic enhancement effect of 14 nm AgNPs (squares), 50 nm AgNPs (spheres), and 80 nm AgNPs (triangles) on the singlet oxygen generation efficiency of the AIE-PS; (b) calculated SOG enhancement factor (EFSOG) for AgNP@AIE-PS at the respective optimum concentration of AgNPs; and simulated (c) scattering contribution (CScat/CExt) and (d) electric field distribution of AgNPs in water, where CScat and CExt are the scattering and extinction efficiency of the AgNPs, respectively. The arrows for E and K indicate the direction of electric field polarization and incident light propagation, respectively. | |
For the control sample, the same amount of AIE-PS molecules was loaded onto negatively-charged silica nanoparticles (SNP) with a diameter of 104 ± 3 nm and zeta potential of −29.6 ± 1 mV.33 As such, AIE-PS molecules on the surface of SNP would adsorb in the same aggregation state as that on the surface of AgNPs. Hence, the SOG enhancement factor (EFSOG) of AgNP@AIE-PS can be calculated based on the first-order kinetic constant of ABDA degradation in the presence of the metal-enhanced sample (KAgNP@AIE–PS) and control sample (KSNP@AIE–PS), respectively, using the following equation:
| 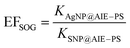 | (1) |
Fig. 2b shows the SOG enhancement factor as a function of AgNP size in the order of 14 nm AgNPs < 50 nm AgNPs < 80 nm AgNPs, where a maximum ∼10-fold enhancement was observed for the 80 nm AgNP@AIE-PS sample. This trend is consistent with the size-dependent light scattering contribution (
CScat/
CExt) of AgNPs in
Fig. 2c, which was obtained by the mathematical simulations of absorbance, scattering and extinction spectra of individual AgNPs (Fig. S3, ESI
†). The size-dependency of ME-SOG as observed is due to the plasmonic coupling between the AgNPs and excited AIE-PS molecules where larger metal nanoparticles are known to exhibit stronger field enhancement than that of the smaller ones.
36,45 The simulation results in
Fig. 2d further show that the electric field surrounding the surface of the 80 nm AgNPs is stronger as compared with the respective 50 nm AgNPs and 14 nm AgNPs under a plane-wave light source with a wavelength of 625 nm (
i.e., the maximum emission wavelength of the AIE-PS).
2.2. Plasmonic effects on the photophysical properties of AgNP@AIE-PS
To assess the plasmonic effect of the AgNPs on the fluorescence properties of the red-emissive AIE-PS, the photoluminescent intensity (PL) of the AgNP@AIE-PS samples was measured (Fig. S4, ESI†) and used to calculate the fluorescence quantum yield (see the ESI,† SI-S3, for detailed calculations). It was found that the presence of AgNPs quenched the fluorescence of the AIE-PS in the hybrid system. Fig. 3a shows the change in the fluorescence quantum yield of AgNP@AIE-PS with different AgNP concentrations. Unlike other particle sizes across different concentrations, there is only a slight change in the fluorescence quantum yield of the AIE-PS when it was adsorbed onto the surface of 80 nm AgNPs (<10% for all tested concentrations). To further evaluate the fluorescence quenching mechanism of AgNP@AIE-PS, the spectral overlap (J(λ)) between the extinction of AgNPs (as the acceptor) and emission of the AIE-PS (as the donor), as shown in Fig. 3b, was quantified using the following equation: | 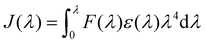 | (2) |
where F(λ) is the normalized fluorescence spectrum of the AIE-PS and ε(λ) is the molar extinction coefficient of the AgNPs in mol−1 dm3 cm−1 at a certain wavelength (λ). The calculated values of J(λ) for the different sizes of AgNPs are 2.96 × 10−13 for the 14 nm AgNPs, 4.63 × 10−13 for the 50 nm AgNPs and 2.29 × 10−12 for the 80 nm AgNPs, which are considerably less than those obtained for the reported fluorescence energy transfer (FRET) pairs.46,47 Thus, non-radiative energy transfer from the AIE-PS to AgNPs via other mechanisms than FRET is expected (Fig. 3c). Despite the fact that few mechanisms48,49 have been proposed to explain the energy transfer between a donor and acceptor pair, nanometal surface energy transfer (NSET) is the one providing a better correlation between the theory and experimental fluorescence quenching results when the fluorescence of a fluorophore is quenched in the vicinity of a metallic surface with insufficient spectral overlap.50 As can be seen in Fig. 3d, NSET is the main responsible mechanism for the fluorescence quenching as observed in AgNP@AIE-PS (see the ESI,† SI-5, for detailed calculations).
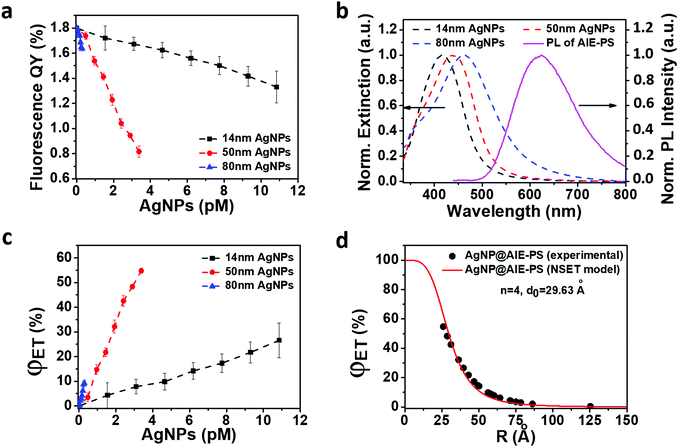 |
| Fig. 3 (a) Change (%) in the fluorescence quantum yield (QY) of different AgNP@AIE-PS samples, (b) spectral overlap between the extinction of AgNPs (dashed-dot lines, left axis) and emission of the AIE-PS (solid line, right axis), (c) energy transfer efficiency (ϕET %) from the AIE-PS to the AgNPs as a function of the size and concentration of the AgNPs, and (d) fitting of experimental energy transfer data (black circles) with the nanometal surface energy transfer (NSET) model (red line) of AgNP@AIE-PS. | |
Next, the photophysical properties of the red-emissive AgNP@AIE-PS hybrids with the greatest SOG enhancement factor at each particle size were investigated (Fig. 2a). Fig. 4a shows that the fluorescence lifetime of AIE-PS molecules in the hybrid system decreases when they were attached to the surface of AgNPs. It is noticed that the presence of larger AgNPs (e.g., 80 vs. 14 nm) would lead to a shorter fluorescence lifetime (i.e., 4.25 versus 4.64 ns) as observed in the AgNP@AIE-PS studied herein. However, there is no direct correlation between the energy transfer efficiency of the hybrids (i.e., 9% for 14 nm AgNP@AIE-PS, 32% for 50 nm AgNP@AIE-PS, and 5% for 80 nm AgNP@AIE-PS) and their fluorescence lifetime. This could be explained by the modification of both radiative and non-radiative decay rates in the vicinity of AgNPs due to the enhanced electric field around them.32,51 As shown in Fig. 4b, the net absorbance spectra of the AIE-PS have been increased in the presence of AgNPs of increasing sizes. This provides strong evidence to support the observation that the 80 nm AgNP@AIE-PS exhibited the highest SOG enhancement factor among other nanohybrids with smaller particle sizes. In addition, the presence of plasmonic NPs also enhanced the photostability of 80 nm AgNP@AIE-PS as compared with the AIE-PS alone in PBS (Fig. 4c), showing its great promise as a theranostic agent for long-time PDT and cell tracking.
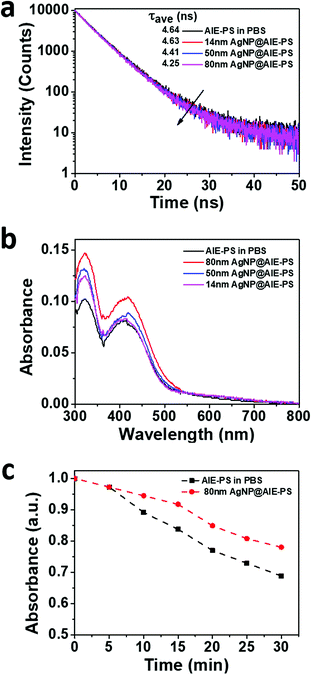 |
| Fig. 4 (a) Fluorescence decay profile of the respective AgNP@AIE-PS hybrids with maximum ME-SOG. (b) Change in the absorbance spectra of the AIE-PS in PBS after forming a hybrid with AgNPs of different sizes. The absorbance of the AgNPs has been subtracted from the spectra. (c) Comparison of the photostability between 80 nm AgNP@AIE-PS and the AIE-PS alone in PBS buffer. Both samples were irradiated with 100 mW cm−2 white light. All the samples were prepared at a concentration of 3 pM 14 nm AgNPs, 2 pM 50 nm AgNPs, 0.22 pM 80 nm AgNPs, and 9 μM of AIE-PS molecules, respectively. The excitation and emission wavelengths for the fluorescence measurements are λex = 374 nm and λem = 625 nm, respectively. | |
As depicted in the simplified Jablonski diagram in Scheme 1, the excited electrons come back to the ground state via radiative (fluorescence) or non-radiative decay, while some of them can go to the triplet state via the intersystem crossing (ISC) process. The electrons in the triplet state can produce singlet oxygen molecules (1O2) via energy transfer to the surrounding triplet molecular oxygen (3O2). In the presence of AgNPs (Scheme 1b), their strong light scattering provides more photons to be absorbed by the AIE-PS. Therefore, the excitation rate in the AIE-PS is increased due to the enhanced photon absorption as well as the enhanced electric field around the AgNPs. The higher rate for excitation of electrons in the singlet state leads to an increase in the ISC rate. This would result in a larger number of electrons present in the triplet state, causing more singlet oxygen to be generated. However, there is substantial energy transfer from the excited AIE-PS* molecules to the surface of AgNPs via the NSET mechanism, which neutralizes the effect of enhanced excitation by the AgNPs. This explains why there is only slight fluorescence quenching (5% for 0.22 pM 80 nm AgNPs) observed in the 80 nm AgNP@AIE-PS with the highest SOG enhancement effect in the nanohybrid system.
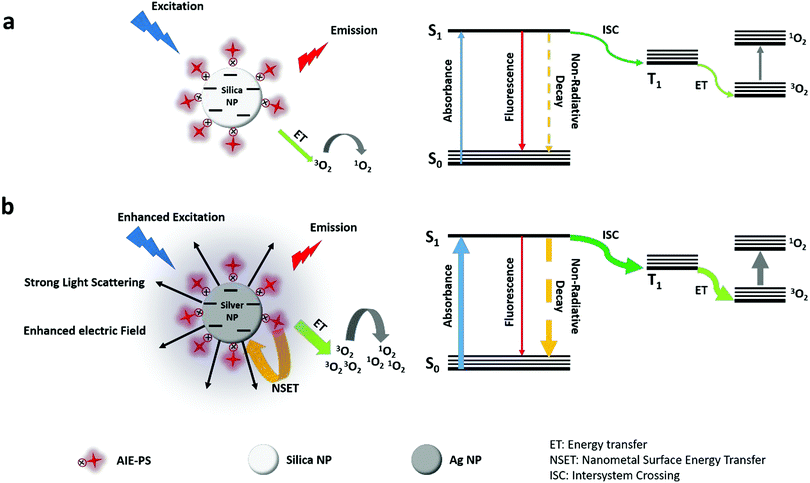 |
| Scheme 1 Simplified Jablonski diagram for the (a) SNP@AIE-PS hybrid and (b) Ag@AIE-PS hybrid. ET is the energy transfer from the excited AIE-PS (in the triplet state) to the surrounding oxygen molecules. ISC stands for the intersystem crossing process (S1 → T1). The thicker arrows refer to an enhanced rate in each process. In part (b), the purple glow and black arrows around the silver nanoparticle refer to the enhanced electric field around the AgNP and strong light scattering by the AgNP, respectively. | |
2.3. Cytotoxicity and application of AgNP@AIE-PS for fluorescence imaging and cancer cell ablation
Firstly, the cytotoxicity of the 80 nm AgNP@AIE-PS was evaluated using the MTT viability assay on both NIH-3T3 mammalian cells and HeLa cancer cells. Three control samples including (1) 80 nm AgNPs, (2) the AIE-PS and (3) SNP@AIE-PS prepared using the same concentration of AIE-PS were also subjected to the cell viability test (Fig. S5, ESI†). The results show that the AIE-PS possesses good cytotoxicity (i.e., cell viability >85% at a concentration <10 μM), while the presence of AgNPs causes a slight drop (∼5%) in the cell viability as compared with the SNP@AIE-PS control sample. Cellular imaging experiments were carried out upon incubating the 80 nm AgNP@AIE-PS with HeLa cells for 12 h prior to the cellular imaging experiments. A similar experiment was done using SNP@AIE-PS and the AIE-PS alone in PBS as controls. Surprisingly, the fluorescence image of HeLa cells in the presence of AgNP@AIE-PS (Fig. 5a) was much brighter than that with the AIE-PS alone in PBS (Fig. 5b), while a similar brightness was observed in the SNP@AIE-PS control sample (Fig. 5c). As the the fluorescence intensities of the three tested samples are similar (prior to the cellular experiments), the observed brighter cellular images could be due to the difference in the overall size of each sample (i.e., ∼80 nm AgNP@AIE-PS, 104 nm SNP@AIE-PS, and 160 nm AIE-PS aggregates in PBS43), leading to differential uptake efficiency by the HeLa cells. Therefore, lower cellular uptake for AIE-PS aggregates alone in PBS was observed as compared to the 80nm AgNP@AIE-PS sample.52,53 Next, the in vitro photodynamic efficiency of the 80 nm AgNP@AIE-PS was evaluated by treating HeLa cells under white light with a power of 40 mW cm−2. Fig. 5d shows the cell viability in the presence of 80 nm AgNP@AIE-PS after treating with white light for 10 minutes. The results show that the PDT treatment with 80 nm AgNP@AIE-PS can effectively kill the cancer cells (∼85% cell death, 10 μM AIE-PS) within 10 min, the performance of which is better than that using the AIE-PS alone (20% cell death) as well as the control sample (23% cell death) tested under the same conditions. This results shows the promising use of the AgNP-enhanced AIE-PS as a next-generation biocompatible photo-theranostic agent for simultaneous bioimaging and effective photodynamic therapy.
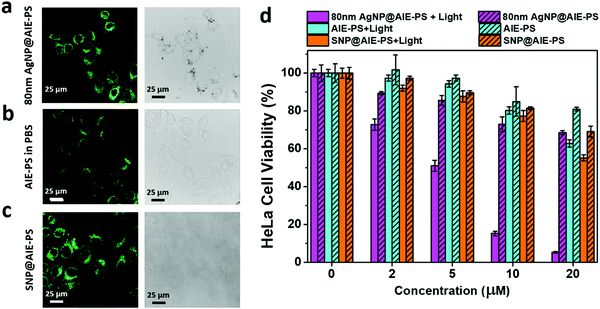 |
| Fig. 5 CLSM (left) and bright-field (right) images of HeLa cells incubated with 10 μM of (a) 80 nm AgNP@AIE-PS, (b) the AIE-PS in PBS, and (c) SNP@AIE-PS; (d) in vitro photodynamic therapy of HeLa cells treated with 80 nm AgNP@AIE-PS (cyan) and SNP@AIE-PS (violet) under white light irradiation (40 mW cm−2) for 10 minutes. Control studies showing cell viability in the presence of respective samples under dark conditions (pattern-filled). | |
3. Conclusions
In summary, a unique theranostic photosentizer comprising silver nanoparticles (AgNPs) and an AIE luminogen has been developed for simultaneous cellular imaging and photodynamic therapy (PDT). AgNP@AIE-PS was formed via a simple electrostatic-induced aggregation approach. It was found that the size and concentration of AgNPs were critical in dictating the metal-enhanced singlet oxygen generation (ME-SOG) property of the nanohybrids. Typically, larger AgNPs can lead to higher SOG enhancement at a lower AgNP concentration, among which the 80 nm AgNP@AIE-PS could achieve a 10-fold enhancement of the SOG capability compared to the AIE-PS alone. Simulation studies have been conducted to confirm that the enhanced singlet oxygen generation in AgNP@AIE-PS was due to the excellent scattering efficiency of metal NPs and electric field enhanced plasmonic coupling effect in the resulting hybrids. It is noteworthy that only minimum fluorescence quenching (5%) with a slight decrease in fluorescence lifetime was observed for the 80 nm AgNP@AIE-PS hybrid. Thus, it is bright enough to be used for simultaneous cellular imaging and photodynamic therapy. In summary, the as-developed AgNP@AIE-PS possesses many unique features, including ME-SOG, high fluorescent brightness, good photostability and low cytotoxicity, making it a promising next-generation multifunctional photosensitizer for theranostic applications.
4. Experimental section
4.1. Materials
Chemicals including silver nitrate, tannic acid (TA), 4-mercaptobenzoic acid (4-MBA), potassium carbonate (K2CO3), 9,10-anthracenediyl-bis(methylene)dimalonic acid (ABDA), Rose Bengal, 4-(dicyanomethylene)-2-methyl-6-(p-dimethylaminostyryl)-4H-pyran (DCM), ethanol, tetraethyl orthosilicate (TEOS), ammonia solution (25%), and phosphate buffer solution (PBS, 1×) were purchased from Sigma–Aldrich and used as received. Ultrapure water (18.2 MΩ cm) was used to prepare all aqueous solutions.
4.2. Synthesis of the water-soluble red-emissive AIE-based photosensitizer
The red-emissive AIE photosensitizer was synthesized according to a previous report.43
4.3. Synthesis of silica nanoparticles
The silica nanoparticles were synthesized according to the modified Stöber method reported in the literature.54,55 Briefly, a mixture of water (8.83 mL), ammonia solution (1 mL), and ethanol (5.67 mL) was prepared. Then, TEOS (1.35 mL) was dissolved in ethanol (13.65 mL) and added to the prepared solution dropwise with vigorous stirring. After 30 min at room temperature, the nanoparticles were washed with ethanol and water to remove unreacted chemicals. Finally, the obtained nanoparticles were dispersed in deionized water (25 mL) by 30 min bath ultrasonication. The final concentration of silica nanoparticles was 1.67 nM.33
4.4. Synthesis of 4-mercaptobenzoic acid-capped AgNPs
4-Mercaptobenzoic acid-capped AgNPs (4-MBA-AgNPs) were synthesized by a ligand exchange approach.56 First, tannic acid-capped AgNPs (TA-AgNPs) were synthesized according to the literature.57 Tannic acid was used as both the reducing and capping agent. Briefly, a tannic acid solution (500 μL, 5 mM) was added to deionized water (DI-water, 19 mL). Then, the solution pH was adjusted to 8, 6.5 and 6 with K2CO3 solution (0.05 M). Finally, AgNO3 (500 μL, 100 mM) was added to the above mixture under constant stirring. AgNPs of 14 nm and 50 nm were collected after 2 h of reaction and 80 nm AgNPs were collected after 6 h of reaction. The as-synthesized AgNPs were dialyzed against DI-water for 24 h prior to ligand exchange. To make 4-mercaptobenzoic acid-capped AgNPs, TA-AgNPs (20 mL) were transferred to a round-bottom flask, followed by addition of 4-MBA (2 mL, 1 mM in 0.1 M NaOH). The mixture solution was stirred vigorously for 2 h to complete the reaction. The final solution was collected and dialyzed against 0.01 M NaOH solution overnight. The concentrations of AgNPs in the final products were estimated to be 155 pM, 48.3 pM, and 4.23 pM for the 14 nm, 50 nm and 80 nm AgNP samples, respectively, according to the extinction molar coefficients reported in the literature.58
4.5. Preparation of AgNP@AIE-PS nanohybrids with maximum SOG enhancement
AgNP@AIE-PS nanohybrids with different sizes of the Ag core (i.e., 14 nm, 50 nm, and 80 nm) were prepared by the adsorption of positively-charged AIE-PS molecules onto the surface of negatively-charged 4-MBA-capped AgNPs. Briefly, an AIE-PS solution in DMSO (i.e., a final concentration of 9 μM) was mixed with the respective size AgNPs under different optimized conditions (i.e., 3 pM for 14 nm AgNPs, 2 pM for 50 nm AgNPs, and 0.22 pM for 80 nm AgNPs) to form the AgNP@AIE-PS nanohybrids with maximum SOG enhancement. The pH was set at 4 to maximize the electrostatic interactions between the AIE-PS molecules and AgNPs. The mixture was stirred for 20 min to complete the adsorption process.
4.6. Characterization
Fluorescence and UV-visible absorption spectra were recorded using an Infinite M-200 microplate reader and Shimadzu UV-2450 spectrophotometer, respectively. TEM images were obtained on a JEOL JEM-2010F transmission electron microscope operating at 200 kV. The zeta potential and hydraulic size of the samples were acquired on a Malvern Zetasizer at room temperature.
4.7. Fluorescence quantum yield measurements
The fluorescence quantum yield (QY) of the samples was measured using DCM as a reference in water. The quantum yield was calculated according to the following formula: | 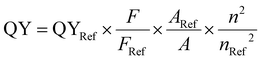 | (3) |
where QY and QYRef are the fluorescence quantum yields of the sample and reference, respectively. F and Fref are the integral of the fluorescence spectra of the sample and reference in the range of 400 nm to 800 nm, respectively. A and ARef refer to the absorbance of the sample and reference at the excitation wavelength (400 nm).
4.8. Detection of singlet oxygen (1O2) generation
The singlet oxygen generation rate was measured using ABDA as an indicator. The AgNP@AIE-PS hybrid (1 mL, with a final AIE-PS concentration of 9 μM) was transferred to a disposable cuvette (1 mL). The sample was irradiated with 40 mW cm−2 white light in the presence of ABDA (a final concentration of 50 μM). The absorbance of the sample was measured every 10 min, and the rate of ABDA degradation was calculated using the following first-order kinetic model:59 | 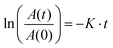 | (4) |
where A(t) and A(0) are the absorbance of ABDA at 379 nm at time t and 0, respectively. K is the first-order kinetic constant for degradation of ABDA and t is time. The absorbance of each photosensitizer sample was subtracted from the observed absorbance of ABDA before calculation of the degradation rate.
4.9.
1O2 quantum yield measurements
The 1O2 quantum yield was measured by a chemical trapping method using ABDA as a 1O2 selective indicator. Water-soluble Rose Bengal was selected as a reference photosensitizer. Briefly, an aqueous solution (1 mL) containing AgNP@AIE-PS or Rose Bengal (5 μM) and 50 μM ABDA was prepared and subjected to white light irradiation at the same conditions as aforementioned. The degradation rate of ABDA was monitored by measuring its absorbance intensity at 379 nm under irradiation over time. The 1O2 quantum yield was calculated using the following equation: | 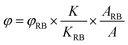 | (5) |
where K and KRB are the degradation rate of ABDA by the test sample and Rose Bengal, respectively. A and ARB are the integral of the absorbance spectra data for the sample and Rose Bengal in the wavelength range of 400–800 nm. φRB is the 1O2 quantum yield of Rose Bengal in water, which is 75%.
4.10. Time-resolved fluorescence spectroscopy
The as-prepared AgNP@AIE-PS samples were excited at 374 nm using a FluoTime 200 TCSPC machine (Picoquant BmbH, Germany) for fluorescent lifetime measurements. The fluorescence decay profile of the samples was collected at 625 nm and modeled using the two-exponential equation below: |  | (6) |
where I(t) is the fluorescence intensity at time t, τi is the decay time, αi is the amplitude, and
. The average life-time can be written as: |  | (7) |
where the contribution fraction of each component (fi) is given as follows: |  | (8) |
The curve fitting was performed using the curve-fitting toolbox in Matlab 2014b software.
4.11. Cell culture
HeLa cells and NIH-3T3 cells were pre-cultured in Dulbecco's Modified Eagle Medium (DMEM) with 10% Fetal Bovine Serum (FBS) and 1% penicillin–streptomycin at 37 °C in a humidified incubator containing 5% CO2.
4.12. Cellular imaging
After reaching confluence, pre-cultured cells were seeded in 8-well chambered cover glass (Nunc Lab-Tek, Thermo Scientific). After adherence, fresh DMEM containing the AIE-PS or 80 nm AgNP@AIE-PS was added and incubated for 12 h at 10 μM (based on the AIE-PS). FBS-free DMEM was used during CLSM imaging. Cell images were taken using a Leica SP8X laser scanning confocal microscope.
4.13. Cytotoxicity study
Methylthiazolyldiphenyltetrazolium bromide (MTT) assay was used to evaluate the cytotoxicity of the AgNP@AIE-PS hybrid system. For the dark cytotoxicity, pre-cultured cells were seeded into 96-well plates at a density of 4000 cells per well. After reaching confluence, fresh DMEM containing the AIE-PS and 80 nm AgNP@AIE-PS was added and incubated for 24 hours at different concentrations. The medium was replaced by 0.5 mg mL−1 methylthiazolyldiphenyltetrazolium bromide in the medium for 4 hours, and DMSO was used to dissolve non-soluble formazan. The absorbance was measured by a microplate reader at 570 nm. For the photodynamic therapy (PDT) study, cells were treated for the same time period at the same concentrations. After changing to fresh medium, 10 min white light irradiation at 40 mW cm−2 was applied to the cells, and the treated cells were further cultured for 6 hours. Standard MTT assay was performed after the PDT study to evaluate the light treatment efficiency. Each condition was repeated three times to calculate the standard deviation.
4.14. Simulation study
In order to calculate the electric field and scattering cross-section of spherical AgNPs, finite element method (FEM) simulations were carried out in the COMSOL multiphysics software package (see http://www.comsol.com). The complex refractive index of Ag was taken from the CRC handbook.60 Water was used as the surrounding environment. A plane wave propagating in the x-direction and linearly polarized along the z-axis spanning the wavelength range of 300 nm to 700 nm was set as the source. Due to the symmetry of the problem, only one-quarter of the sphere was modeled.
Conflicts of interest
There are no conflicts to declare.
Acknowledgements
M. T. Y. would like to thank Dr Soroosh Daqiqeh Rezaei for his valuable help and discussion on the simulation part. M. T. Y. also would like to thank the Agency of Science, Technology and Research, Singapore (A*STAR) for financial support via the SINGA scholarship. This work was supported by the Institute of Materials Research and Engineering, A*STAR, under Biomimetic and Biomedical Materials program (IMRE/00-1P1400), in collaboration with Newcastle University (RSA/CCEAMD5010), UK and Singapore. The financial support from National University of Singapore (R279-000-482-133) and National Research Foundation (R279-000-444-281) is also appreciated.
References
- A. Gupta, S. Wang, P. Pera, K. Rao, N. Patel, T. Y. Ohulchanskyy, J. Missert, J. Morgan, Y.-E. Koo-Lee and R. Kopelman, Multifunctional nanoplatforms for fluorescence imaging and photodynamic therapy developed by post-loading photosensitizer and fluorophore to polyacrylamide nanoparticles, J. Nanomed. Nanotechnol., 2012, 8, 941–950 CrossRef CAS PubMed.
- J. P. Celli, B. Q. Spring, I. Rizvi, C. L. Evans, K. S. Samkoe, S. Verma, B. W. Pogue and T. Hasan, Imaging and photodynamic therapy: mechanisms, monitoring, and optimization, Chem. Rev., 2010, 110, 2795–2838 CrossRef CAS PubMed.
- J. F. Lovell, T. W. Liu, J. Chen and G. Zheng, Activatable photosensitizers for imaging and therapy, Chem. Rev., 2010, 110, 2839–2857 CrossRef CAS PubMed.
- Y. Yu, W. D. Lee and Y. N. Tan, Protein-protected gold/silver alloy nanoclusters in metal-enhanced singlet oxygen generation and their correlation with photoluminescence, Mater. Sci. Eng., C, 2020, 109, 110525 CrossRef CAS PubMed.
- X. T. Zheng, Y. C. Lai and Y. N. Tan, Nucleotide-derived theranostic nanodots with intrinsic fluorescence and singlet oxygen generation for bioimaging and photodynamic therapy, Nanoscale Adv., 2019, 1, 2250–2257 RSC.
- Y. Yu, J. Geng, E. Y. X. Ong, V. Chellappan and Y. N. Tan, Bovine Serum Albulmin Protein-Templated Silver Nanocluster (BSA-Ag13): An Effective Singlet Oxygen Generator for Photodynamic Cancer Therapy, Adv. Healthcare Mater., 2016, 5, 2528–2535 CrossRef CAS PubMed.
- Y. Choi, X. T. Zheng and Y. N. Tan, Bioinspired carbon dots (biodots): emerging fluorophores with tailored multiple functionalities for biomedical, agricultural and environmental applications, Mol. Syst. Des. Eng., 2020, 5, 67–90 RSC.
- T. J. Dougherty, C. J. Gomer, B. W. Henderson, G. Jori, D. Kessel, M. Korbelik, J. Moan and Q. Peng, Photodynamic therapy, J. Natl. Cancer Inst., 1998, 90, 889–905 CrossRef CAS PubMed.
- E. S. Marmur, C. D. Schmults and D. J. Goldberg, A review of laser and photodynamic therapy for the treatment of nonmelanoma skin cancer, Dermatol. Surg., 2004, 30, 264–271 Search PubMed.
- J. Fuchs and J. Thiele, The role of oxygen in cutaneous photodynamic therapy, Free Radical Biol. Med., 1998, 24, 835–847 CrossRef CAS PubMed.
- M. A. Doustvandi, F. Mohammadnejad, B. Mansoori, H. Tajalli, A. Mohammadi, A. Mokhtarzadeh, E. Baghbani, V. Khaze, K. Hajiasgharzadeh, M. M. Moghaddam, M. R. Hamblin and B. Baradaran, Photodynamic therapy using zinc phthalocyanine with low dose of diode laser combined with doxorubicin is a synergistic combination therapy for human SK-MEL-3 melanoma cells, Photodiagn. Photodyn. Ther., 2019, 28, 88–97 CrossRef CAS PubMed.
- L. B. Negri, T. J. Martins, R. S. da Silva and M. R. Hamblin, Photobiomodulation combined with photodynamic therapy using ruthenium phthalocyanine complexes in A375 melanoma cells: Effects of nitric oxide generation and ATP production, J. Photochem. Photobiol., B, 2019, 198, 111564 CrossRef CAS PubMed.
- Z. Zhou, J. Song, L. Nie and X. Chen, Reactive oxygen species generating systems meeting challenges of photodynamic cancer therapy, Chem. Soc. Rev., 2016, 45, 6597–6626 RSC.
- L. Huang, B. Bhayana, W. Xuan, R. P. Sanchez, B. J. McCulloch, S. Lalwani and M. R. Hamblin, Comparison of two functionalized fullerenes for antimicrobial photodynamic inactivation: Potentiation by potassium iodide and photochemical mechanisms, J. Photochem. Photobiol., B, 2018, 186, 197–206 CrossRef CAS PubMed.
- W. Xuan, Y. He, L. Huang, Y.-Y. Huang, B. Bhayana, L. Xi, J. A. Gelfand and M. R. Hamblin, Antimicrobial Photodynamic Inactivation Mediated by Tetracyclines in Vitro and in Vivo: Photochemical Mechanisms and Potentiation by Potassium Iodide, Sci. Rep., 2018, 8, 17130 CrossRef PubMed.
-
J. B. Birks, Photophysics of aromatic molecules, 1970 Search PubMed.
- C. Tanielian, C. Wolff and M. Esch, Singlet oxygen production in water: aggregation and charge-transfer effects, J. Phys. Chem., 1996, 100, 6555–6560 CrossRef CAS.
- J. F. Lovell, C. S. Jin, E. Huynh, H. Jin, C. Kim, J. L. Rubinstein, W. C. Chan, W. Cao, L. V. Wang and G. Zheng, Porphysome nanovesicles generated by porphyrin bilayers for use as multimodal biophotonic contrast agents, Nat. Mater., 2011, 10, 324 CrossRef CAS PubMed.
- J. F. Lovell, J. Chen, M. T. Jarvi, W.-G. Cao, A. D. Allen, Y. Liu, T. T. Tidwell, B. C. Wilson and G. Zheng, FRET quenching of photosensitizer singlet oxygen generation, J. Phys. Chem. B, 2009, 113, 3203–3211 CrossRef CAS PubMed.
- F. Hu, D. Mao, Y. Wang, W. Wu, D. Zhao, D. Kong and B. Liu, Metal–Organic Framework as a Simple and General Inert Nanocarrier for Photosensitizers to Implement Activatable Photodynamic Therapy, Adv. Funct. Mater., 2018, 28, 1707519 CrossRef.
- W. Wu, D. Mao, F. Hu, S. Xu, C. Chen, C. J. Zhang, X. Cheng, Y. Yuan, D. Ding and D. Kong, A Highly Efficient and Photostable Photosensitizer with Near-Infrared Aggregation-Induced Emission for Image-Guided Photodynamic Anticancer Therapy, Adv. Mater., 2017, 29, 1700548 CrossRef PubMed.
- F. Hu, S. Xu and B. Liu, Photosensitizers with Aggregation-Induced Emission: Materials and Biomedical Applications, Adv. Mater., 2018, 30, 1801350 CrossRef PubMed.
- J. Geng, W. L. Goh, C. Zhang, D. P. Lane, B. Liu, F. Ghadessy and Y. N. Tan, A highly sensitive fluorescent light-up probe for real-time detection of the endogenous protein target and its antagonism in live cells, J. Mater. Chem. B, 2015, 3, 5933–5937 RSC.
- F. Hu, Y. Yuan, W. Wu, D. Mao and B. Liu, Dual-Responsive Metabolic Precursor and Light-Up AIEgen for Cancer Cell Bio-orthogonal Labeling and Precise Ablation, Anal. Chem., 2018, 90, 6718–6724 CrossRef CAS PubMed.
- F. Hu, D. Mao, X. Cai, W. Wu, D. Kong and B. Liu, A Light-Up Probe with Aggregation-Induced Emission for Real-Time Bio-orthogonal Tumor Labeling and Image-Guided Photodynamic Therapy, Angew. Chem., 2018, 130, 10339–10343 CrossRef.
- F. Hu, S. Xu and B. Liu, Photosensitizers with Aggregation-Induced Emission: Materials and Biomedical Applications, Adv. Mater., 2018, 1801350 CrossRef PubMed.
- J. Ferreira, P. F. Menezes, C. Kurachi, C. Sibata, R. Allison and V. S. Bagnato, Photostability of different chlorine photosensitizers, Laser Phys. Lett., 2007, 5, 156 CrossRef.
- Y. Zhang, K. Aslan, M. J. Previte and C. D. Geddes, Metal-enhanced singlet oxygen generation: a consequence of plasmon enhanced triplet yields, J. Fluoresc., 2007, 17, 345–349 CrossRef CAS PubMed.
- Y. Zhang, K. Aslan, M. J. Previte and C. D. Geddes, Plasmonic engineering of singlet oxygen generation, Proc. Natl. Acad. Sci. U. S. A., 2008, 105, 1798–1802 CrossRef CAS PubMed.
- C. D. Geddes and J. R. Lakowicz, Metal-enhanced fluorescence, J. Fluoresc., 2002, 12, 121–129 CrossRef.
- K. Aslan, I. Gryczynski, J. Malicka, E. Matveeva, J. R. Lakowicz and C. D. Geddes, Metal-enhanced fluorescence: an emerging tool in biotechnology, Curr. Opin. Biotechnol, 2005, 16, 55–62 CrossRef CAS PubMed.
- J. R. Lakowicz, Radiative decay engineering 5: metal-enhanced fluorescence and plasmon emission, Anal. Biochem., 2005, 337, 171–194 CrossRef CAS PubMed.
- S. Dinda, F. L. Yap, V. Suresh, R. K. Gupta, D. Das and S. Krishnamoorthy, Quantitative Detection with Surface Enhanced Raman Scattering (SERS) Using Self-Assembled Gold Nanoparticle Cluster Arrays, Aust. J. Chem., 2013, 66, 1034–1038 CrossRef CAS.
- M. Tavakkoli Yaraki, S. Daqiqeh Rezaei and Y. N. Tan, Simulation guided design of silver nanostructures for plasmon-enhanced fluorescence, singlet oxygen generation and SERS applications, Phys. Chem. Chem. Phys., 2020, 22, 5673–5687 RSC.
- M. Tavakkoli Yaraki, S. Daqiqeh Rezaei, E. Middha and Y. N. Tan, Synthesis and Simulation Study of Right Silver Bipyramids via Seed-Mediated Growth cum Selective Oxidative Etching Approach, Part. Part. Syst. Charact., 2020, 37, 2000027 CrossRef CAS.
- M. K. Khaing Oo, Y. Yang, Y. Hu, M. Gomez, H. Du and H. Wang, Gold nanoparticle-enhanced and size-dependent generation of reactive oxygen species from protoporphyrin IX, ACS Nano, 2012, 6, 1939–1947 CrossRef CAS PubMed.
- M. Tavakkoli Yaraki, F. Hu, S. Daqiqeh Rezaei, B. Liu and Y. N. Tan, Metal-enhancement study of dual functional photosensitizers with aggregation-induced emission and singlet oxygen generation, Nanoscale Adv., 2020, 2, 2859–2869 RSC.
- N. Macia, R. Bresoli-Obach, S. Nonell and B. Heyne, Hybrid Silver Nanocubes for Improved Plasmon-Enhanced Singlet Oxygen Production and Inactivation of Bacteria, J. Am. Chem. Soc., 2018, 141, 684–692 CrossRef PubMed.
- O. Planas, N. Macia, M. Agut, S. Nonell and B. Heyne, Distance-dependent plasmon-enhanced singlet oxygen production and emission for bacterial inactivation, J. Am. Chem. Soc., 2016, 138, 2762–2768 CrossRef CAS PubMed.
- Y. Cheng, A. C. Samia, J. D. Meyers, I. Panagopoulos, B. Fei and C. Burda, Highly efficient drug delivery with gold nanoparticle vectors for in vivo photodynamic therapy of cancer, J. Am. Chem. Soc., 2008, 130, 10643–10647 CrossRef CAS PubMed.
- N. L. Pacioni, M. a. González-Béjar, E. Alarcón, K. L. McGilvray and J. Scaiano, Surface plasmons control the dynamics of excited triplet states in the presence of gold nanoparticles, J. Am. Chem. Soc., 2010, 132, 6298–6299 CrossRef CAS PubMed.
- M. A. B. N. Rivas Aiello, J. J. Romero, S. G. Bertolotti, M. N. C. Gonzalez and D. O. Mártire, Effect of Silver Nanoparticles on the Photophysics of Riboflavin: Consequences on the ROS Generation, J. Phys. Chem. C, 2016, 120, 21967–21975 CrossRef CAS.
- F. Hu, X. Cai, P. N. Manghnani, W. Wu and B. Liu, Multicolor monitoring of cellular organelles by single wavelength excitation to visualize the mitophagy process, Chem. Sci., 2018, 9, 2756–2761 RSC.
- S. Xu, Y. Yuan, X. Cai, C.-J. Zhang, F. Hu, J. Liang, G. Zhang, D. Zhang and B. Liu, Tuning the singlet-triplet energy gap: a unique approach to efficient photosensitizers with aggregation-induced emission (AIE) characteristics, Chem. Sci., 2015, 6, 5824–5830 RSC.
- Y. Chen, K. Munechika and D. S. Ginger, Dependence of Fluorescence Intensity on the Spectral Overlap between Fluorophores and Plasmon Resonant Single Silver Nanoparticles, Nano Lett., 2007, 7, 690–696 CrossRef CAS PubMed.
- J. John, L. Thomas, N. A. George, A. Kurian and S. D. George, Tailoring of optical properties of fluorescein using green synthesized gold nanoparticles, Phys. Chem. Chem. Phys., 2015, 17, 15813–15821 RSC.
- D. Ghosh, A. Girigoswami and N. Chattopadhyay, Superquenching of coumarin 153 by gold nanoparticles, J. Photochem. Photobiol., A, 2012, 242, 44–50 CrossRef CAS.
-
V. May and O. Kühn, Charge and energy transfer dynamics in molecular systems, John Wiley & Sons, 2008 Search PubMed.
- R. Chance, A. Prock and R. Silbey, Molecular fluorescence and energy transfer near interfaces, Adv. Chem. Phys., 1978, 37, 1–65 CAS.
- C. Yun, A. Javier, T. Jennings, M. Fisher, S. Hira, S. Peterson, B. Hopkins, N. Reich and G. Strouse, Nanometal surface energy transfer in optical rulers, breaking the FRET barrier, J. Am. Chem. Soc., 2005, 127, 3115–3119 CrossRef CAS PubMed.
- P. Pompa, L. Martiradonna, A. Della Torre, F. Della Sala, L. Manna, M. De Vittorio, F. Calabi, R. Cingolani and R. Rinaldi, Metal-enhanced fluorescence of colloidal nanocrystals with nanoscale control, Nat. Nanotechnol., 2006, 1, 126 CrossRef CAS PubMed.
- X.-Y. Sun, Q.-Z. Gan and J.-M. Ouyang, Size-dependent cellular uptake mechanism and cytotoxicity toward calcium oxalate on Vero cells, Sci. Rep., 2017, 7, 41949 CrossRef CAS PubMed.
- E. Oh, J. B. Delehanty, K. E. Sapsford, K. Susumu, R. Goswami, J. B. Blanco-Canosa, P. E. Dawson, J. Granek, M. Shoff, Q. Zhang, P. L. Goering, A. Huston and I. L. Medintz, Cellular Uptake and Fate of PEGylated Gold Nanoparticles Is Dependent on Both Cell-Penetration Peptides and Particle Size, ACS Nano, 2011, 5, 6434–6448 CrossRef CAS PubMed.
- W. Stöber, A. Fink and E. Bohn, Controlled growth of monodisperse silica spheres in the micron size range, J. Colloid Interface Sci., 1968, 26, 62–69 CrossRef.
- Y. Wang and B. Liu, Conjugated polyelectrolyte-sensitized fluorescent detection of thrombin in blood serum using aptamer-immobilized silica nanoparticles as the platform, Langmuir, 2009, 25, 12787–12793 CrossRef CAS PubMed.
- J. V. Maya Girón, E. Zelaya, A. Rubert, G. Benítez, P. Carro, R. C. Salvarezza and M. E. Vela, Surface chemistry of 4-mercaptobenzoic acid self-assembled on Ag(111) and Ag nanoparticles, J. Phys. Chem. C, 2013, 117, 24967–24974 CrossRef.
- Y. Cao, R. Zheng, X. Ji, H. Liu, R. Xie and W. Yang, Syntheses and characterization of nearly monodispersed, size-tunable silver nanoparticles over a wide size range of 7–200 nm by tannic acid reduction, Langmuir, 2014, 30, 3876–3882 CrossRef CAS PubMed.
- D. Paramelle, A. Sadovoy, S. Gorelik, P. Free, J. Hobley and D. G. Fernig, A rapid method to estimate the concentration of citrate capped silver nanoparticles from UV-visible light spectra, Analyst, 2014, 139, 4855–4861 RSC.
- N. A. Kuznetsova, N. S. Gretsova, O. A. Yuzhakova, V. M. Negrimovskii, O. L. Kaliya and E. A. Luk'yanets, New Reagents for Determination of the Quantum Efficiency of Singlet Oxygen Generation in Aqueous Media, Russ. J. Gen. Chem., 2001, 71, 36–41 CrossRef CAS.
-
J. F. Shackelford, Y.-H. Han, S. Kim and S.-H. Kwon, CRC materials science and engineering handbook, CRC Press, 2016 Search PubMed.
Footnotes |
† Electronic supplementary information (ESI) available. See DOI: 10.1039/d0qm00469c |
‡ Y. P. and F. H. contributed equally to this work. |
|
This journal is © the Partner Organisations 2020 |
Click here to see how this site uses Cookies. View our privacy policy here.