DOI:
10.1039/D0QM00323A
(Review Article)
Mater. Chem. Front., 2020,
4, 2571-2609
Organic/inorganic nanocomposites for cancer immunotherapy
Received
15th May 2020
, Accepted 12th June 2020
First published on 13th June 2020
Abstract
Cancer immunotherapy provides an effective way to deal with cancer. Although immunotherapy strategies have shown encouraging therapeutic effects, the inherent limitations of immunotherapy, such as multiple tumor immune evasion methods, low response rate, and systemic toxicity, still hinder its clinical applications. In recent decades, nanomaterials have been considered promising in cancer immunotherapy since they can realize targeted delivery and interact with the immune system to induce or enhance the antitumor immune responses. Among them, organic/inorganic nanocomposites are ideal candidates for cancer immunotherapy since they could combine the advantages of both organic and inorganic components. Multifunctional organic/inorganic nanocomposites could help overcome the shortcomings of current cancer immunotherapy, and realize the combination of immunotherapy and other therapeutic strategies with synergistic antitumor effects. Herein, we review the recent progress of organic/inorganic nanocomposites designed for cancer immunotherapy. The immunotherapy strategies of nanocomposites are summarized from the perspective of achieving immune enhancement. The challenges of nanocomposites in cancer immunotherapy are also discussed.
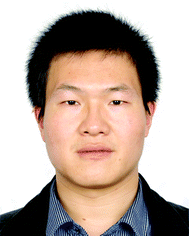
Mingqiang Hao
| Dr Mingqiang Hao received his PhD degree in Medicine from the Chinese Center for Disease Control and Prevention in 2017. He then worked at the Beijing Center for Disease Control and Prevention as a medical researcher for the control of HIV infection. In 2019, he joined Beijing University of Chemical Technology as a postdoctoral researcher. His current research interests focus on the applications of organic/inorganic nanocomposites and novel immune adjuvants in cancer immunotherapy. |
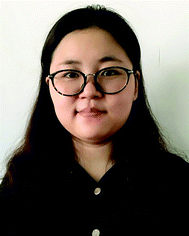
Beibei Chen
| Beibei Chen received her BS degree from Beijing University of Chemical Technology in 2018. She is currently a master student under the supervision of Prof. Fu-Jian Xu and Prof. Nana Zhao at Beijing University of Chemical Technology. Her research interests focus on the design and synthesis of nanocomposites with special morphologies for cancer immunotherapy. |
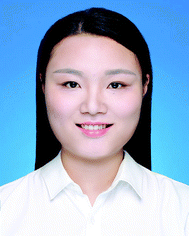
Xiaoyi Zhao
| Xiaoyi Zhao received her BS from Beijing University of Chemical Technology, China, in 2017. She is currently a PhD student under the supervision of Prof. Fu-Jian Xu and Prof. Nana Zhao at Beijing University of Chemical Technology. Her current research interests focus on the rational design and synthesis of nanocomposites and their related biomedical applications. |
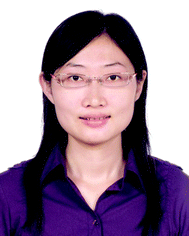
Nana Zhao
| Prof. Nana Zhao is currently a professor at Beijing University of Chemical Technology. She obtained her PhD degree in physical chemistry from Peking University, China, in 2008 under the supervision of Prof. Limin Qi, and was a postdoctoral scholar with Prof. Eugenia Kumacheva at the University of Toronto, Canada, and Prof. Lutgard De Jonghe at Lawrence Berkeley National Laboratory. She joined Beijing University of Chemical Technology, China, in 2012. She was a recipient of the National Science Fund for Outstanding Young Scholars (NSFC, 2019). Her current research focuses on the design, synthesis, and application of versatile organic/inorganic nanocomposites. |
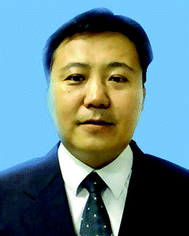
Fu-Jian Xu
| Prof. Fu-Jian Xu is the Executive Director of Beijing Laboratory of Biomedical Materials, Beijing University of Chemical Technology. His research interests focus on functional biomacromolecules. He was a recipient of the National Science Fund for Distinguished Young Scholars (NSFC, 2013), Cheung Kong Distinguished Professor (Ministry of Education of China, 2014) and Beijing Outstanding Young Scientist Program (2018). |
1. Introduction
Cancer threatens human health and causes leading death worldwide,1 and has been recognized as incurable for a long time. Immunotherapy provides a promising strategy for cancer treatment.2 Immunotherapy directly focuses on amplifying anti-tumor immune responses and modulating the tumor immunosuppressive microenvironment.3 Immunotherapy mainly includes cytokine therapy, tumor vaccines, immune checkpoint blockade (ICB) therapy, chimeric antigen receptor T-cell (CAR-T) therapy and so on.4 The use of bacteria to activate the immune responses and kill tumors is the earliest embryonic form of immunotherapy.5 However, due to the limited efficacy, it has not received much attention. In the 1980s and 1990s, the US Food and Drug Administration (FDA) approved the treatment of interferon-α (IFN-α, leukemia) and IL-2 (renal cell carcinoma (RCC) and melanoma), respectively, for cancer immunotherapy. In 2004, the US FDA approved imiquimod, IL-12, tumor necrosis factor α (TNF-α) and interferon-γ (IFN-γ). In 2010, the US FDA approved the first therapeutic tumor vaccine, an autologous dendritic cell (DC) vaccine, for the treatment of prostate cancer. In 2011, the high-performance ICB therapy antibody CTAL-4 was approved for the treatment of melanoma. Subsequently, inhibitors related to programmed cell death protein 1 (PD-1)/programmed death-ligand 1 (PD-L1) were approved and significant clinical results were achieved. CAR-T cell adoptive therapy was approved in 2017.6
Although cancer immunotherapy has brought dawn to the treatment of tumors, there are still some problems that need to be solved, such as low clinical response rates, immune-related adverse events, atypical clinical reactions, and so on.3 For cytokine therapy, the use of high doses of cytokines to ensure durable responses in patients might cause serious toxic reactions such as fever, nausea and vomiting, and metabolic acidosis.7 Tumor vaccines aim to fight against tumors by introducing specific immune responses activated by strong immunogenic tumor antigens. However, several studies have shown that although tumor vaccines could induce tumor-specific T cell responses, they often failed in the control of tumor growth in the clinic.8 ICB therapy involves the blockade of inhibitory signals to amplify antitumor immune responses and release the brake of the immune system. Although ICB therapy launched a new era in cancer immunotherapy and induced a durable clinical benefit, drug resistance and side effects still limit the use of this therapy.9,10 T-cell-related cellular immunotherapy produces anti-tumor effects by stimulation in vitro or reinfusion of artificially genetically modified T cells into patients. CAR-T cellular immunotherapy such as anti CD19 CAR-T therapy has shown encouraging clinical results among patients with lymphoma. However, this therapy still needs to be strengthened to improve the therapeutic effect on solid tumors.11,12 The cost of treatment, the complexity of preparation, and the adverse effects of treatment all need to be addressed.13,14
On the other hand, nanomaterials have attracted remarkable attention in cancer immunotherapy due to their advantages in targeted delivery, sustained and controlled release of bioactive molecules, facile surface functionalization, high performance in immune response activation, and combination therapy.15–17 Nanomaterials can enhance the therapeutic effect of immunotherapy in the following aspects. Firstly, targeting the immunosuppressive environment to improve the clinical response rate of immunotherapy. Some nanomaterials can effectively reverse the immunosuppression (such as reeducation of M2 type immunosuppressive macrophages) and then improve the immune response by targeting the tumor immunosuppressive microenvironment.18,19 Then nanomaterials could activate the immune responses by interacting with immune cells or tumor cells and further promote the therapeutic effect of immunotherapy.20 In addition, nanomaterials could serve as a targeted delivery system for immunotherapy to increase the enrichment and accumulation of immunotherapy-related drugs or active substances (such as adjuvants, tumor antigens, and checkpoint antibodies) in tumor areas, and further reduce the side effects of immunotherapy.17 Moreover, some multifunctional nanomaterials could introduce chemotherapy, radiotherapy, thermotherapy and photodynamic therapy. These therapies may lead to the release of tumor antigens and induce immune responses when killing tumor cells to achieve synergistic effectiveness.10
Among nanomaterials, polymeric nanomaterials and inorganic nanoparticles with favorable properties show great potential and have been investigated extensively in immunotherapy.21–23 Organic/inorganic nanocomposites integrate the advantages of organic and inorganic components, and could also produce synergistic effects. In addition, versatile functions could be realized through rational design of the composition, size, and morphology. Therefore, organic/inorganic nanocomposites get a broader application space and development prospects in cancer immunotherapy. Through the design of the composition, size and morphology of nanocomposites to modulate the functions, the immunosuppressive tumor microenvironment (TME) could be adjusted, the anti-tumor immune response could be more strongly activated, and ICB treatment could be enhanced to overcome immune evasion and low response rates in immunotherapy. In addition, the rational design of organic and inorganic components can also enhance the targeting function and responsiveness to the TME to reduce the dosage, thus reducing systemic toxicity. In this review, we will introduce the advantages of nanocomposites and summarize their applications in the following chapters. Detailed information on how nanocomposites settled the challenges in immunotherapy is discussed in the respects of targeting the immunosuppressive TME, activating antitumor immune responses, ICB therapy and combination of immunotherapy strategies (Fig. 1).
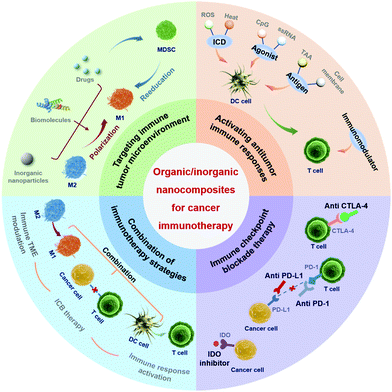 |
| Fig. 1 The application of organic/inorganic nanocomposites in cancer immunotherapy. | |
2. The advantage of organic/inorganic nanocomposites in immunotherapy
In this review, organic/inorganic nanocomposites refer to nanoparticles integrating organic and inorganic components (Fig. 2),24 which are promising in a wide range of biological applications.25–27 Among them, organic components mainly include small molecules, polymers, biomolecules (including polysaccharides), drugs, photosensitizers, immune active substances, etc. Inorganic components mainly refer to inorganic nanoparticles and inorganic ions. The favorable optical, magnetic, and chemical properties of nanocomposites could be utilized in immunotherapy. Organic/inorganic nanocomposites can combine the functions of both organic and inorganic components, which could also produce synergistic effects to promote immunotherapy. Meanwhile, the morphology and size effects of organic/inorganic nanocomposites on the immune system can be investigated. In this part, we mainly introduce the advantages of organic/inorganic nanocomposites for cancer immunotherapy.
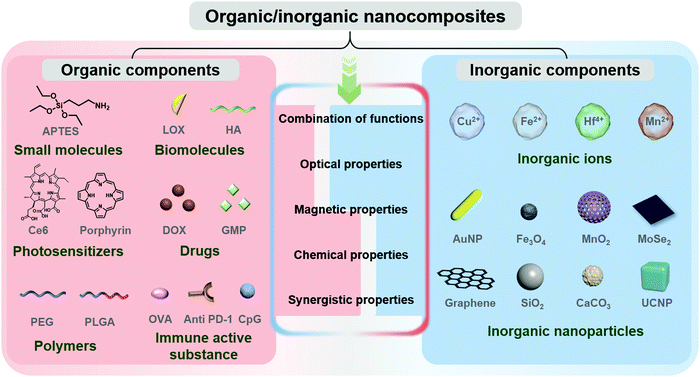 |
| Fig. 2 Complementary compositions of organic and inorganic components of nanocomposites. | |
2.1. Combination of functions
Organic/inorganic nanocomposites can integrate components with different optical,28 magnetic,29 and chemical properties through materials design to achieve dispersibility,30 targeting,31,32 and responsiveness.33,34 For example, synthetic polymers such as poly(ethylene glycol) (PEG)35,36 or biomolecules37 can be introduced to increase the dispersibility and circulation time of nanocomposites in vivo. In addition to the enhanced permeability and retention (EPR) effect,38 targeting molecules such as hyaluronic acid (HA),39 folic acid (FA),40 and anti PD-1 antibody41 can also be introduced to improve the targeting of the nanocomposites. It is also feasible to design chemical bonds or inorganic nanoparticles that respond to the TME in nanocomposites to achieve TME-responsiveness. Moreover, immune active substances such as antigens and antibodies could be introduced into nanocomposites to directly enhance the immune responses.
2.2. Optical properties
Regarding optical properties, we mainly focus on the absorption properties which are associated with the activation of immune responses. Through hyperthermia or the generation of reactive oxygen species (ROS),42 organic/inorganic nanocomposites with strong absorption in the near-infrared (NIR) region can cause immunogenic cell death (ICD).10 In addition, the mild heating generated by the photothermal properties can reduce the tissue osmotic density between solid tumors and expand tumor blood vessels by destroying tumor cells and the extracellular matrix, thereby enhancing the infiltration of immune cells into tumors.43
Moreover, photothermal nanocomposites could result in thermal elastic expansion and generate ultrasonic pressure waves under a pulsed laser, which can be used as contrast agents for photoacoustic (PA) imaging,44 which could be used to locate the nanocomposites at tumor sites.45 PA imaging was proved to track the immune responses caused by nanocomposites and may provide guidance for cancer immunotherapy.46
2.3. Magnetic properties
Nanocomposites comprising magnetic nanoparticles or ions demonstrate intriguing magnetic properties and related functions.47 Herein, we mainly focus on magnetic targeting, magnetic hyperthermia and magnetic resonance imaging (MRI) in relation to immunotherapy.
Under a static constant magnetic field, magnetic nanocomposites could be targeted to increase the concentration of the material at the tumor site and enhance the interaction with the immune system.48 At the same time, the intratumoral retention of reprogrammed macrophages with magnetic nanocomposites could be prolonged.49 Under an alternating magnetic field, magnetic hyperthermia caused by the high saturation magnetization could damage tumor cells and release tumor-associated antigens.47
In addition, MRI could be utilized to monitor the enrichment of nanocomposites in real time and guide anti-tumor therapy.50–52 Similar to PA imaging, the MRI results can be used to detect the activation of immune responses. In addition, nanocomposites with an MRI function also provide guidance on the treatment time or dose to increase the release of tumor antigens and optimize the treatment effect.46
2.4. Chemical properties
Chemical reactions could occur between nanocomposites and H+, H2O2, glutathione (GSH), etc., which exist in the TME.53 The effect of nanocomposites on immune responses is mainly achieved through the chemical reaction of the nanomaterials with H2O2.
Organic/inorganic nanocomposites comprising MnO2, Fe3O4, catalase, lactate oxidase (LOX), etc. can trigger the decomposition of H2O2 in the TME to H2O and O2, thereby alleviating tumor hypoxia.54 Nanocomposites can also be designed to generate H2O2 and improve the oxygen production efficiency.55 The increase of M2 macrophages in the immune TME is related to hypoxia. Relieving hypoxia can polarize M2 macrophages into M1 macrophages and enhance cancer treatment.56 On the other hand, some nanocomposites could decompose H2O2 by the Fenton reaction to generate ˙OH.57 This property can be used for chemodynamic therapy (CDT) to cause ICD, or regulate macrophage polarization,58 both of which could induce T cell penetration into tumor tissue. Nanocomposites can also be designed to degrade in response to H2O2, H+, GSH, etc. and release components to stimulate the immune responses.59
2.5. Synergistic properties
The organic and inorganic components of nanocomposites may promote each other to produce synergistic properties,60,61 which promote immune effects by enhanced antigen production or interactions between the material and immune cells.
Nanocomposites can improve tumor hypoxia through the optical, magnetic and chemical properties of the inorganic components. In addition to ICD, nanocomposites with photothermal or magnetic hyperthermia properties can also induce vascular injury to improve tumor oxygenation and vascular perfusion, thereby alleviating tumor hypoxia.62 Through the design of nanocomposites, H2O2 could be catalyzed to O2, which can enhance the efficiency of photodynamic therapy (PDT) restricted by hypoxia, thus realizing a synergistic effect. The improved ICD caused by PDT could in turn enhance the immune response. Moreover, the heat generated by the inorganic components could promote the release of the organic payload to increase antigen production,63 thereby improving the anti-tumor immune responses. Nanocomposites can also coordinate the biological targeting of the organic components with the magnetic targeting of the inorganic components.64 Magnetic targeting could increase the enrichment of nanocomposites in tumor tissues, while the organic components could promote their binding with T cells. Such synergistic effects could enhance the killing effect of T cells.
2.6. Size and shape effects
The size and shape of nanoparticles will affect the endocytosis, biological distribution, clearance rate and biocompatibility, which will further affect their biomedical applications in vivo.65 The size and shape of nanoparticles are demonstrated to have an effect on cytotoxicity and gene transfection efficiency.66–69 Moreover, the size, shape, and surface properties of nanoparticles affect the delivery of payloads to tumors or other target organs, which affects the uptake of subsequent antigen-presenting cells (APCs).70 Therefore, the activation of immune responses may be promoted by designing the size and shape of nanocomposites.
By adjusting the size of nanoparticles, the interaction between nanoparticles and immune cells can be adjusted to promote antigen presentation and immune cell activation.71–73 When the nanoparticle size is larger than 500 nm, it is more easily absorbed by tissue cells, reducing the interaction between materials and immune cells.74 When the size is smaller than 10 nm, it will quickly enter the circulatory system and be excluded from the body.75 Therefore, a size in the range of 10–500 nm may be a suitable range for nanomaterials to interact with the immune system. It is reported that 80 nm gold nanoparticles are moderately endocytosed by DCs, but the content is highest in lysosomes, which is conducive to their degradation and release of antigens. In this regard, maturation of DCs could be maximized and a strong T cell immune response could be induced.76 In addition, it is found that larger-sized nanoparticles can trigger a strong immune response.77 This is mainly due to the fact that small-sized nanoparticles (5–15 nm) are easily removed by lymph node follicles, while large-sized nanoparticles (50–100 nm) are easily retained in lymph nodes. The selective retention might induce stronger antigen stimulation to activate the immune responses.
In addition to the size, the shape of nanomaterials will affect the endocytosis efficiency. Nanorods are generally considered to have the highest absorption rate, followed by spherical, cylindrical, and cubic shapes.78 However, when examining the degree of activation of immune responses by four types of West Nile virus (WNV) protein/Au nanocomposites, it was found that although more rod-shaped nanocomposites were internalized by DCs, the immune response caused by spherical nanocomplexes was stronger. In addition to the endocytosis efficiency, the immune response is also related to inflammatory cytokines induced by different shapes of nanocomposites.79 The symmetry of nanocomposites also affects the immune responses. It was found that the performance of asymmetric nanocomposites is better than that of symmetric counterparts.80
Versatile organic/inorganic nanocomposites offer the possibility to realize a combination of functions, favorable properties, and reasonable design of size and shape. All these factors could be utilized to enhance the immune responses. It is also worth mentioning that combinatorial effects of these factors should also be comprehensively taken into account to evaluate the performance since the interaction of the nanocomposites with the immune system is complicated.81 We will introduce the various applications of nanocomposites in cancer immunotherapy according to the mechanism of immune responses triggered by nanocomposites.
3. Targeting the immune tumor microenvironment
The occurrence, growth and metastasis of tumors are closely related to the surrounding microenvironment.82,83 The immunosuppression within the TME evades monitoring of the host immune system, which leads to the failure of immunotherapy.84,85 The effectiveness of immunotherapy depends largely on whether it can overcome the obstacle of immune escape. Therefore, the regulation of the immunosuppressive TME is of great significance. The immunosuppressive TME is mainly composed of immunosuppressive cells such as myeloid-derived suppressor cells (MDSCs), tumor-associated macrophages (TAMs) and regulatory T cells (Tregs).86 MDSCs can directly differentiate into TAMs under hypoxic conditions.87 TAMs are the main types of inflammatory cells, which are associated with the progression of the disease and are one of the markers of poor prognosis.88 Macrophages play an important role in innate immunity and acquired immunity. As an important part of innate immunity, they can clear tumor cells and pathogens by phagocytosis, secreting cytokines and other bioactive molecules to modulate the immune TME. Furthermore, the modulated immune microenvironment could promote phagocytosis and presentation of antigens by macrophages, and finally realize the regulation of adaptive immunity.
However, macrophages in the immunosuppressive TME are the main factors that promote tumor progression, and are related to poor clinical prognosis. Based on the inflammatory roles in tumors, TAMs can be divided into pro-inflammatory macrophages (M1) and anti-inflammatory macrophages (M2). M1 macrophages can kill tumor cells and secrete a variety of pro-inflammatory cytokines (IL-γ, TNF-α, and IL-12). As immunomodulatory cells, M2 macrophages can secrete immunosuppressive cytokines (IL-10 and TGF-β), inhibit the activity of DCs and effector T cells, and finally terminate the anti-tumor immune response. Macrophages are susceptible to the immunosuppressive TME; M1 macrophages with killing effects often differentiate into immunosuppressive M2 cells, which will lead to the failure of tumor therapy.18 However, immunosuppressive M2 can also be reeducated to M1. To date, novel strategies for targeting TAMs have been developed.19,89 Herein we focus on the application of TAM-targeted organic/inorganic nanocomposites to modulate the immunosuppressive TME for improved cancer immunotherapy.
3.1. Composition and construction of nanocomposites targeting the tumor immune microenvironment
Organic/inorganic nanocomposites could modulate the immunosuppressive TME. In most cases, inorganic components of the nanocomposites play a major role, such as Fe3O4,49,90–92 MnO2,55,93,94 MoSe2,95,96 and Au.97 The synthesis of these nanoparticles is relatively simple and the yield is high, which has certain potential for large-scale preparation and promotion to clinical use. They could be synthesized through template,55,93 one-step reduction,94 chemical co-precipitation,49 hydrothermal,91,92 liquid exfoliation,95,96 or seed-mediated growth97 methods. However, the dispersibility of the as-prepared nanoparticles is poor. The combination with organic components, such as chitosan,95 PEG,92,93 polyvinyl pyrrolidone (PVP),96 carboxymethyldextran,90 and bovine serum albumin (BSA),97 could improve the stability and biocompatibility. In addition, organic molecules could introduce a targeting function to improve the accumulation of the nanocomposites at the tumor site, such as HA,49,94 mannose,94 targeting peptides,55,92etc. Moreover, other organic components such as chemical drugs,93,97 photosensitizers,93 and enzymes55 can also be included in organic/inorganic nanocomposites. The combination of these organic components and inorganic nanoparticles is mainly realized by electrostatic adsorption,55,94,95 ligand exchange,49,90,97 hydrophobic interaction,96,97 or conjugation through ester92,97 or amide bonds.93
Furthermore, some organic components may play a regulatory role in the immune microenvironment, such as HA,94 LOX,55 chemical drug Gem analogue gemcitabine monophosphate (GMP)98 and so on. They can regulate the polarization of macrophages by regulating the metabolism of lactic acid55 or directly acting on macrophages94,98 to achieve the modulation of the immunosuppressive TME. They are combined with inorganic nanomaterials through electrostatic adsorption55,94 or direct precipitation98 to realize increased delivery efficiency through active and passive targeting.55,94,98 Taking advantage of the responsive degradation characteristics of MnO255,94 and calcium phosphate98 nanoparticles in the TME, the resultant organic/inorganic nanocomposites could achieve efficient and controlled delivery. We believe that other properties of inorganic nanoparticles, including photothermal effects, magnetic targeting, and MRI, can also be used to give nanocomposite nanomaterials more functions with improved anti-tumor effectiveness.
Some organic/inorganic nanocomposites as a whole regulate the immune microenvironment, such as nanoparticles extracted from cuttlefish ink (CINP),99 and metal complex copper N-(2-hydroxy acetophenone)glycinate (CuNG).100,101 CINP is obtained by direct centrifugal extraction from cuttlefish ink; the main component is melanin, and in addition ∼20% polysaccharides and ∼1% Cu, Zn and other metals. These two materials can also repolarize macrophages from an immunosuppressed M2 phenotype to an immune-promoted M1 phenotype. We will review the immune effects and mechanisms mediated by organic/inorganic nanocomposites.
3.2. Iron oxide-based nanocomposites
Iron is an essential element of the human body with important physiological functions.102 Biological macromolecules such as hemoglobin, non-heme enzymes, cytochrome and myoglobin all contain iron. Meanwhile, since it is easy for iron to gain and lose electrons, iron ions can mediate the generation of superoxide anions and hydroxyl radicals. Iron may also have some toxicity to organisms. The Fenton reaction of iron with hydrogen peroxide leads to the formation of ROS and destroys biological macromolecules such as proteins, lipids and DNA.103
Iron oxide-based nanocomposites have been approved by the US FDA for the treatment of iron deficiency and are widely used as contrast agents or drug carriers in clinical or preclinical studies.104,105 In recent years, an FDA-approved iron oxide nanocomposite (ferumoxytol) has been found to inhibit the growth of tumors.90 Ferumoxytol is composed of an iron oxide nanoparticle core and a carboxymethyldextran coating.
The level of iron in tumor cells is higher than normal cells. Tumor cells are more likely to induce ROS with iron and this leads to tumor cell apoptosis. Then the apoptotic tumor cells can cause persistent polarization of macrophages from an M2 to an M1 phenotype.106 H. E. Daldrup-Link et al. found that ferumoxytol can induce the reeducation of TAM from an M2 to an M1 phenotype and increase lymphocyte infiltration in the tumor.90 They demonstrated that ferumoxytol can increase cancer cell cytotoxicity by up-regulating the production of macrophage ROS. Dead tumor cells can further promote the activation of macrophages and the production of ROS, maintain the sustained production of TNF-α and nitric oxide (NO), and finally realize continued M1 macrophage polarization.
For the better intracellular internalization of iron oxide-based nanocomposites, X. Z. Zhang et al. designed HA modified superparamagnetic iron oxide nanoparticles (HIONs) to artificially reprogram macrophages.49 The HA coating could enhance the macrophage internalization of iron oxide nanoparticles (IONPs) to increase the intracellular ROS level and inflammatory factors, which could effectively induce tumor cell apoptosis and educate M2 to M1 macrophages. For a better targeted-delivery efficiency, tumor targeted peptides can be used to functionalize nanoparticles. Short peptide CREKA (Cys–Arg–Glu–Lys–Ala) could target fibrin–fibronectin complexes on 4T1 breast tumor cells. H. M. Fan et al. designed an elaborate hybrid nanoparticle which could realize ICD of 4T1 cells by magnetothermodynamic (MTD) therapy. In addition, amplified ROS could be generated under an alternating magnetic field, which effectively induced calreticulin (CRT) exposure and macrophage polarization to pro-inflammatory M1 phenotypes.92 Short peptide CREKA was chosen as a ligand to form nanocomposites to target the tumor more efficiently and could significantly improve tumor inhibition.
Besides ROS, iron also participates in the polarization of M1 macrophages through other pathways such as the interferon regulatory factor 5 (IFR5) signal pathway and NF-κB signal pathway. TNF receptor associated factor 6 (TRAF6) is upstream of IRF5 activation. It has been proved that iron mediates the activation of IRF5 by participating in the ubiquitination of TRAF6. IRF5 further participates in the TLR-MyD88 signal pathway, and finally promotes M1 macrophage polarization by inducing the expression of proinflammatory cytokines (IL-6, IL-12, and TNF-α).107,108 In addition, the crystallinity of IONPs could also influence the activation of the IFR5 signal pathway. C. Z. Yu et al. designed magnetite and hematite IONPs with similar size, morphology, and surface properties to study the mechanism of iron oxide-induced macrophage activation (Fig. 3).91 Compared with hematite IONPs, magnetite IONPs are more effective in M1 polarization and inhibition of tumor growth. Magnetite IONPs specifically rely on the IFR5 signal pathway to achieve M1 polarization and down-regulate M2-related arginase-1.
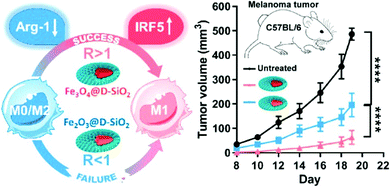 |
| Fig. 3 Mechanism of M1 activation induced by magnetite and hematite INOPs with different crystallinity. Reproduced with permission from ref. 91. Copyright 2019, American Chemical Society. | |
3.3. Manganese dioxide-based nanocomposites
MnO2-Based organic/inorganic nanocomposites have been widely used as responsive materials for the acidic TME. Studies have shown that MnO2 can be degraded by reacting with H+ or GSH in the TME, and can catalyze the decomposition of H2O2 into H2O and O2, and thus relieve hypoxia.109–111 The hypoxic TME can promote the infiltration of Tregs and transform the TAMs into immunosuppressive M2 macrophages.112,113 Therefore, relieving tumor hypoxia can promote the reversal of the immunosuppressive TME. MnO2 can be degraded in vivo and then excreted through the kidneys with low risk of accumulation in vivo, which is a promising material targeting the immunosuppressive TME.
Z. Liu et al. designed biodegradable MnO2/PEG nanocomposites for TME-targeted combination therapy.93 The nanocomposites were reported to respond to the low acidic TME, produce oxygen to relieve hypoxia, promote the repolarization from M2 to M1, upregulate the secretion of IL-12, and increase the infiltration of cytotoxic T lymphocytes (CTLs) in the tumor.
The macrophage mannose receptor is highly expressed on M2-like macrophages, and mannose-modified nanoparticles can effectively target M2-like macrophages.94,114 X. Y. Chen et al. effectively targeted the high accumulation of TAMs using mannose and HA modified manganese dioxide nanoparticles to relieve hypoxia. In their study, mannose/MnO2 nanocomposites could target M2 macrophages, which effectively alleviate the hypoxia in the tumor sites. HA can further polarize the anti-inflammatory, pro-tumoral M2 macrophages into pro-inflammatory, anti-tumor M1 macrophages.94
In addition to hypoxia, the accumulation of a metabolite, lactic acid, is also a feature of the immunosuppressive TME. It is supposed that lactic acid can promote M2 macrophage polarization by maintaining the expression of hypoxia-inducible factor-1 α (HIF1-α). X. Z. Zhang et al. designed red blood cell membrane (mRBC)-coated hollow MnO2 nanoparticles embedded with LOX and a glycolysis inhibitor (denoted as PMLR nanocomposites) (Fig. 4a).55 The mRBC helps nanocomposites avoid the clearance of macrophage phagocytosis through CD47 (Fig. 4b). In tumor cells, the acidic pH and endogenous H2O2 decompose MnO2 and release LOX to catalyze the oxidation of lactic acid (Fig. 4c and d). The resultant H2O2 could then be catalyzed by MnO2 to produce O2 and further promote the oxidation of lactic acid by LOX. At the same time, the released glycolysis inhibitor could stop the production of lactic acid by inhibiting glycolysis. The PMLR nanocomposites were found to activate the macrophages by lactic acid exhaustion through the toll-like receptor (TLR) signaling pathway and NF-κB signaling pathway. Hollow MnO2 nanoparticles could further amplify the lactic acid exhausting function of the nanocomposites by alleviating tumor hypoxia.
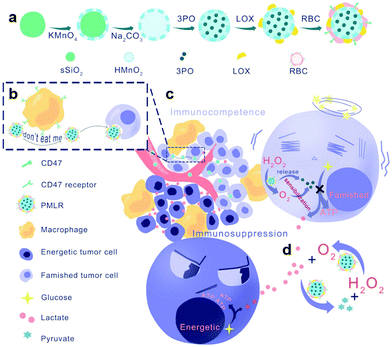 |
| Fig. 4 Schematic illustration of the (a) preparation steps, (b) avoidance of macrophage phagocytosis, (c) intra/extracellular lactic acid exhaustion process and (d) cascade catalysis process of PMLR nanocomposites. Reproduced with permission from ref. 55. Copyright 2019, Wiley-VCH. | |
3.4. Other material-based nanocomposites
Several studies have reported that thermal effects induced by nanoomposites could modulate the immunosuppressive TME. Nanocomposites that could be applied for photothermal therapy (PTT) can up-regulate the expression of cytokines such as IFN-γ or TNF-α, or down-regulate the expression of CD206 receptor or Arg-1 to mediate the polarization of macrophages to M1 type. PTT mediated by mRBC coated MoSe2 nanocomposites (mRBC/MoSe2) could significantly decrease the mRNA level of Arg1 and CD206, and enhance the mRNA level of TNF-α, suggesting TAM reprogramming from the M2 to the tumoricidal M1 phenotype.95 In the study, the release of tumor antigens induced by the photothermal effect activated CTLs and up-regulated the secretion of IFN-γ, and thus promoted the transformation of M2 macrophages to M1 macrophages (Fig. 5). In addition, BSA/Au nanocomposites were reported to downregulate the expression of CD206 and legumain after photothermal treatment.97 The photothermal effect of Au nanorods in the nanocomposites can directly inhibit the polarization of TAMs to M2 macrophages. X. Z. Zhang et al. extracted natural melanin nanoparticles from cuttlefish, which can effectively induce the polarization of M1 macrophages by activating the mitogen-activated protein kinase signal pathway.99 Copper chelate also has the potential to reeducate macrophages from the M2 phenotype to the M1 phenotype. S. K. Choudhuri et al. reported that CuNG could reprogram TAMs to the pro-immunogenic phenotype leading to an anti-tumor immune response.100,101
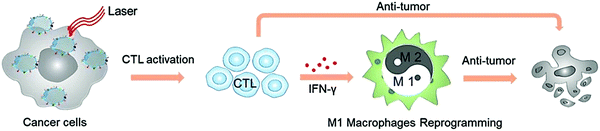 |
| Fig. 5 Schematic illustration of TAM reprogramming to the tumoricidal M1 phenotype mediated by mRBC/MoSe2-mediated PPT to potentiate the antitumor effect. Reproduced with permission from ref. 95. Copyright 2019, Wiley-VCH. | |
TAMs can be differentiated from MDSCs,87 which also play a negative role in regulating immune activation in the immunosuppressive TME.115 MDSCs can inhibit the anti-tumor response by interacting with other immune cells, thus promoting tumor growth and immune escape. In the TME, they can differentiate into TAMs, Tregs and other immunosuppressive cells.87 Therefore, targeting MDSCs can alleviate tumor immunosuppression and improve anti-tumor activity. The common drugs targeting MDSCs are Gemcitabine (Gem), 5-fluorouracil (5-FU), cyclophosphamide and paclitaxel.115 J. A. Chen et al. realized high performance Gem delivery in the TME by formulate GMP into a lipid-coated calcium phosphate (LCP) nanoparticle. The resultant LCP-GMP nanocomposites can significantly mediate the down regulation of p-STAT3 in tumors and then suppress the MDSCs in the TME. They could induce the apoptosis of tumor cells and the transformation from M2 macrophages to M1 macrophages, and further restore CD8+ T-cell-mediated anti-tumor immune responses.98
Scavenger receptor type B-1 (SCARB1) is highly expressed on MDSCs. Spherical high-density lipoproteins (HDLs) rich in apolipoprotein A-I (apo A-I) can bind to this receptor with high affinity. At the same time, some studies have proved that apo A-I has anti-tumor effects in a mouse model. C. S. Thaxton et al. obtained high density HDL/Au nanocomposites116 which are similar to natural HDL particles in size, shape, surface charge and surface composition. The HDL/Au nanocomposites could target MDSCs by specifically binding to SCARB-1 receptors. In the metastatic TME, the HDL/Au nanocomposites could increase the inflammation of CD8+ T cells and reduce the ratio of suppressive Tregs.
All-trans retinoic acid (ATRA) can promote the differentiation of MDSCs into mature DCs, macrophages and granulocytes to regulate the immunosuppressive TME and enhance the anti-tumor immune response. Z. P. Zhang et al. realized chemo-immunotherapy by using biodegradable hollow mesoporous silica nanoparticles (DHMSN) to co-deliver ATRA, doxorubicin (DOX) and IL-2.117 ATRA could significantly reduce the number of MDSCs in tumors, and the combined effect of ATRA and DOX could increase the number and promote the maturation of DCs. The combined use of IL-2 further enhanced the proliferation of T lymphocytes. The resultant A/D/I-dHMLB nanocomposites could realize effective combination of tumor cell immunogenic death, regulation of the immune microenvironment and promotion of the T lymphocyte response, resulting in a synergistic anti-tumor effect.
4. Activating antitumor immune responses
Activating antitumor immune responses, especially the T cell-mediated immune responses, is one of the effective strategies for cancer immunotherapy.4,118 Due to the immunosuppressive TME, the body is often unable to successfully initiate anti-tumor immune responses.119 According to the biological characteristics of the immune response, the establishment of an effective anti-tumor immune response usually requires the release of tumor antigens, activation of the innate immune system, maturation of APCs, the activation and proliferation of tumor-specific T lymphocytes, T cell infiltration in the tumor site and specific recognition of tumor cells. Through these steps, the body can establish effective anti-tumor immune responses and then inhibit the growth of tumors.120,121
Traditionally, immune activation is mainly achieved through the use of immune adjuvants and tumor therapeutic vaccines.122 Traditional biological adjuvants (such as CpG and poly(I:C)) and tumor vaccines (protein vaccines, peptide vaccines, and nucleic acid vaccines) cannot induce effective immune responses due to their low immunogenicity and easy degradation.123,124 Studies have shown that nanoparticles can stimulate the innate immune responses and activate APCs to promote antigen presentation.125 Moreover, they could effectively protect antigens and adjuvants from degradation as carriers to enhance targeted delivery to APCs and the antitumor immune responses. In this chapter, the application of organic/inorganic nanocomposites in antitumor immune response activation is discussed through different activating routes.
4.1. Inducing immunogenic cell death
ICD mainly refers to a kind of cell death modality that stimulates an immune response against dead-cell related antigens. Usually, the identification of ICD is mainly based on the release of damage-associated molecular patterns (DAMPs), such as surface-exposed CRT, passively released high mobility group protein B1 (HMGB1) and secreted adenosine triphosphate (ATP).126,127 ATP facilitates the recruitment of DCs into the tumor. CTR and HMGB1 stimulate the uptake, processing and presentation of antigens by DCs. Altogether, the anti-tumor immune responses could be finally initiated, resulting in a tumor killing effect.128 Various therapies for tumors such as chemotherapy, radiotherapy, CDT and PDT have been reported to have the ability to induce ICD.10 ICD can be induced by cell death signals triggered by non-endoplasmic reticulum (ER) associated targets.129 Herein, we mainly focus on the organic/inorganic nanocomposites that can activate the immune system by tumor antigens by ICD.
4.1.1. ICD induced by ROS.
ROS-based ER stress can elicit ICD with high immunogenicity. PDT is a promising strategy for tumor treatment which has been approved by the FDA for clinical treatment.130 For PDT, tumors are killed by photoactivating exogenous ROS or singlet oxygen (1O2) produced by photosensitizers.131 PDT can induce ICD and tumor antigen release. The most prominent feature of ICD is the release of DAMP signals. The DAMP signals further lead to the activation of APCs and promote the presentation of tumor-associated antigens by ICD, which will promote the immune response.128,132 Z. F. Liu et al. designed tumor integrin αvβ6-targeting peptide functionalized graphene oxide (GO) to deliver a photosensitizer, photochlor(2-[1hexyloxyethyl]-2-devinyl pyro pheophorbide-alpha, HPPH), to tumor cells for PDT.133 ICD induced by PDT was found to significantly stimulate DC maturation, increase the infiltration of CTLs, and finally effectively inhibit the growth and metastasis of the tumor.
The porous nature of nanoscale metal–organic frameworks (nMOFs) facilitates high loading efficiency of photosensitizers and the diffusion of ROS in tumor cells.134 W. Lin et al. designed Hf-porphyrin and chlorin-based nMOFs for improved PDT,135,136–138 leading to surface CRT expression on tumor cells. The exposed CRT would serve as a signal to activate APCs and induce a tumor specific T cell response. Indoleamine 2,3-dioxygenase (IDO) is an immunoregulatory enzyme highly expressed in tumors that prevents the activation of T cells and promotion of T cell energy and apoptosis. For further enhancement in PDT, they combined PDT with an IDO inhibitor and achieved effective local and distant tumor eradication in colorectal cancer models. Hypoxia is common in tumors and will impair the effect of PDT.139 In order to overcome tumor hypoxia and enhance cancer immunotherapy induced by PDT, W. Lin et al. designed Fe-nMOFs composed of Fe3O and porphyrin (Fig. 6).138 When irradiated under hypoxic conditions, the Fe-nMOFs catalyzed the decomposition of intracellular H2O2 into O2, and then O2 was converted to cytotoxic 1O2 by photoexcited porphyrins. It was proved that Fe-nMOFs could induce higher levels of CRT exposure, indicating a stronger ICD induction ability.
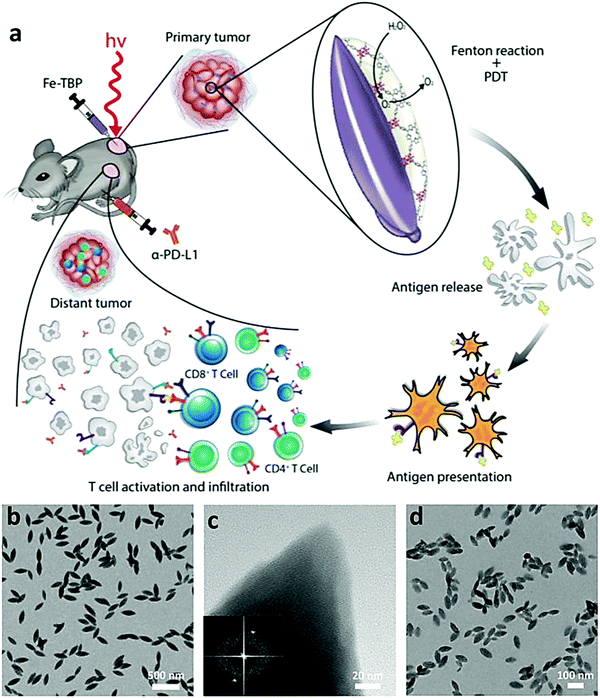 |
| Fig. 6 (a) Schematic illustration of Fe-nMOFs to overcome hypoxia for improved PDT and cancer immunotherapy. Transmission electron microscopy (TEM) images (b and d) and high resolution TEM image (c) of Fe-nMOFs. Inset in (c): fast Fourier transform of Fe-nMOFs. Reproduced with permission from ref. 138. Copyright 2018, American Chemical Society. | |
In addition to PDT, ICD can also be induced by ROS produced by CDT. In the presence of Fe2+, H2O2 can be decomposed into highly active hydroxyl radicals in a pH-dependent way, which drives the production of tumor-specific ROS. J. L. Shi et al. reported that amorphous iron nanoparticle-based composites could induce a Fenton reaction in the TME.140 Copper-based nanocomposites also possess the ability to induce a Fenton reaction.141,142 M. Y. Gao et al. prepared nanocomposites employing polyethylene glycol modified Cu2−xSe nanoparticles, β-cyclodextrin and chloride e6 (Ce6).143 Cu+ and Ce6 can decompose H2O2 in tumors and produce a large amount of ROS to induce the production of ICD. The nanocomposites were found to enhance M1 macrophage polarization, effectively kill tumor cells, induce anti-tumor immunity, and inhibit tumor metastasis and recurrence.
4.1.2. ICD induced by hyperthermia.
PTT is a promising strategy for local thermal ablation of tumors by converting the energy of incident light into heat. In the clinic, systemic or local hyperthermia can enhance the sensitivity of traditional chemotherapy and radiotherapy. Meanwhile, it has been shown that high temperature can affect the immune response, stimulate the maturation and migration of DCs, enhance antigen presentation and initiate a T cell immune response. The mechanism of the effect of hyperthermia on immune responses is still not clear.130 Studies have shown that high temperature can up-regulate the expression of heat shock proteins (HSPs).144 HSPs, especially HSP70, as an endogenous danger signal of the immune system, can activate innate and acquired immune responses. At the same time, HSP70 can also stimulate the maturation of APCs, enhance the expression of major histocompatibility complex (MHC)-I molecules on the surface of tumor cells, and then improve the intensity of immune responses.145 Z. Zhang et al. developed a kind of storage framework composed of graphene oxide-based nanocomposites.146 After irradiation with an 808 nm laser, the increase of HSP70 expression can stimulate the maturation of DCs. For in vivo PTT, DC maturation (CD86+CD11c+) increased slightly, and Tregs (CD4+Foxp3+) increased significantly, while CTLs (CD3+CD8+) decreased significantly. Paclitaxel can effectively block immune escape by eliminating Foxp3+ T cells and promoting the maturation of DCs. When PTT was combined with paclitaxel, a synergistic effect with intensified antitumor immunological efficacy was observed.
The main feature of ICD is the change of DAMP markers, including but not limited to the exposure of CRT, and the release of HMGB1 and ATP. ICD could be confirmed when these three markers could be detected at the same time. In order to clarify the mechanism of ICD caused by PTT, R. Fernandes et al. verified the effect of temperature on tumor cell ICD by changing the Prussian blue nanoparticle concentration and laser power (Fig. 7).147 The results showed that the viability of neuroblastoma cells (Neuro2a cells) decreased when the temperature was higher than 48 °C. The 50.7 °C group and 61.1 °C group exhibited all three markers. Higher temperature (84.3 °C) only showed the exposure of CRT and the release of ATP, so higher temperature may not induce effective ICD. In vivo immunotherapy results showed that there was an optimal temperature window of ICD (63.3–66.4 °C). Therefore, a tunable immune response to heat could be realized to maximize the therapeutic effectiveness.
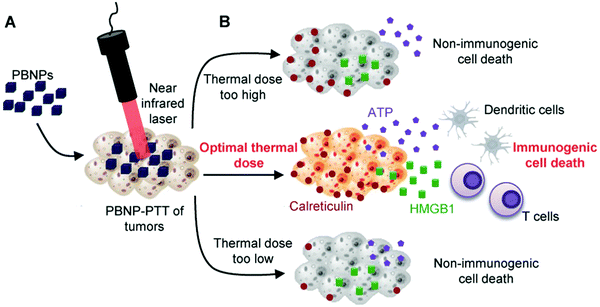 |
| Fig. 7 Optimal thermal window of ICD generated by Prussian blue-based PTT. Reproduced with permission from ref. 147. Copyright 2018, Wiley-VCH. | |
4.1.3. ICD induced by a combination of ROS and hyperthermia.
Hyperthermia can induce vascular blood flow in the tumor site to improve hypoxia and enhance the therapeutic effect of PDT.148,149 In addition, while a higher PTT treatment temperature cannot successfully induce ICD of tumor cells, PDT can induce efficient ICD through the production of ROS.147 Therefore, the combination of ROS and hyperthermia could synergistically enhance the antitumor effect. W. F. Dong et al. developed a kind of Janus organosilica-iron oxide/Ce6 nanocomposites to induce ICD by PDT and magnetic hyperthermia.150 They found that PDT and magnetic hyperthermia have a synergistic enhancement effect on the induction of ICD. In vivo antitumor experiments showed that the nanocomposites under a laser and an alternating magnetic field partially reduced the number of metastatic foci in the lungs, increased the secretion of TNF-α, IFN-γ and IL-6, and increased intratumoral infiltration of CTLs.
In order to further improve the depth of tissue penetration and therapeutic effect of PDT, J. Lin et al. designed PVP/Cu2MoS4–Au nanocomposites,151 which show the enhancement of absorption in the NIR region, and better photothermal conversion efficiency and ROS production. Cu2MoS4/Au could also catalyze the decomposition of H2O2 into O2 in the TME, which could significantly improve the hypoxia to improve the therapeutic effect of PDT. Therefore, the PVP/Cu2MoS4–Au nanocomposites could release tumor-associated antigens through ICD, activate DCs, promote the secretion of cytokines, induce a strong T cell immune response and prevent tumor metastasis.
4.1.4. ICD induced by an imbalance of osmotic pressure.
An imbalance of osmotic pressure in the cells has also been reported to induce ICD.152 J. Xie et al. innovatively synthesized PEGylated sodium chloride nanoparticles (PEG/NaCl) through a microemulsion reaction (Fig. 8a).152 The degradation of PEG/NaCl nanocomposites can cause an imbalance of osmotic pressure in the cells and the decrease of cell viability (Fig. 8b). The released cathepsin B induced formation of the NLRP3 inflammatory body, and mediated pyroptosis by the caspase-1 pathway, and finally leads to ICD. The nanocomposite treated tumor cells showed high-efficiency CRT protein exposure and significantly increased ATP and HMGB-1 secretion (Fig. 8c). In vivo antitumor results demonstrated significant inhibition of tumor growth in the group of the nanocomposites (Fig. 8d).
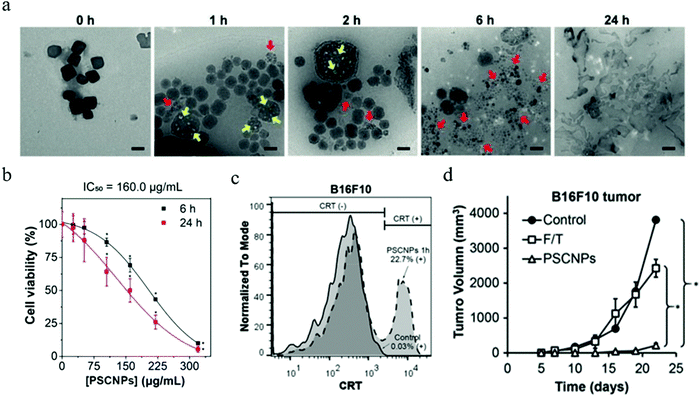 |
| Fig. 8 (a) TEM images of NaCl nanoparticles and their degradation in water over time. (b) Cell viability of PC-3 cells after 6 and 24 h incubation with NaCl nanoparticles at a concentration from 26.3 to 320 μg mL−1. (c) CRT presentation on dying B16F10 cells. (d) In vivo tumor growth inhibition of B16F10 tumors. Reproduced with permission from ref. 152. Copyright 2019, Wiley-VCH. | |
4.2. Delivery of Toll-like receptor agonist
TLR is an important part of the mammalian immune system, which plays a role by recognizing pathogen-related molecular patterns (PAMPs), connecting innate immunity and acquired immunity, and TLR serves as one of the earliest determinants of immune activation.153 TLRs have become important therapeutic targets for the treatment of infectious diseases, cancer and allergies. Many TLR agonists are currently in clinical trials or approved as immune stimulants. TLR3, 4, 7/8 and 9 agonists represent promising immunotherapy for cancer and have been included in the US National Cancer Institute's list of the most promising immunotherapeutic agents for cancer.154 The maturation of DCs is a prerequisite for initiating an antigen-specific immune response. TLR-mediated DC activation leads to enhanced phagocytosis, up-regulated expression of MHC and costimulatory molecules (CD80, CD86 and CD40), up-regulated expression of CC-chemokine receptor 7 (CCR7), migration to draining lymph nodes, secretion of cytokines and antigen presentation to lymphocytes. The activation of TLR3, 4, 7/8 and 9 receptors can significantly up-regulate the secretion of type I interferon, which is not only related to antiviral defense, but also enhances the adaptive immune response by promoting cross-presentation of antigens, promoting T cell proliferation, preventing T cell apoptosis, inducing DC maturation and activating NK cells.155
CpG oligodeoxynucleotides (CpG ODNs) are a TLR9 agonist that simulates the pathway of innate immunity activated by bacterial DNA.156 Unmethylated CG dinucleotides can be found in prokaryote DNA with high frequency, but are rare in eukaryotic DNA. When bacterial infection occurs, unmethylated CpG motifs (consisting of a central unmethylated CG dinucleotide plus flanking regions) in bacterial DNA can activate the TLR9 pathway, and initiate a protective immune response against bacteria.157 CpG ODNs serve as a TLR9 agonist by mimicking the immunostimulatory activity of bacterial DNA. Y. Gao et al. constructed nanocomposites by loading CpG ODN agonists onto H3R6 polypeptide conjugated multiwalled carbon nanotubes (MHR-CpG) for immunotherapy of prostate cancer.158 MHR could efficiently deliver CpG and selectively accumulate in the tumor site and tumor-draining lymph nodes, and could specifically target the TLR9 receptor, increase the proportion of CD4+ and CD8+ T cells in the spleen, and up-regulate the expression of TNF-α and IL-6. The overall tumor inhibition rate of the MHR-CpG nanocomposite group was significantly higher than that of other groups.
PTT can lead to the death of tumor cells and antigen release, while CpG can further promote the maturation of DCs, and promote the presentation of released tumor antigens.156 N. F. Zheng et al. used palladium nanosheets to deliver CpG (PDNCs), achieving the combination of PTT and CpG immunotherapy.159 CpG combined with PTT can increase the infiltration of CD8+ cells in the tumor, and promote the activation of CTLs and secretion of IFN-γ, and finally induce a strong antitumor immune response, which can significantly control tumor growth and improve the survival rate of mice.
TLR7/8 can recognize single strand RNA and initiate an immune response.160 D. A. Mitchell et al. designed organic/inorganic nanocomposites (RNA/IO) based on mRNA encoding tumor antigen-loaded cationic nanoliposomes and IONPs (Fig. 9).161 RNA/IO nanocomposites can effectively activate the immune response through the TLR7 pathway. RNA/IO could significantly change the RNA expression profile of DCs and up-regulate the expression of genes related to viral defense including type I interferon production, TLR signaling, the innate immune response, lymphocyte migration (CCL3 and CCL4) and T-cell-activating cytokine IL-12. The introduction of IONPs can not only enhance the RNA transfection of DCs, but also serve as a tracer of DC migration through MRI. They found that the T2-weighted MRI intensity in the lymph nodes was associated with the transport of DCs and could be used as an early warning indicator of the intensity of the anti-tumor response.
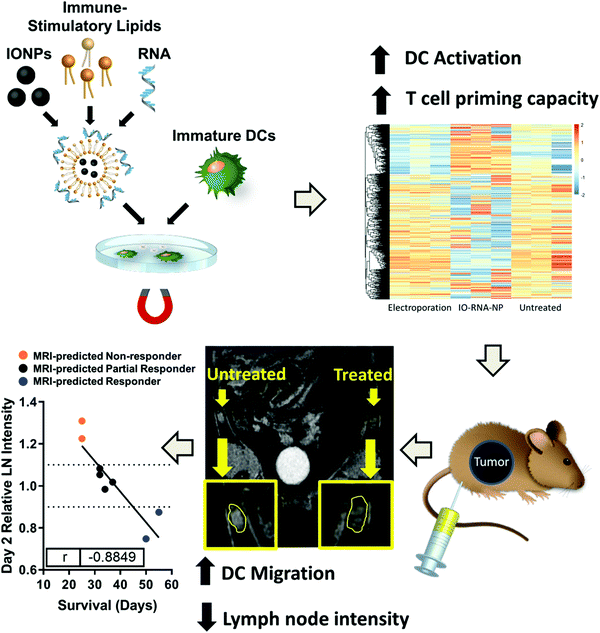 |
| Fig. 9 Schematic illustration of incubation of RNA/IO nanocomposites with DCs in the presence of a magnetic field to stimulate antigen-specific T-cells. Reproduced with permission from ref. 161. Copyright 2019, American Chemical Society. | |
4.3. Delivery of antigens
Antitumor immune responses mainly depend on a T cell-mediated cellular immune response to eliminate tumors.8 Tumor vaccines were designed to elicit a tumor specific T cell response in the body, and this has been seen as the main strategy for cancer immunotherapy. In the development of tumor vaccines, four key factors determine whether the vaccine works, including tumor antigens, immune adjuvants, delivery vehicles and formulations.162 Among them, the premise of successful preparation of a tumor vaccine is to select ideal target antigens. The most common antigens used in tumor vaccines are tumor-associated antigens and tumor-specific antigens. Tumor-associated antigens include antigens that are overexpressed in tumor cells or involved in tumor cell differentiation and are not expressed in normal tissues. The common ones are human epidermal growth factor receptor 2 and human telomere reverse transcriptase, mammaglobin-A, prostate-specific antigen, melanoma antigen recognized by T cells and so on. Tumor specific antigens refer to the antigens specifically expressed in tumor cells, which are foreign antigens and not controlled by central tolerance. Both chicken egg ovalbumin (OVA) and tumor cell membranes can be used as antigens to activate immune responses. As the most commonly used model antigen, OVA is easy to obtain and the composition of the antigen is single. For relatively complete cell lines expressing OVA antigen, it can be used to establish tumor models such as the B16-OVA,163 E.G7-OVA,164 and LLC-OVA165 models. The composition of tumor cell membrane antigens is complicated, and contains almost all the antigens needed to activate the immune responses. In addition, the combination with tumor cell membranes could increase the biocompatibility of nanocomposites and endow tumor targeting.166,167
When the antigen enters the body, it is first phagocytized, processed and presented through DCs. The entry of antigens into DCs and effective processing and presentation is the first step in initiating an anti-tumor immune response.168 Nanocarriers have been widely used in the delivery of tumor antigens. They provide protection for tumor antigens and prevent antigens from being degraded by biological enzymes in the circulatory system or tumor tissues.169 Compared with soluble antigens, nanoparticles are more easily ingested and captured by APCs.79,170 In addition, better targeting can be achieved by modifying nanoparticles.171
Due to the advantages of high accessible surface areas and high payload, mesoporous hollow materials with high radial dendritic pore structure have attracted much attention recently.172 C. Z. Yu et al. report the synthesis of shell number controllable, dendritic mesoporous organosilica hollow spheres.173 The model antigen OVA and B16F10 tumor cell fragments were used to evaluate their potential in cancer immunotherapy. It was found that the double-shelled nanocomposite induced a stronger antitumor immune response than the one-shelled counterparts. J. Lin et al. developed large-pore mesoporous-silica-coated upconversion nanoparticles to load OVA or tumor cell fragments and the photosensitizer merocyanine 540 and this acted as a novel strategy for cancer photodynamic immunotherapy.174 The results showed that the nanocomposites played a synergistic role in stimulating an immune response through PDT. H. A. Santos et al. designed thermally oxidized porous silicon nanoparticles encapsulated into acetalated dextran polymeric particles. The particles were then co-extruded together with cancer cell membrane vesicles to formulate the final core–shell nanocomposites.175 They found that the nanocomposites could significantly promote the expression of CD86 and CD80, which could activate a T cell response toward a Th1 cell mediated response.
IONPs can induce the polarization of macrophages from the M2 phenotype to the M1 phenotype, which has been discussed in the previous chapter.49,90–92 Aminosilane-coated superparamagnetic IONPs have already been approved by the US FDA and the European Union.90 A. G. Wu et al. used amphiphilic polymer-coated IONPs to react with tumor model antigen OVA by covalent bonding (Fig. 10).176 IONP-OVA nanocomposites could stimulate bone marrow stromal cells to secrete IFN-γ and TNF-α and activate macrophages. They could further stimulate the maturation of DCs and activate a T cell immune response effectively. This can not only inhibit the growth of B16-OVA tumors, but also prevent the formation of subcutaneous tumors and metastatic tumors. Their studies have proved that IONPs can play the dual role of carriers and immune adjuvants cooperating with antigens to activate the immune responses.
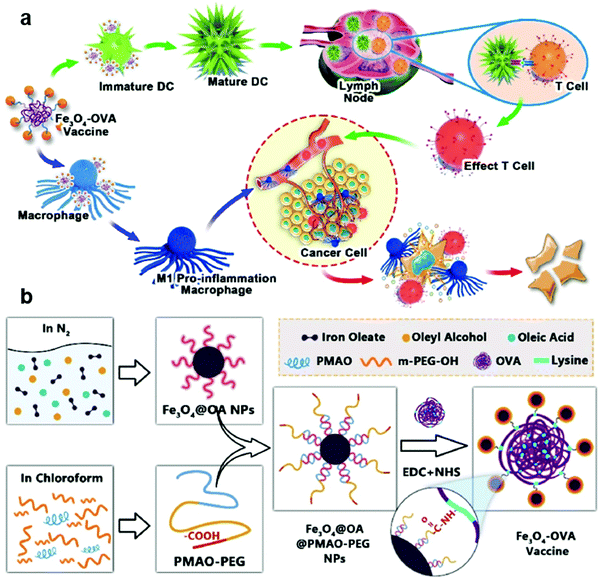 |
| Fig. 10 Schematic illustration of (a) the IONP-OVA vaccine strategy and (b) the synthesis of the IONP-OVA vaccine. Reproduced with permission from ref. 176. Copyright 2019, Elsevier Ltd. | |
In addition to the effective delivery of antigens, APCs are also very important for the processing and presentation of antigens. APCs such as DCs initiate a specific T-cell immune response through antigen uptake, processing and presentation.177 Therefore, the antigen presentation efficiency of DCs may also affect the effect of cancer immunotherapy.178 As the cell component carrying tumor antigens, the tumor cell membrane has been used in the development of tumor vaccines.167,179 In order to improve the efficiency of DCs in antigen presentation, L. F. Zhang et al. prepared a nanoparticle vaccine (BM-Au) by coating AuNPs with bacterial outer membrane vesicles (OMVs) derived from Escherichia coli.180 The data showed that, compared with OMVs, the BM-Au nanocomposites could effectively induce the activation and maturation of DCs in mouse lymph nodes. The tumor cell membrane was also utilized to promote the immune response of the body. Z. P. Zhang et al. synthesized gold nanoparticles using B16-F10 cells, and the cells secreted Au nanoparticles (AuNP@B16F10) coated with a tumor cell membrane (tumor antigens) (Fig. 11).181 Then AuNP@B16F10 is introduced into DCs, and worked as a tumor vaccine through the combination of PTT and immunotherapy. The resultant AuNP@DCB16F10 nanocomposites could significantly increase the proportion of CD3+CD8+ T lymphocytes in distal tumors and promote the secretion of IFN-γ and IL-6, and TNF-α, among which the immune response was stronger when combined with PTT. X. Z. Zhang et al. fused DCs with a tumor cell membrane to share the cytoplasm while having two independent nuclei (Fig. 12).182 They then used the bioreprogrammed fusion cell membrane to coat MOF nanoparticles and prepare a tumor vaccine (NP@FM). The vaccine contains both tumor antigens and MHC-1 molecules for antigen presentation. The fused cell membrane has a stronger ability to activate T cells to transform into CTLs, since NP@FM could directly present antigens to T cells and activate T cells. They found that the activation of DCs was mainly through these pathways of cytokine–cytokine receptor interactions, the chemokine signaling pathway, and the TNF signaling pathway. The imaging function of the MOF confirmed that NP@FM nanocomposites could significantly promote the lymphatic migration and homing ability of mature DCs.
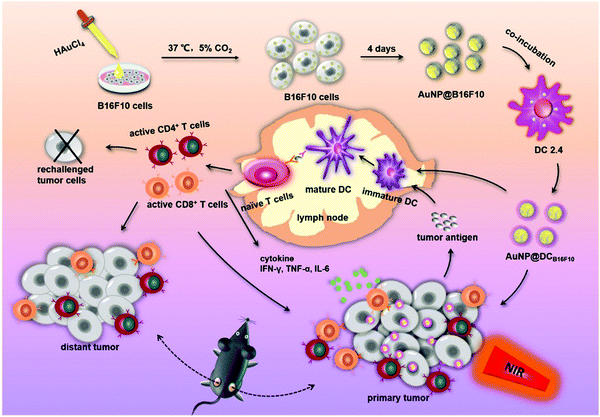 |
| Fig. 11 Schematic preparation of AuNP@DCB16F10 and mechanism of the AuNP@DCB16F10-mediated combinational treatment modality. Reproduced with permission from ref. 181. Copyright 2019, American Chemical Society. | |
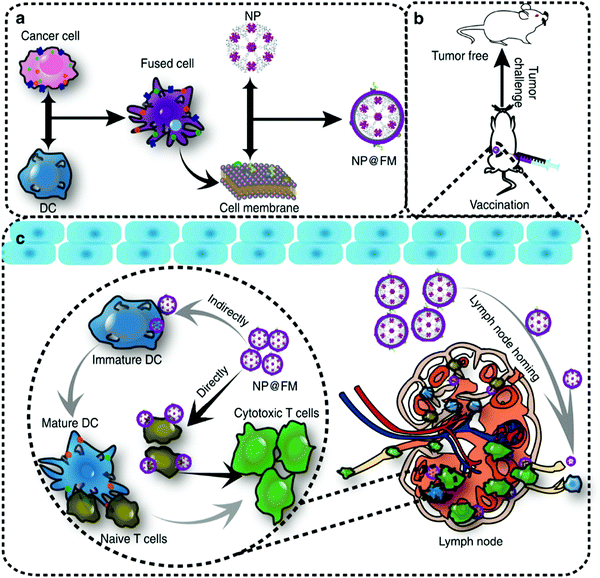 |
| Fig. 12 Schematic illustration of (a) preparation of NP@FM. (b) Vaccination of NP@FM for tumor prevention and (c) mechanisms of MOF@FM inducing immune responses. Reproduced with permission from ref. 182. Copyright 2019, Springer Nature. | |
4.4. Stimulating T cells
T cells play an important role in cancer immunotherapy. Although T cell activation is generally mediated by APCs in vivo, T cell activation can also be achieved by introducing T cell stimulators, in vitro antigen stimulation, artificial modification and so on. In the TME, tumors can escape the tumor killing effect of the immune system through a variety of ways. T cells may exist in the form of anergic T cells, exhausted T cells, and senescent T cells.183 In order to activate T cells for anti-tumor immunity, L. Yin et al. used a zeolitic imidazolate framework (ZIF-8) to carry chemotherapeutic drug DOX and immunomodulator Avasimibe for combined chemotherapy and immunotherapy.184 Avasimibe is an inhibitor of ACAT (a key enzyme of cholesterol esterification in CD8+ T cells), which can enhance its tumor killing effect by changing the metabolism of T cells. The resultant nanocomposites could not only kill tumor cells by DOX, but also improve the tumor killing activity of CTLs, and exert a synergistic antitumor effect of chemotherapy and immunotherapy.
Due to the existence of the immunosuppressive TME, T cells in vivo often cannot respond effectively. In order to activate the T cell response and exert its antitumor effect, it is an effective strategy to activate immune cells in vitro and then inject them in vivo. As early as 60 years ago, adoptive lymphocyte transfer has been used to effectively target tumors in mouse models.185 The emergence of genetic engineering technology has brought great progress to the activation of T cells in vitro.186 CAR-T therapy is through the introduction of antigen receptor genes into T cells cultured in vitro, and then through rapid expansion and activation to obtain a large number of effector T cells, which can have a powerful anti-tumor effect after being introduced into patients.187 Because CAR-T depends on the genetic modification and culture of T cells in vitro, the process of processing and manufacturing CAR-T is crucial to the success of the treatment strategy.
J. P. Spatz et al. prepared an anti CD3 antibody modified quasi-hexagonal array of gold nanoparticles combined with a cross-linked integrin-bound polyethylene glycol (PEG) hydrogel to expand T cells in vitro.188 Then, their group developed a new way to activate and prepare a large number of T cells in vitro.189 They prepared the nanostructured surface composed of quasi-hexagonal ordered gold nanoparticles on the surface of TiO2 by the block copolymer micellar lithography method. The antibodies of anti CD3 and anti CD28 were used as costimulatory signals of T cell activation, and the surface of TiO2 was modified with arginine–glycine–aspartic acid (RGD) cell adhesion peptide to promote cell adhesion. The nanocomposites were found to activate primary human CD4+ T cells in vitro, which is an effective alternative to the preparation of activated T cells in vitro. M. J. Butte et al. used superparamagnetic IONPs and alginate to prepare microbeads and modified the surface of microbeads with anti CD3 and anti CD28 antibodies to prepare artificial antigen presenting cells (aAPCs).190 They confirmed that oscillatory force enhanced engineered aAPCs can provide stronger antigenic signals for T cell activation in vitro than traditional dynaband cultures.
The US FDA has approved CAR-T therapy for treatment of acute lymphoblastic leukemia and diffuse large B-cell lymphoma.185 However, due to the characteristics of the solid immune TME and tumor structure, CAR-T cannot effectively penetrate into the tumor site and have a tumor killing effect. In order to further clear the obstacles, H. Y. Xie et al. fabricated PD-1 antibody-coated iron oxide nanoclusters. Then the activated T cells combined with nanoclusters may be successfully recruited to the tumor sites under the guidance of an in vitro magnetic field.191 At the same time, due to the existence of the acidic TME, PD-1 antibodies can undergo targeted release at the tumor site, blocking the inhibition of the function of activated T cells by tumor cells.
4.5. Others
We have previously introduced strategies to activate the immune response by inducing ICD to release antigens, delivery of antigens or agonists, and activating T cells. However, due to the complex interaction between tumor cells and the TME, a single measure may not activate the anti-tumor response effectively in vivo.192 For the antitumor response, the main tumor killing effect is mediated by CTLs. However, the activating of this effective T cell immune response is conducted by functions such as phagocytosis, processing and presentation of antigens by DCs. Mature DCs present antigens to T cells in lymph nodes and activate tumor-specific CD8+ CTLs. DCs play a key role in initiating tumor immunity.193 Antigens are the premise of activating a specific T cell immune response, but the presentation efficiency of antigens in vivo is low, and it is not easy to induce an efficient Th1 immune response. Common TLR agonists include TLR9 agonist (CpG) and TLR3 agonist (Poly I:C), which can effectively promote the Th1 immune response.194 The use of adjuvants such as TLR receptor agonists can promote the maturation of DCs. The combined use of antigens and TLR agonists can effectively promote the cross presentation of antigens and induce a Th1 immune response to play the role of tumor killing.195 In recent years, it is found that nanoparticles themselves can play the role of an adjuvant to activate DCs and the adjuvant effect is affected by the size and morphology.76,170,196,197 In addition, DCs can also be cultured in vitro and stimulated by antigens. When these engineered DCs are infused in vivo, they will directly activate T cells to exert an anti-tumor immune response.198
4.5.1. Co-delivering antigens and TLR agonists.
CpG is a TLR9-dependent adjuvant for eliciting a Th1 type T cell response, and ROS can promote APC maturation.156 C. Z. Yu et al. designed PEI-functionalized dendritic mesoporous organosilica nanoparticles to deliver OVA antigen and CpG adjuvants.199 With the high GSH in the TME and the release of the antigen and adjuvant, CpG bound to intracellular TLR9 receptors and co-activated a OVA-specific immune response. GSH depletion can further lead to elevated ROS levels, and promote the maturation of APCs. J. C. Zhang et al. loaded OVA antigen in the synthesis process of a MOF, and realized the co-delivery of OVA and CpG.200 The resultant nanocomposites could dissociate and promote the lysosome escape of antigens under pH 5.0, and activate the cross presentation of antigens.
Traditionally, aluminum adjuvants have been used as agonists for Th2 type immune responses. They are often used in prophylactic vaccines related to infection prevention and promote antibody production. X. Sun et al. transformed an aluminum hydroxide adjuvant from a gel to nanocarriers for the co-delivery of OVA and CpG adjuvants.201 The resultant nanocomposites could effectively improve the delivery and co-localization of OVA and CpG adjuvants in DCs and macrophages. They could also promote the infiltration of CTLs in tumor tissue, promote the secretion of IFN-γ, and obtain more effective OVA-specific killing efficiency.
The induced immune response is also affected by the size and morphology of nanoparticles.79 In order to optimize the co-delivery efficiency of antigens and adjuvants, L. Zhan et al. used different sizes of spherical Au nanoparticles to deliver OVA antigens and CpG adjuvants respectively.76 Au nanoparticles of 60 nm showed the strongest effect of antigen presentation while 80 nm nanoparticles have the best CpG delivery ability. Compared with the single component Au nanoparticles, AuNP60/OVA and AuNP80/CpG nanocomposites could significantly promote the homing of OVA and CpG adjuvants to lymphoid tissue and promote the OVA-specific CD8+ T cell response.
Poly(I:C), a TLR-3 agonist, is a negatively charged double-stranded RNA. SIINFEKL is an epitope peptide in OVA antigens that can be efficiently presented by MHC-I. Using the electrostatic interaction between the positive SIINFEKL epitope peptide modified by arginine (SIIN*) and poly(I:C) adjuvant, IPEM-Au nanocomposites were prepared by self-assembly of immune membranes (IPEMs) on Au.202 The resultant nanocomposites could effectively internalize and activate the TLR signal in primary DCs, and further present SIIN* epitope peptides.
4.5.2. Activating DCs by nanocomposites.
DCs are APCs, which play an important role in initiating and regulating innate and acquired immunity. DCs can sense external danger signals, such as foreign proteins, cellular receptors, pathogen associated molecular patterns and DAMPs. These danger signals can activate DCs, and then initiate the specific immune response of antigens and play an anti-tumor effect.203 In order to further improve the specific immune response of tumor-associated antigens, adjuvants such as TLR agonists are usually needed to further activate DCs.204,205 In addition to common immune adjuvants, nanoparticles themselves can also induce innate immunity, especially DC activation. The particle size, morphology and symmetry could all influence the activation of DCs. E. G. Bennett et al. found that both mesoporous silica nanoparticles (270 nm) and microparticles (2.5 μm) with surface areas above 500 m2 g−1 could promote the expression of CD86 on the surface of DCs.197 H. Sawa et al. synthesized gold nanoparticles to deliver WNV envelope protein and found that gold nanoparticles could promote the level of WNV-specific antibodies.79 Meanwhile, the size and shape of nanocomposites may influence the secretion of cytokines by bone-marrow-derived DCs (BMDCs). C. Z. Yu et al. synthesized asymmetric silica nanoparticles and found that asymmetric nanostructures could induce higher levels of CD40 and CD86 expression on the surface of DCs and macrophages compared with symmetrical structures.206 H. Y. Liu et al. used glucose modified mesoporous silica nanoparticles to obtain a biodegradable carbon–silica nanocomposite (denoted as CSN).207 CSN could significantly induce the expression of CD86 and CD80 markers on DCs and promote the maturation of DCs. The cGAS-STING pathway can enhance the secretion of type I IFN and promote the maturation of DCs by sensing the damage of DNA in cells.208 Studies have shown that Mn2+ can promote the activities of cGAS and STING and enhance the ability of the STING pathway to activate innate immunity.209 Z. Zhang et al. prepared nanocomposites employing DOX-loaded amorphous porous manganese phosphate nanoparticles and phospholipids.210 DOX was observed to lead to DNA damage and then stimulate the STING pathway. Mn2+ could further enhance the STING pathway, stimulate the secretion of interferon type 1, and then promote the maturation of DCs.
A DC vaccine has been approved by the US FDA for the treatment of prostate cancer.211 A DC vaccine refers to the use of patients' own mononuclear cells to induce DC production in vitro, and then loading the tumor antigen, and finally obtaining DCs activated by a peptide presenting epitope on the surface. The control of the DC maturation is a key issue in the preparation of a DC vaccine, as immature DCs cannot effectively activate CD8+ T lymphocytes while mature DCs show reduced capability of antigen phagocytosis and cross-presentation.212 Y. Li et al. surface-engineered DCs with polydopamine/Ca2+ nanocomposites for control over the DC maturation.212 Ca2+ acted as a physical bridge between the DC surface and polydopamine to maintain cell viability. Polydopamine could effectively prevent DC activation by scavenging ROS. Instead, NIR laser irradiation could remotely activate DC maturation through the photothermal effect of polydopamine (39 °C). Therefore, programmed DC maturation could be achieved in vitro, which is beneficial for DCs to play an efficient role in activating T cell immune responses.
5. Immune checkpoint blockade (ICB) therapy
ICB therapy is a widely used anti-tumor immunotherapy which has achieved great clinical success over the past few years. In order to avoid accidental damage to normal cells, when activated, T cells will express certain proteins that have the function of an “immune checkpoint”, such as PD-1. Corresponding proteins can be expressed on the surface of normal cells to prevent them from being attacked by T cells. However, tumor cells could overexpress some immunosuppressive proteins, such as PD-L1 or PD-L2, etc., and bind to the corresponding proteins expressed on T cells, tricking T cells and causing immune escape. This combination of tumor cells and T cells can inhibit T cell functions, thereby preventing T cells from effectively killing tumor cells. The role of drugs for ICB therapy is to replace the connection on the surface of tumor cells with corresponding antibodies or inhibitors, so that the matching between tumor cells and T cells could be blocked. Therefore, checkpoint blockade is used to expose the camouflage of tumor cells, so that T cells can restore their functions to attack tumor cells. Currently, checkpoint blockade strategies are commonly used in combination with inhibitors (such as anti PD-L1,55,93,213–215 anti PD-1,191,216,217–221 and anti CTLA-4,222–224), blocking peptides (such as AUNP12225) or plasmids to cut off PD-L1 gene expression in tumor cells,226 and so on. As mentioned earlier, the application of ICB therapy is still largely limited by low objective response rates, risk of autoimmune disease, and relatively high cost.227 Therefore, ICB therapy is usually combined with modulation of the immunosuppressive TME or activation of T cells. They work in a synergistic manner to inhibit tumor growth. In this section, we briefly introduce some examples of ICB treatment.
Organic materials are mostly used in ICB therapy. Z. Gu et al. employed 1-methyl-DL-tryptophan (1-MT, an IDO inhibitor)-modified HA to load anti PD-1 antibody and then integrated them into a microneedle system to inhibit melanoma growth.220 Then, the released anti PD-1 could exert PD-1 blockade to effectively induce T cells to attack cancer cells. This synergistic delivery strategy can trigger long-lasting and effective antitumor treatments. In addition, the delivery of anti PD-1 via hydrogels proved a good effect of inhibiting tumor angiogenesis. L. Wang et al. used an alginate hydrogel to co-deliver two FDA-approved drugs (celecoxib and anti PD-1) for tumor treatment.221 The combination of chemotherapy and ICB therapy could reduce immunosuppressive Tregs. It also increased the production of anti-vascular chemokines and inhibited tumor angiogenesis.
Organic/inorganic nanocomposites are also employed in ICB therapy. Iron oxide-based nanocomposites show excellent characteristics of a high loading rate and magnetic targeting. They could effectively deliver inhibitors to avoid side effects caused by systemic delivery. H. Y. Xie et al. prepared magnetic nanoclusters modified with anti PD-1 (IONP/anti PD-L1, Fig. 13a).191 They used anti PD-1 to improve the effect of adoptive cell transfer therapy, which has been widely used in clinical practice. Unlike general medical treatment, it requires extracting T cells from patients, expanding them in vitro, and then returning them to patients. Magnetic targeting was utilized to improve the accumulation of nanocomposites and utilization of anti PD-1. Taking advantage of the magnetic properties and combination of anti PD-1 and PD-1, T cells are efficiently transported to the tumor site with MRI guidance (Fig. 13b and c). The therapeutic effect of immune cytotoxicity and checkpoint blockade was significantly improved with prolonged survival time (Fig. 13d).
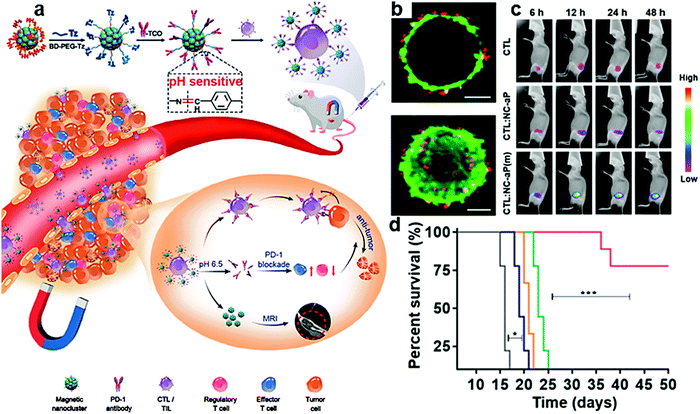 |
| Fig. 13 (a) Schematic illustration of IONP/anti PD-L1 for improved adoptive T cell therapy for solid tumors. (b) Representative confocal and corresponding 3D reconstruction images of T cells (green) and iron oxide-anti PD-L1 (red). (c) Visualization of the tumor-targeting ability. (d) Average survival percentages of mice after different treatments. Reproduced with permission from ref. 191. Copyright 2019, American Chemical Society. | |
J. P. Schneck et al. developed immunoswitch nanomaterials based on iron-dextran to deliver checkpoint inhibitors.228 The organic/inorganic nanocomposites can close the immunosuppressive PD-L1 pathway on tumor cells and simultaneously open the co-stimulatory 4-1BB pathway on CD8+ T cells by transmitting anti PD-L1 and anti 4-1BB. T cells are then stimulated by MHC-1 signals on the surface of tumor cells to activate and kill tumor cells. Sequencing analysis of tumor infiltrating T cells showed that enhanced tumor growth inhibition was achieved by altering the T cell receptor sequence and thereby inducing the expansion of the CD8+ T cell population. The design of the nanocomposites could improve the effectiveness of blocking agents and reduce costs, thereby avoiding the complexity of using multiple therapeutic agents. At the same time, further adjustments could be made to optimize the biodistribution, such as designing the shape and size of the nanoparticles to reduce the clearance rate.
Gold-based nanocomposites provide excellent computed tomography (CT) imaging capabilities for ICB therapy. This is of significance since it usually takes two months after treatment to assess whether a patient responds to ICB therapy. R. Popovtzer et al. utilized the CT imaging function of gold nanoparticles to integrate visualization of the treatment and shorten the evaluation time.229 Tumor-bearing mice showed different anti PD-L1 uptake rates, which corresponded to the infiltration of T cells in the tumor site and the tumor suppressing effect. This strategy can reduce the amount of immunotherapy drugs, and can predict the patient's response to ICB treatment, and accelerate personalized treatment.
Compared with organic nanomaterials used for ICB therapy, organic/inorganic nanocomposites may provide more possibilities, such as tumor targeting and visualization. Due to their optical, electrical, and magnetic properties, organic/inorganic nanocomposites used in PTT, PDT, magnetocaloric therapy, etc. could be combined with ICB therapy to achieve synergistic effects.
6. Combination of immunotherapy strategies
In addition to the immunotherapy strategies discussed above, the regulation of the immune TME, activation of immune responses, and ICB therapy could be combined to effectively inhibit tumor growth. Modulation of the TME can improve the therapeutic effect on tumors, but its own therapeutic effect is not ideal and needs to be used in combination with other treatments. Activation of immune responses by PTT, PDT and other therapies can effectively kill tumors locally, but they may also cause upregulation of immunosuppressive cells, PD-1, and PD-L1. ICB therapy has been used in clinical practice, but it only works for certain patients. The combination of regulating the immunosuppressive TME and activating immune responses can enable checkpoint inhibitors to function efficiently. Therefore, the combination of immunotherapy strategies has become the general trend. Versatile organic/inorganic nanocomposites offer more possibilities for combination. In this section, we discuss the combined immunotherapy strategies mediated by organic/inorganic nanocomposites.
6.1. Modulation of the immunosuppressive TME and ICB therapy
By modulation of the TME from an immunosuppressive state to a promoted state, “cold” tumors are transformed into “hot” tumors that are sensitive to treatments. In this case, if ICB therapy is combined then checkpoint blockade will be greatly improved. PD-1/PD-L1 checkpoint blockade is usually selected as a representative.
ROS is an important signaling messenger that affects the immune TME. By adjusting the level of ROS, the polarization of macrophages can be adjusted to make tumor cells sensitive to other treatments, and then up-regulate the expression of tumor-associated antigens, which can significantly improve the effect of ICB therapy. ROS-responsive nanoparticles could regulate the immune TME by modulating the ROS level to enhance the antitumor immune responses.218
In addition to adjusting ROS levels, there are more approaches for organic/inorganic nanocomposites to modulate the immunosuppressive TME. Combined with ICB therapy, the survival time of mice could be significantly improved. Z. Gu et al. prepared anti PD-1-loaded CaCO3 nanoparticles and the demethylating agent Zebularine to form a pH/ROS dual-responsive hydrogel (Fig. 14a).217 The tumor-associated antigen expression and reduction of MDSCs could be enhanced by Zebularine (Fig. 14b–d). Therefore, the immune TME was regulated to make tumor cells more favorable for T cell identification. However, Zebularine can induce increased PD-L1 expression on the surface of tumor cells, so it is necessary to combine with ICB therapy. Anti PD-1 blocks the PD-1/PD-L1 interaction and triggers a strong anti-melanoma immune response. The design of pH/ROS dual-responsiveness of the hydrogel helps the controlled and sustained release of Zebularine and anti PD-1. Compared with Zebularine or anti PD-1 treatment, the Zebularine and anti PD-1 combined treatment showed significant tumor suppression, and the survival time of mice was significantly prolonged. It was also found that locally delivered organic/inorganic nanocomposites can effectively induce a systemic anti-tumor immune response. This strategy, which combines the regulation of the immune TME and ICB therapy, can help suppress tumor growth and prolong the survival time of B16F10 melanoma mice.
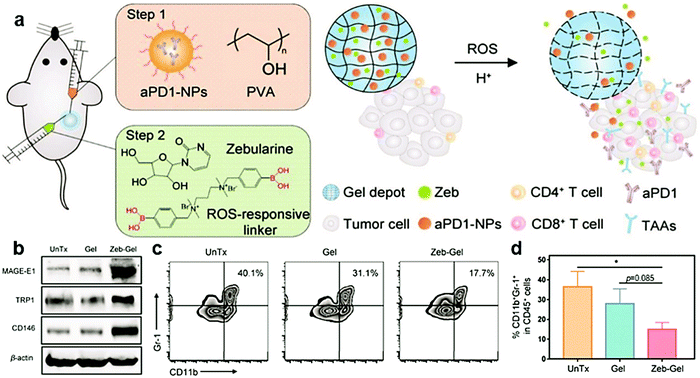 |
| Fig. 14 (a) Schematic illustration of the combination strategy of TME modulation and ICB therapy using ROS/H+ responsive scaffolds. (b) Tumor-associated antigen expression analyzed by western blotting assay. (c and d) Representative images and the quantitative analysis of MDSCs (CD11b+Gr-1+) in CD45+ cells by flow cytometry. Reproduced with permission from ref. 217. Copyright 2019, Wiley-VCH. | |
Taking advantage of CaCO3 and anti PD-L1 to inhibit tumor growth and recurrence, Z. Gu et al. designed a fibrin gel for postoperative cancer immunotherapy.230 In this study, the removal of H+ during the degradation of CaCO3 nanoparticles may be responsible for the polarization of M2 macrophages to M1 macrophages. Anti CD47 was loaded into CaCO3 nanoparticles to block the “don’t eat me” signal expressed by tumor cells through CD47 to improve the recognition and clearance of macrophages and T cells. By systemic injection of anti PD-L1, the regulation of the immunosuppressive TME and ICB therapy were combined, while promoted local antitumor immune responses and systemic suppression of tumor recurrence were realized.
In addition to CaCO3, the design of components of organic/inorganic nanocomposites may bring more functions. For example, if MnO2 or IONPs could be introduced, MRI or magnetic targeting could be integrated for imaging-guided cancer immunotherapy with high effectiveness.
6.2. Activation of immune responses and ICB therapy
Among the combined strategies, the combination of immune response activation and ICB therapy is the most widely used. There are various ways to activate immune responses. They could be activated by ICD through some treatments (including PTT,43,95,214,219,225,231,232 PDT,137,138,213,233–238 CDT,235,239 sonodynamic therapy,213 starvation therapy,240 magnetic hyperthermia therapy,222,241 radiation therapy223,232,242,243), agonists,213–215,219,222,223,233,244,245 or antigens,244,245etc. Many types of checkpoint blockade are used in combination with immunotherapy strategies. Checkpoints PD-L1,138,213,214,226,233–235,243,245 and IDO,137,242 which are located on tumor cells, or checkpoints PD-1,216,219,225,236,244 CTLA-4,222–224 and CSPG-4,43 which are located on T cells, can be selected. Some studies directly encapsulate the components used to activate T cells and checkpoint blockade to enter tumor tissue.137,216,225,242 Other studies choose to first transport materials to activate T cells, and then inject checkpoint inhibitors,43,138,213–215,219,222–224,233–236,243–245 which can improve the blocking effect more specifically.
6.2.1. ICD and checkpoint inhibitors.
PDT, PTT and other treatments can kill tumor cells through ROS or hyperthermia, and generate tumor-related antigens from tumor cell residues, activate the immune system, and produce a local antitumor immune response. However, PD-1/PD-L1 and other similar pathways inhibit the activation of CD8+ T cells. So while ICD causes antitumor immune responses, it will increase the number of immunosuppressive Tregs and up-regulate PD-1 and PD-L1 expression through the increase of IFN-γ,246,247 so the combined use with checkpoint inhibitors can restore the activity of CD8+ T cells and increase the antitumor effect. Moreover, the activation of immune responses and the use of checkpoint inhibitors can activate a systemic immune response.
PDT is a common tumor treatment method for ICD caused by ROS damage. It can destroy local tumors and minimize normal tissue damage, but it has no effect on suppressing tumor metastasis. The combination with ICB therapy can effectively induce antitumor immune responses.248 Some therapeutic-related agents, such as photosensitizer porphyrin,137,138,233–236 can be used as ligands and combined with metal ions to form nMOFs, which are excellent PDT nanoplatforms. PDT inhibits the growth of primary tumors and its combination with ICB therapy can effectively induce a systemic anti-tumor immune response. W. Lin et al. prepared Hf-chlorin nMOFs, and used their porous structure to load IDO inhibitors to achieve a combination of PDT and IDO checkpoint blocking to induce regression of treated primary tumors and untreated distal tumors.137 Massive stressed and dying necrotic tumor cells induced by PDT could be engulfed by the innate immune effector cells. After the tumor derived antigens were presented to T cells, a tumor-specific T cell response could be stimulated. However, IDO expressed by tumor cells can prevent T cells from expanding, inhibiting their activity and even causing T cell apoptosis. Combination with IDO inhibitors can restore T cell activity, increase T cell infiltration into tumors, and effectively kill cancer cells. To alleviate the problem of tumor hypoxia during PDT, Fe-porphyrin nMOFs were prepared to generate 1O2 under stimulation through a Fenton-like reaction.138 The combination of intraperitoneal injection of anti PD-L1 and PDT can cause systemic antitumor immune responses and induce T cell infiltration into the tumor. C. Yan et al. proposed upconversion nanoparticle (UCNP)-porphyrin nMOFs to improve the depth and tissue penetration of PDT (Fig. 15a–c).234 They used the scaffold structure of the MOF to load the hypoxia-activated drug tirapazamine. Chemotherapy was combined with PDT, and synergistically killed cancer cells to achieve the purpose of more effectively activating immune cells through ICD (Fig. 15d), and then combined with anti PD-L1 to improve the therapeutic effect on hypoxic tumors. W. Lin et al. synthesized Cu-porphyrin nMOFs, which cooperate with PDT and CDT to kill cancer cells by ROS,235 which could cause ICD and promote immune cell activation. After combination with anti PD-1, growth of primary and metastasis tumors could be effectively inhibited. For the combination of ICB therapy with PDT, photosensitizers could also be loaded in the nanocomposites.237,238 In addition to PDT, sonodynamic therapy could also be utilized to enhance the production of ROS. Y. Chen et al. used the ultrasound sensitizer hematoporphyrin monomethyl ether and the adjuvant R837 to prepare nanocomposites.213 After combining with anti PD-L1, an anti-tumor immune response and long-term immune memory could be realized.
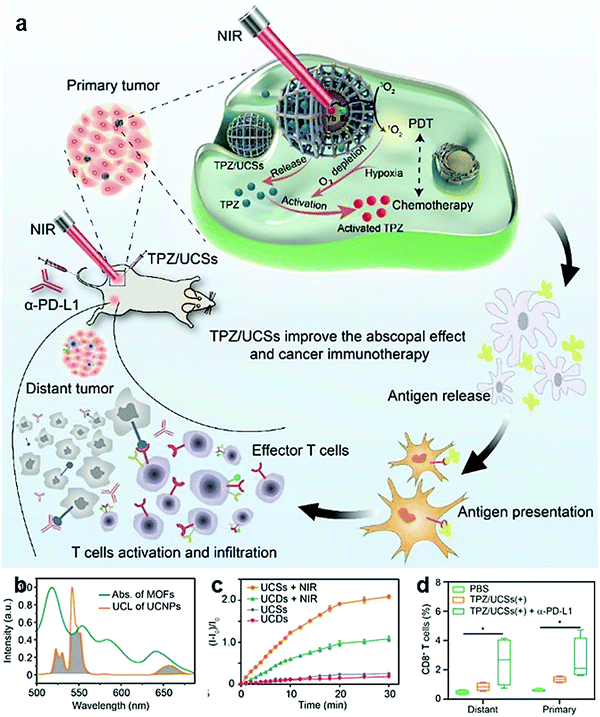 |
| Fig. 15 (a) Schematic illustration of the structure of UCNP-porphyrin nMOFs and their application for tumor treatment through the combination of NIR light-triggered PDT and hypoxia-activated chemotherapy with immunotherapy. (b) Absorption spectrum of the nMOFs. (c) NIR light-induced 1O2 generation by nMOFs determined by SOSG assay. (d) The percentage of tumor-infiltrating CD8+ T cells in total tumor cells. Reproduced with permission from ref. 234. Copyright 2020, American Chemical Society. | |
Hyperthermia induced by gold, carbon, and black phosphorus quantum dots with high light-to-heat conversion efficiency shows excellent antitumor efficacy, but at the same time the expression of PD-1/PD-L1 will be up-regulated. The combined use with checkpoint blockers could block the inhibition of T cells by these proteins and has excellent performance in inhibiting tumor metastasis and recurrence. J. You et al. encapsulated gold nanoshells and anti PD-1 peptide in poly(lactic-co-glycolic acid) to construct a photothermal ablation and ICB treatment strategy for malignant and metastatic tumors.225 The release of anti PD-1 peptide in the body is controlled by NIR light, which can achieve sustained release of drugs for up to 40 days, solving the problem of frequent clinical administration. This strategy combines a PTT-activated immune response with ICB therapy and shows low systemic toxicity but strong systemic immune responses. L. Mei et al. coated black phosphorus quantum dots with cancer cell membranes to form nanovesicles, which were loaded into a hydrogel that contains GM-CSF and LPS, and used them in combination with anti PD-1 (CpG/LPS-P).219 A good inhibitory effect in the surgical removal of residual tumors and metastatic cancer cells was observed (Fig. 16a and b). After NIR irradiation, GM-CSF and LPS can recruit (Fig. 16c) and activate (Fig. 16d) DCs, enhance antigen presentation, and activate T cells. In addition, injecting anti PD-1 into the tail vein can reinvigorate exhausted CD8+ T cells and induce a strong and durable antitumor immune response (Fig. 16e). This treatment strategy can effectively reduce the metastasis and recurrence of the primary tumor after surgical resection. Unlike conventional PTT, single-walled carbon nanotubes can not only be used for photothermal ablation of tumors, but also stimulate the maturation of DCs. Based on this characteristic, Z. Liu et al. selected single-walled carbon nanotubes with high light-to-heat conversion efficiency in combination with anti CTLA-4 to perform photothermal ablation of the primary tumor and prevent tumor metastasis.231 In the absence of light, the nanocomposites only stimulated the maturation of DCs and presented antigens originally present in the tumor cells, which could not provoke an effective immune response or inhibit tumor growth. When PTT was applied, a combination occurred since PTT eliminated the primary tumor and promoted the release of tumor-associated antigens, and then the maturation of DCs increased the activation efficiency of the immune responses. Furthermore, anti CTLA-4 therapy reduced Tregs activity and increased CD4+ T cell and CD8+ T cell infiltration, which improved the effective killing of tumor cells by the immune response. Moreover, magnetic hyperthermia therapy needs to be combined with ICB therapy to trigger a systemic antitumor immune response to treat diffuse metastatic tumors. Z. Liu et al. utilized the high magnetocaloric conversion efficiency of Fe3O4 to induce ICD caused by magnetic hyperthermia therapy.222 The combination of local injection of nanocomposites and systematic injection of anti CTLA-4 will lead to systemic therapeutic responses to inhibit tumor metastasis and trigger long-term immune memory to prevent tumor recurrence.
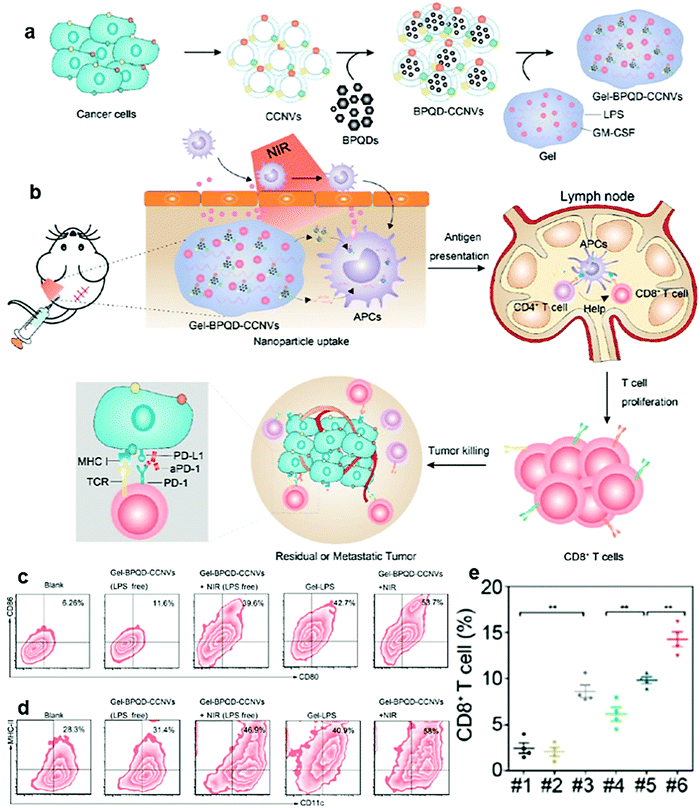 |
| Fig. 16 Schematic illustration of (a) preparation and (b) cancer immunotherapy of CpG/LPS-P. (c and d) Representative flow cytometry plots of (c) mature DCs and (d) CD11c+ MHC-II+ cells from different treatment groups. (e) Representative ratios of different groups of T cells in residual tumors from different groups. Reproduced with permission from ref. 219. Copyright 2019, American Chemical Society. | |
Radiation and starvation treatment can also destroy local tumor growth, and activate immune responses through ICD. After combining with ICB therapy, a systemic anti-tumor immune effect could be achieved. W. Lin et al. developed radiotherapy based on HF-porphyrin nMOFs (Fig. 17),242 which could efficiently absorb X-ray photons for radiotherapy. The combination with ICB therapy will expand the application of radiotherapy with a systemic antitumor immune response. In order to combine with ICB therapy, IDO inhibitors were loaded into nMOF pores and achieved an unprecedented 100% antitumor effect. Compared with traditional therapies, starvation therapy can provide more effective tumor ablation and induce an immune response. W. Liu et al. designed glucose oxidase-loaded silica nanoparticles to combine starvation therapy with immunotherapy.240 Glucose oxidase can convert glucose in tumor tissue to gluconic acid and hydrogen peroxide, cut off the energy supply to tumor cells, and cause apoptosis. After being injected with the nanocomposites and anti PD-1, higher DC maturation and infiltration of CD4+ T and CD8+ T cells were realized. Compared with monotherapy, the combination of starvation therapy and ICB therapy can effectively enhance the blocking effect of anti PD-1, showing great potential for cancer treatment.
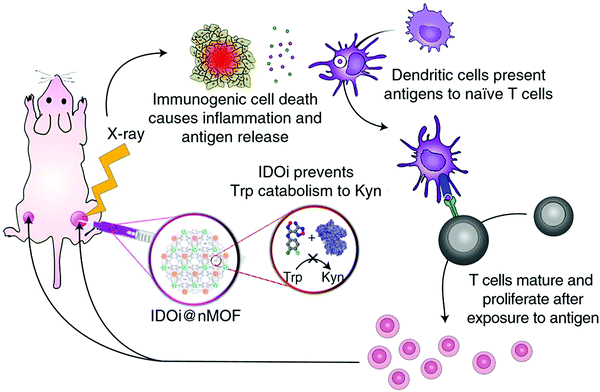 |
| Fig. 17 Hf-porphyrin nMOFs enable synergistic radiotherapy and immunotherapy. Reproduced with permission from ref. 242. Copyright 2018, Springer Nature. | |
6.2.2. ICD and agonists and checkpoint inhibitors.
The occurrence of an antitumor immune response is a complex process, which needs specific antigens, effective presentation of antigens, and activation of T cells. ICD can kill tumor cells and release tumor-associated antigens. Agonists can greatly activate DCs to present antigens to T cells. Checkpoint inhibitors will block pathways that inhibit T cell activity and increase the presence of tumor-infiltrating T cells and restore the T cell lethality. The combination of ICD, agonists and checkpoint inhibitors can effectively improve the activation of the antitumor immune response. This complementary therapy can produce systemic immunity against tumors.
When organic nanomaterials are used to realize the combination of ICD, agonists and checkpoint inhibitors, loading of photosensitizers or photothermal agents is usually needed. For example, Z. Liu et al. encapsulated the photothermal agent ICG and agonist R837 in poly(lactic-co-glycolic) acid to produce a powerful immune response.249 Then through systemic injection of anti CTLA-4 to combine with ICB therapy, the activated immune response could effectively inhibit tumor metastasis. In contrast, organic/inorganic nanocomposites can cause ICD themselves,214,222,233,250 making the combination easier. While activating the immune responses, more functions could be achieved, such as increased tissue penetration depth, magnetic targeting, and visualization of treatment. W. Lin et al. designed W-porphyrin nMOFs for the combination of PDT with anti PD-L1, and the nMOFs were used to load the agonist CpG (Fig. 18a).233 Cancer cells killed by PDT could provide tumor-associated antigens, while agonists stimulate DCs to present antigens to increase the T cell activity (Fig. 18b–e). In addition, inhibitors restore the function of T cells. The combination of PDT, agonists and ICB therapy can provide a tumor inhibition rate of ∼97%. Z. Liu et al. fabricated R837-Ce6/UCNP nanocomposites for a similar combination.251 Anti CTLA-4 was introduced to combine activated immune responses with ICB therapy. Under NIR irradiation, PDT directly destroyed tumor cells, and R837 stimulated an immune response by triggering DC maturation and cytokine secretion. The combination of immunotherapy strategies could effectively eliminate primary tumors through PDT, inhibit distant tumors, and induce long-term immune memory effects.
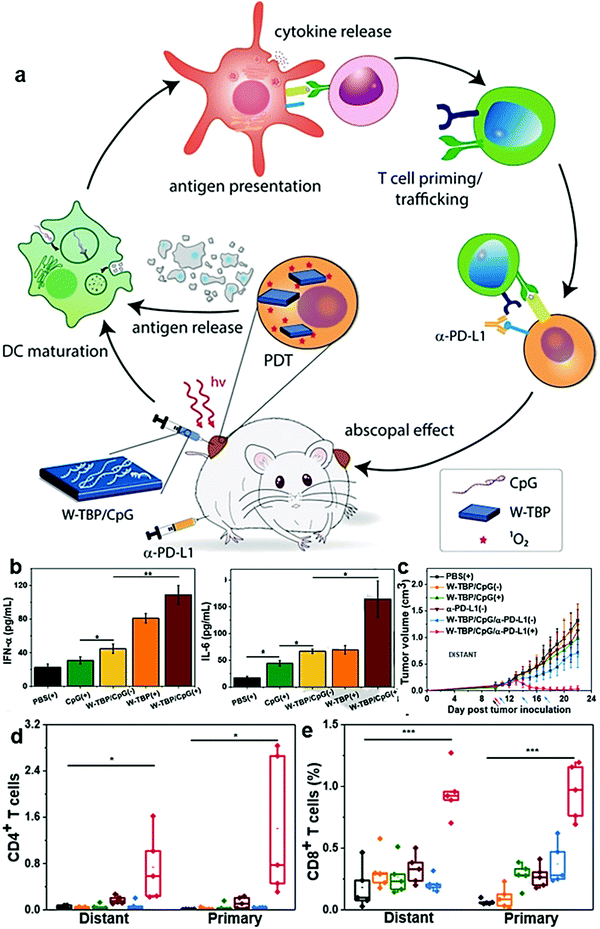 |
| Fig. 18 (a) W-porphyrin/CpG promoted antigen presentation via immunogenic PDT to afford systemic antitumor immunity. (b) ELISA of IFN-γ and IL-6 in blood of mice. (c) Growth curves of distant tumors of bilateral TUBO tumor-bearing mice. (d and e) The percentage of tumor-infiltrating CD4+ T cells (d), and CD8+ T cells (e) with respect to the total tumor cells. Reproduced with permission from ref. 233. Copyright 2019, Wiley-VCH. | |
In addition to ROS, hyperthermia can also be used in combination with agonists and checkpoint inhibitors to fight tumors. Z. Liu et al. prepared Fe3O4 nanoparticles as magnetic hyperthermia agents, and through local application of agonist R837 and systemic injection of checkpoint inhibitor anti CTLA-4, long-term systemic therapy is achieved.222 After magnetic hyperthermia therapy released tumor-associated antigens, a small amount of local injection of R837 could effectively stimulate DCs to rapidly process and present tumor antigens, which in turn stimulated T cells to produce immune responses. However, the Tregs in the distal tumor also increase, and cannot completely inhibit the growth of the distal tumor. After combining with anti CTLA-4, the activity of Tregs was inhibited, and completely inhibition of distant tumors could be achieved. B. Yang et al. used FDA approved poly(ethylene glycol)-block-poly(lactic-co-glycolic acid) copolymer, Fe3O4 nanoparticles, and imiquimod (R837) to form nanocomposites.214 In this work, Fe3O4 acts as photothermal, magnetic targeting, and T2 contrast agents. Under NIR irradiation, tumor-associated antigens could be released to activate immune responses. R837 promotes DC maturation, thereby enhancing the activation and proliferation of antigen-specific lymphocytes. Combined with anti PD-L1, a strong systemic antitumor immune response could be stimulated and promoted by PD-L1 ICB therapy to prevent tumor metastasis.
6.2.3. Antigens and agonists and checkpoint inhibitors.
Unlike ICD-induced tumor-associated antigen release, organic/inorganic nanocomposites can carry foreign antigens (such as OVA or cancer cell membranes) to directly stimulate APCs and activate antitumor immune responses. Combined with agonists and ICB therapy, this can help improve the recognition and lethality of T cells to obtain long-term systemic antitumor effects.
The model protein OVA is a commonly used foreign antigen and has broad applications in the design of tumor vaccines. Some metal–organic complexes loaded with OVA and agonists can significantly stimulate the maturation of DCs and present antigens. The synergistic effect with checkpoint inhibitors can improve the therapeutic effect and prolong their survival time. For example, Z. Liu et al. constructed a coordination polymer nanoplatform using Mn-meso-2,6-diaminopimelic acid, which was used to carry OVA and combined with anti PD-1 to inhibit the tumor growth of B16-OVA tumor-bearing mice (Fig. 19a).244 In this work, OVA stimulated DC maturation. With the help of agonist meso-2,6-diaminopimelic acid, it could effectively enhance the antigen presentation of DCs by promoting the expression of IL-12p40 and TNF-α, and further activate the immune response (Fig. 19b). The presence of Mn2+ enabled an MRI function to monitor the in vivo delivery of nanocomposites in real time (Fig. 19c). The intravenous injection of anti PD-1 combined ICB therapy with OVA and agonists to obtain better therapeutic effects (Fig. 19d and e). The study also showed that there was an immune memory effect in mice. S. R. Little et al. loaded OVA on aluminum-based alginoketal nanoparticles, which encapsulated the IDO inhibitor 1-MT and agonist poly(I:C), and then used anti PD-L1 in combination to demonstrate a good therapeutic effect for melanoma.252 After the as-prepared nanocomposites were delivered to the tumor site, the level of kynurenine was detected to decrease while the content of INF-α increased, indicating that the activity of IDO was inhibited. After combination with anti PD-L1, the growth of primary and contralateral tumors was significantly inhibited, and the survival rate in one month reached 100%.
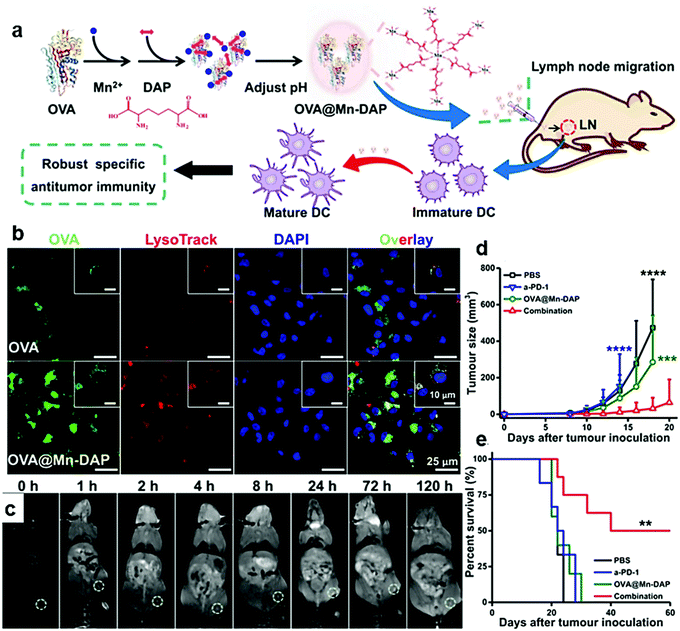 |
| Fig. 19 (a) Schematic illustration of the synthesis and function of OVA-Mn-meso-2,6-diaminopimelic acid nanocomposites. (b) Confocal fluorescence images of DCs after incubation with free OVA-cy5.5 or OVA-cy5.5@Mn-DAP for 20 h. (c) In vivo T1-weighted MR images at various time points. (d) Average tumor growth curves for different groups of B16-OVA tumor-bearing mice after different treatments. (e) Survival data of various groups of mice. Reproduced with permission from ref. 244. Copyright 2019, American Chemical Society. | |
Mesoporous silica nanoparticles could easily control the adsorption and release of antigens and adjuvants due to the unique pore structure. J. Kim et al. prepared an injectable dual-scale mesoporous silica vaccine which could be loaded with OVA and agonist CpG and combined with anti CTLA-4 for anti-tumor immunotherapy.253 In this study, mesoporous silica microrods were used to form a three-dimensional macroporous skeleton, which induced DCs to enter the skeleton by releasing DC chemokines. Then mesoporous silica nanoparticles carrying OVA and CpG were internalized by the recruited DCs to activate DCs and stimulate them to present antigens to T cells. This dual-scale mesoporous silica vaccine could activate a large number of antigen-specific T cells and significantly inhibit melanoma growth. After intraperitoneal injection of anti CTLA-4, the combination of ICB therapy and an antigen-specific vaccine can synergistically enhance the anti-tumor response and further inhibit tumor growth, which demonstrated a higher anti-tumor effect and animal survival rate.
Unlike OVA antigens, cancer cell membrane antigens come from patients, which have more complex surface antigens to mimic clinical conditions and can be metabolized in biological systems without generating by-products. Therefore, for clinical applications, future research may focus on the use of tumor-associated antigens (such as cancer cell membranes or tumor lysates) to achieve personalized cancer therapy. C. Wang et al. selected cancer cell membranes to coat Ag nanoparticles and combined with anti PD-L1 to achieve great inhibition of tumor metastasis and recurrence in both B16F10 and 4T1 tumor models.245 This research directly proved the importance of the combination of tumor-associated antigen-activated immunity and ICB therapy.
6.2.4. Others.
There are some other methods to realize the combination of activated immune responses and ICB therapy in addition to those mentioned above, such as combined agonists and checkpoint inhibitors, or combined antigens and checkpoint inhibitors, etc. These methods can also enhance the T cell responses to a certain extent, effectively enhance the treatment effects of ICB therapy, reduce adverse reactions, and improve the survival time of mice.
S. Y. Chen et al. synthesized Fe3O4-fucoidan-dextran nanoparticles, which are coupled with the agonist anti CD3/anti CD28 through dextran. Then anti PD-L1 was integrated to achieve the combined antitumor effect of an agonist and checkpoint inhibitor.254 By intravenous injection, the magnetic nanocomposites could rejuvenate T cells through agonists. Meanwhile, the nanocomposites could target tumors through magnetic targeting, which could reduce off-target effects to the greatest extent, improve the therapeutic effect and reduce the dosage of nanocomposites. Compared with anti-PD-L1 ICB treatment alone, the combination of the agonist and anti PD-L1 can extend the survival time of mice from 32 to 63 days. This work demonstrates the potential of integrating anti PD-L1 and agonists into a nanocomposite to enhance the therapeutic effect of ICB therapy.
The presentation of foreign antigens by DCs can also cause the activation and infiltration of T cells, which could combine with ICB therapy to promote the activation and proliferation of T cells and reduce tumor recurrence. L. Huang et al. skillfully delivered two nucleic acids through liposome-coated calcium phosphate nanoparticles.255 One nucleic acid encoded the mRNA of melanoma tumor antigen as an antigen, and the other nucleic acid encoded siRNA that blocks the expression of PD-L1 as a checkpoint inhibitor, which combines antigen delivery and ICB therapy to activate the immune system. The nanoparticles are functionally modified with mannose as a targeting ligand and a long-term inhibitory effect on tumor growth and metastasis was observed. After subcutaneous administration, mRNA antigens and siRNA that blocks expression of the PD-L1 immune checkpoint can be introduced into DCs at the same time. Acid-responsive degradation of calcium phosphate in the lysosomal region prompted the internalization of nanocomposites to quickly release the cargo. The mRNA vaccine encoding melanoma antigen stimulated DCs to mature, activated their presentation of antigens to T cells, and triggered a strong antigen-specific T cell response. The co-delivery of PD-L1 siRNA and the mRNA vaccine resulted in the down-regulation of PD-L1, thereby significantly promoting the activation and proliferation of T cells. The enhanced T cell response has an effective inhibitory effect on tumor growth and metastasis. This work provides a reference for the development of mRNA vaccine vectors to enhance the antitumor immune responses.
6.3. Combination of immune TME modulation, immune response activation and ICB therapy
In the last section, we introduced organic/inorganic nanocomposites used for combined activation of the immune system and ICB therapy, which has a significant effect on the effectiveness of immunotherapy to inhibit tumor metastasis and recurrence. However, there are a variety of immunosuppressive cells in the immune TME (such as MDSCs, M2 TAMs, and Tregs), which affect the therapeutic effects. Reversing immunosuppression can enhance the tumor response to various treatments. Therefore, a combination of modulation of the immunosuppressive TME, activation of the immune response, and ICB therapy could further improve the therapeutic effectiveness of immunotherapy. Firstly, modulation of the immunosuppressive TME makes the tumor change to a sensitive state, which is convenient for treatments. Then, more T cells are activated to kill tumor cells. And, finally, the breakthrough in the self-protection of tumor cells by checkpoint inhibitors could avoid immune escape and enable T cells to perform more powerful functions. The combination of these three strategies will improve the efficiency of antitumor immune responses and the therapeutic effect in all aspects.
MnO2 and Fe3O4 nanoparticles exhibit excellent characteristics in the design of nanomaterials combining these three immunotherapy strategies. The fabrication of organic/inorganic nanocomposites based on these two materials can simultaneously modulate the immunosuppressive TME, activate immune responses, and realize ICB therapy. Because MnO2 nanoparticles can catalyze the decomposition of H2O2 in the TME to produce O2, they could improve hypoxia at the tumor site. MnO2-based nanocomposites can polarize M2 macrophages to M1 macrophages and improve the immune TME. At the same time, MnO2 nanoparticles could be easily formed as hollow structures which possess high loading capacity and can be used for drug delivery to activate immune responses. They can be used in combination with checkpoint inhibitors to maximize the effect of immunotherapy. In addition, Mn2+ could be utilized for MRI, which is beneficial for tracking the transport and responsive degradation of the nanocomposites in the body, and for visualizing of the treatment process. Fe3O4 can regulate macrophage polarization to modulate the immunosuppressive TME. At the same time, Fe3O4 nanoparticles possess good magnetothermal and photothermal conversion efficiency, and can activate immune responses through ICD. In combination with checkpoint inhibitors, activated immune responses could be increased to enhance the antitumor effect. Moreover, Fe3O4 possesses unique magnetic properties, which can realize magnetic targeting and MRI at the tumor site.
Z. Liu et al. designed hollow MnO2 nanoparticles to load photosensitizer Ce6 and chemotherapeutic drug DOX, which were modified with PEG to obtain excellent stability and dispersibility. Anti PD-L1 was then integrated to inhibit in situ and distal tumor growth.93 After injection of the nanocomposites, strong Ce6 fluorescence and T1-weighted MR signals appeared in tumors, indicating the high passive tumor homing of these nanocomposites. Under the acidic microenvironment, MnO2 nanoparticles degraded to release drugs. In addition, O2 generated in situ could relieve tumor hypoxia, polarize M2 macrophages to M1 macrophages, and improve the immune TME. ICD could be induced by both Ce6-mediated PDT and DOX-mediated chemotherapy, which activated the immune responses to inhibit tumor growth. In order to achieve systemic antitumor immunity, anti PD-L1 was introduced to combine with modulation of the immunosuppressive TME and activation of the immune system. The combination of the three strategies mainly gave CD4+ and CD8+ T cells better tumor cell lethality. X. Zhang et al. also used the advantages of MnO2 and anti PD-L1 to conduct the design of nanocomposites.55 They used the cavity of hollow MnO2 nanoparticles to load a glycolysis inhibitor and LOX. In this research, LOX consumes lactic acid, and the resultant H2O2 is decomposed into O2 by MnO2, which was used in the glycolysis process. The consumption of lactic acid could regulate the phenotype of macrophages, and exhibited excellent regulatory capacity for the immune TME. The use of glycolysis inhibitors impedes glucose metabolism in tumor cells, thereby cutting off the supply of energy to achieve antitumor therapy and induce ICD, which activated T cell immune responses. After combining with anti PD-L1, the T cell function was enhanced and synergistic tumor suppression effects were realized.
Z. Zhang et al. designed organic/inorganic nanocomposites based on Fe3O4 that regulated the immune TME, activated the immune system, and blocked checkpoints.58 They encapsulated Fe3O4 nanoparticles in tumor-derived antigen particles, and loaded the agonist CpG on the surface. By applying anti PD-L1 to combine with ICB therapy, the nanocomposites can synergistically regulate the immunosuppressive TME and the host's anti-tumor immunity. The nanocomposites distributed in the TME can regulate ROS through a Fenton reaction, and modulate the infiltrating TAMs to the M1 phenotype. The regulation transformed the “cold” tumor into a “hot” tumor, and induced the infiltration of a large number of CTLs. The tumor inhibition rate reached 83%, and the survival time of the mice was extended to 3 months. This strategy shows that the co-delivery of antigens, agonists, and checkpoint inhibitors, and the regulation of the immune TME are very effective for combination immunotherapy strategies, but more in-depth research is still needed for clinical cancer treatment.
In addition to MnO2 and Fe3O4, the chemotherapeutic drug docetaxel also exhibits excellent immune TME regulation ability, which can be used to design nanocomposites for a combination of immune TME modulation, immune response activation and ICB therapy. C. Dong et al. proposed multifunctional CuS-docetaxel/CpG nanocomposites for collaborative phototherapy (including PDT and PTT) and enhanced immunotherapy (Fig. 20a).256 FA provided a targeting function for the nanocomposites to improve their accumulation at the tumor site. CuS nanoparticles with high photothermal conversion efficiency worked as photothermal agents and carriers. As a photosensitizer, porphyrin participated in the PDT. The resultant ROS hyperthermia from PTT could damage cancer cells to activate the immune responses by ICD. Docetaxel could reduce the proportion of MDSCs and induce MDSC polarization to M1 macrophages, which helped promote the production of inflammatory cytokines for improved immunotherapy (Fig. 20b). The presence of CpG accelerated the maturation of DCs and better presented the antigens to T cells. Combined with anti PD-L1, it is beneficial to block the dysfunction and failure of T cells in the PD-1/PD-L1 pathway, which could enhance T cell infiltration and effectively kill tumor cells (Fig. 20c). Therefore, CuS nanoparticles, porphyrin and CpG activated T cell immune responses by hyperthermia, ROS and DC maturation, respectively. Docetaxel was responsible for immunosuppressive TME regulation. Anti PD-L1 restored the T cell function to improve the tumor lethality. These three ways cleverly combined to regulate the immune responses and enhance the effect of T cells by employing CuS nanoparticles as carriers. This combination of docetaxel, PDT, PTT, CpG, and anti PD-L1 demonstrated a strong antitumor effect and may provide new ways for breast cancer immunotherapy.
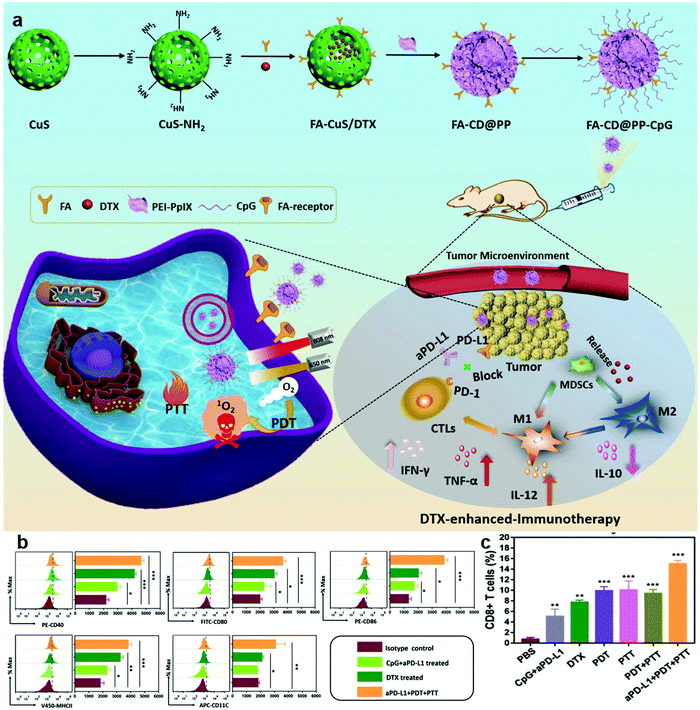 |
| Fig. 20 (a) Rational design and synthesis of CuS-docetaxel/CpG nanocomposites for docetaxel-enhanced immunotherapy. (b) Flow cytometric assay of CD40, CD80, CD86, MHC class II, and CD11c expression from different groups. (c) Proportion of tumor-infiltrating CD8+ T cells. Reproduced with permission from ref. 256. Copyright 2019, Wiley-VCH. | |
7. Conclusions and perspectives
In this review, we mainly summarized the design and application of organic/inorganic nanocomposites in cancer immunotherapy. From the perspective of achieving immune enhancement, nanocomposites play a role in cancer immunotherapy in three strategies: targeting the immunosuppressive TME, activating antitumor immune responses, and ICB therapy. Due to the versatility of nanocomposites, a combination of these immunotherapy strategies could also be realized through rational design of the components.
In general, hypoxia and the acid TME are the main causes of tumor immunosuppression. Therefore, nanomaterials that can improve hypoxia and the acidic pH inside the tumor might be able to alleviate the immunosuppressive TME. Some previous studies targeting the TME may require re-assessment of their impact on the immune microenvironment. At the same time, the combination of drugs targeting the tumor metabolic pathway can interrupt the production of hypoxia and lactic acid, and further modulate the immunosuppressive TME to activate antitumor immunity. Nanocomposites targeting the immune TME could usually polarize immunosuppressive M2 cells to pro-inflammatory M1 cells. When the nanocomposites are administered systemically, it is necessary to pay more attention to the side effects such as their accumulation and abnormal immune activation in non-tumor sites. This further raises higher requirements for the design of nanocomposites targeting the immunosuppressive TME.
The activation of antitumor immune responses is one of the most widely studied strategies mediated by nanocomposites for cancer immunotherapy. ICD can lead to the release of tumor-associated antigens, which in turn leads to the activation of immunity. However, immune activation induced by ICD can up-regulate the expression of cytokines such as IFN-γ, and then up-regulate the expression of PD-L1 on the surface of tumor cells to reduce the intensity of the immune response. Therefore, when ICD is used in tumor therapy, it is best to combine PL-L1 or PD-1 inhibitors to maximize the tumor killing effect of activated CTLs. In research of activating the immune response by delivering antigens, coating the nanoparticles with tumor cell membranes is an ideal strategy to improve the biocompatibility and delivery efficiency. However, due to the low abundance of tumor antigens on the surface of the tumor cell membrane, the intensity of the cellular immune response still needs to be further improved. Therefore, how to improve the abundance of tumor-associated antigens on tumor cell membranes is a problem that needs to be solved in the future. In addition, there is a risk of introducing PD-L1 while delivering tumor cell membranes, so the need for combined ICB therapy still needs to be considered.
When organic/inorganic nanocomposites are used in immunotherapy strategy combinations, most nanocomposites contain checkpoint inhibitors. Currently, more work is focused on the combination of activating immune responses and ICB therapy. The immunotherapy by this combination seems to be sufficient to suppress primary and metastatic tumors. A few studies were reported on combination of immune strategies to modulate the immunosuppressive TME and activate the immune response. Reversal of the immunosuppression can make the tumor cells sensitive to various therapies and may better activate antitumor immune responses. However, in some studies, it is found that if there are no checkpoint inhibitors, the immunotherapy effect is limited, which may cause tumor recurrence. Therefore, the combination of these three strategies may improve the interaction of T cells and tumor cells more comprehensively, which leads to a longer-term and effective therapeutic effect. From this perspective, organic/inorganic nanocomposites with the virtues of multiple functions, favorable properties, and diverse design strategies may have great potential in cancer immunotherapy.
Abbreviations
1-MT | 1-Methyl-DL-tryptophan |
1O2 | Singlet oxygen |
5-FU | 5-Fluorouracil |
aAPCs | Artificial antigen presenting cells |
APCs | Antigen-presenting cells |
apo A-I | Apolipoprotein A-I |
ATP | Adenosine triphosphate |
ATRA | All-trans retinoic acid |
AuNP@B16F10 | Au nanoparticles secreted by B16-F10 cells |
BM-Au | Coated AuNPs with bacterial outer membrane vesicles |
BMDCs | Bone-marrow-derived DCs |
BSA | Bovine serum albumin |
CAR-T | Chimeric antigen receptor T-cell |
CCR7 | CC-chemokine receptor 7 |
CDT | Chemodynamic therapy |
Ce6 | Chloride e6 |
CINP | Nanoparticles extracted from cuttlefish ink |
CpG ODNs | CpG oligodeoxynucleotides |
CpG/LPS-P | Hydrogel with GM-CSF/LPS and black phosphorus quantum dots |
CREKA | Cys–Arg–Glu–Lys–Ala |
CRT | Calreticulin |
CSN | Carbon–silica nanocomposite |
CT | Computed tomography |
CTLs | Cytotoxic T lymphocytes |
CuNG | Copper N-(2-hydroxy acetophenone)glycinate |
DAMPs | Damage-associated molecular patterns |
DHMSN | Biodegradable hollow mesoporous silica nanoparticles |
DOX | Doxorubicin |
EPR | Enhanced permeability and retention |
ER | Endoplasmic reticulum |
FA | Folic acid |
FDA | Food and drug administration |
Gem | Gemcitabine |
GMP | Gemcitabine monophosphate |
GO | Graphene oxide |
GSH | Glutathione |
HA | Hyaluronic acid |
HDL | High-density lipoprotein |
HIF1-α | Hypoxia-inducible factor-1 α |
HIONs | HA modified superparamagnetic iron oxide nanoparticles |
HMGB1 | High mobility group protein B1 |
HPPH | 2-[1Hexyloxyethyl]-2-devinyl pyro pheophorbide-alpha |
HSPs | Heat shock protein |
ICB | Immune checkpoint blockade |
ICD | Immunogenic cell death |
IDO | Indoleamine 2,3-dioxygenase |
IFN-α | Interferon-α |
IFN-γ | Interferon-γ |
IFR5 | Interferon regulatory factor 5 |
IONP/anti PD-L1 | Magnetic nanoclusters modified with anti PD-1 |
IONPs | Iron oxide nanoparticles |
IPEMs | Immune membranes |
LCP | Lipid-coated calcium phosphate |
LOX | Lactate oxidase |
MDSCs | Myeloid-derived suppressor cells |
MHC | Major histocompatibility complex |
MHR | H3R6 polypeptide conjugated multiwalled carbon nanotubes |
mRBC | Red blood cell membrane |
mRBC/MoSe2 | mRBC coated MoSe2 nanocomposites |
MRI | Magnetic resonance imaging |
MTD | Magnetothermodynamic |
Neuro2a cells | Neuroblastoma cells |
NIR | Near-infrared |
nMOFs | Nanoscale metal–organic frameworks |
NO | Nitric oxide |
NP@MF | Fusion cell membrane coated MOF nanoparticles |
OMVs | Outer membrane vesicles |
OVA | Ovalbumin |
PA | Photoacoustic |
PAMPs | Pathogen-related molecular pattern |
PD-1 | Programmed cell death protein 1 |
PD-L1 | Programmed death-ligand 1 |
PDNCs | Palladium nanosheets to deliver CpG |
PDT | Photodynamic therapy |
PEG | Poly(ethylene glycol) |
PEG | Polyethylene glycol |
PEG/NaCl | PEGylated sodium chloride nanoparticles |
PTT | Photothermal therapy |
PVP | Polyvinyl pyrrolidone |
RCC | Renal cell carcinoma |
RGD | Arginine–glycine–aspartic acid |
RNA/IO | Organic/inorganic nanocomposites based on mRNA |
ROS | Reactive oxygen species |
SCARB1 | Scavenger receptor type B-1 |
SIIN* | SIINFEKL epitope peptide modified by arginine |
TAMs | Tumor-associated macrophages |
TEM | Transmission electron microscopy |
TLR | Toll-like receptor |
TME | Tumor microenvironment |
TNF-α | Tumor necrosis factor α |
TRAF6 | TNF receptor associated factor 6 |
Tregs | Regulatory T cells |
UCNP | Upconversion nanoparticles |
WNV | West Nile virus |
ZIF-8 | Zeolitic imidazolate framework |
Conflicts of interest
The authors declare no conflict of interest.
Acknowledgements
This work was supported by the National Key Research and Development Program of China (Grant No. 2016YFA0201501), National Natural Science Foundation of China (Grant No. 51773013 and 51922022), Beijing Outstanding Young Scientist Program (Project No. BJJWZYJH01201910010024), and Fundamental Research Funds for the Central Universities (Project No. BHYC1705A and XK1802-2).
References
- F. Bray, J. Ferlay, I. Soerjomataram, R. L. Siegel, L. A. Torre and A. Jemal, Global cancer statistics 2018: GLOBOCAN estimates of incidence and mortality worldwide for 36 cancers in 185 countries, Ca-Cancer J. Clin., 2018, 68, 394–424 CrossRef PubMed.
- R. S. Riley, C. H. June, R. Langer and M. J. Mitchell, Delivery technologies for cancer immunotherapy, Nat. Rev. Drug Discovery, 2019, 18, 175–196 CrossRef CAS PubMed.
- J. Nam, S. Son, K. S. Park, W. Zou, L. D. Shea and J. J. Moon, Cancer nanomedicine for combination cancer immunotherapy, Nat. Rev. Mater., 2019, 4, 398–414 CrossRef.
- V. Velcheti and K. Schalper, Basic Overview of Current immunotherapy approaches in cancer, Am. Soc. Clin. Oncol. Educ. Book, 2016, 35, 298–308 CrossRef PubMed.
- M. G. Kramer, M. Masner, F. A. Ferreira and R. M. Hoffman, Bacterial therapy of cancer: promises, limitations, and insights for future directions, Front. Microbiol., 2018, 9, 16 CrossRef PubMed.
- T. Christofi, S. Baritaki, L. Falzone, M. Libra and A. Zaravinos, Current perspectives in cancer immunotherapy, Cancers, 2019, 11, 1472 CrossRef CAS PubMed.
- M. B. Atkins, L. Kunkel, M. Sznol and S. A. Rosenberg, High-dose recombinant interleukin-2 therapy in patients with metastatic melanoma: long-term survival update, Cancer J., 2000, 6(Suppl 1), S11–S14 Search PubMed.
- I. Melero, G. Gaudernack, W. Gerritsen, C. Huber, G. Parmiani, S. Scholl, N. Thatcher, J. Wagstaff, C. Zielinski, I. Faulkner and H. Mellstedt, Therapeutic vaccines for cancer: an overview of clinical trials, Nat. Rev. Clin. Oncol., 2014, 11, 509–524 CrossRef CAS PubMed.
- S. M. Vareki, C. Garrigós and I. Duran, Biomarkers of response to PD-1/PD-L1 inhibition, Crit. Rev. Oncol. Hematol., 2017, 116, 116–124 CrossRef PubMed.
- Q. Chen, M. Chen and Z. Liu, Local biomaterials-assisted cancer immunotherapy to trigger systemic antitumor responses, Chem. Soc. Rev., 2019, 48, 5506–5526 RSC.
- J. Tchou, Y. Zhao, B. L. Levine, P. J. Zhang, M. M. Davis, J. J. Melenhorst, I. Kulikovskaya, A. L. Brennan, X. Liu, S. F. Lacey, J. A. D. Posey, A. D. Williams, A. So, J. R. Conejo-Garcia, G. Plesa, R. M. Young, S. McGettigan, J. Campbell, R. H. Pierce, J. M. Matro, A. M. DeMichele, A. S. Clark, L. J. Cooper, L. M. Schuchter, R. H. Vonderheide and C. H. June, Safety and efficacy of intratumoral injections of chimeric antigen receptor (CAR) T cells in metastatic breast cancer, Cancer Immunol. Res., 2017, 5, 1152–1161 CrossRef CAS PubMed.
- S. Kakarla and S. Gottschalk, CAR T cells for solid tumors: armed and ready to go, Cancer J., 2014, 20, 151–155 CrossRef CAS PubMed.
- M. Kalos, B. L. Levine, D. L. Porter, S. Katz, S. A. Grupp, A. Bagg and C. H. June, T cells with chimeric antigen receptors have potent antitumor effects and can establish memory in patients with advanced leukemia, Sci. Transl. Med., 2011, 3, 73r–95r Search PubMed.
- Z. Zhao, Y. Chen, N. M. Francisco, Y. Zhang and M. Wu, The application of CAR-T cell therapy in hematological malignancies: advantages and challenges, Acta Pharm. Sin. B, 2018, 8, 539–551 CrossRef PubMed.
- L. Jeanbart and M. A. Swartz, Engineering opportunities in cancer immunotherapy, Proc. Natl. Acad. Sci. U. S. A., 2015, 112, 14467–14472 CrossRef CAS PubMed.
- M. S. Goldberg, Immunoengineering: how nanotechnology can enhance cancer
immunotherapy, Cell, 2015, 161, 201–204 CrossRef CAS PubMed.
- Z. Zhao, L. Zheng, W. Chen, W. Weng, J. Song and J. Ji, Delivery strategies of cancer immunotherapy: recent advances and future perspectives, J. Hematol. Oncol., 2019, 12, 126 CrossRef PubMed.
- M. Sylvestre, C. A. Crane and S. H. Pun, Progress on modulating tumor-associated macrophages with biomaterials, Adv. Mater., 2019, 31, 1902007 Search PubMed.
- M. Ovais, M. Guo and C. Chen, Tailoring nanomaterials for targeting tumor-associated macrophages, Adv. Mater., 2019, 31, 1808303 CrossRef PubMed.
- J. J. Moon, B. Huang and D. J. Irvine, Engineering nano- and microparticles to tune immunity, Adv. Mater., 2012, 24, 3724–3746 CrossRef CAS PubMed.
- L. Ke, P. Cai, Y. L. Wu and X. Chen, Polymeric nonviral gene delivery systems for cancer immunotherapy, Adv. Ther., 2020, 3, 1900213 CrossRef CAS.
- R. Meir, K. Shamalov, O. Betzer, M. Motiei, M. H. Fried, R. Yehuda, A. Popovtzer and C. J. Cohen, Nanomedicine for cancer immunotherapy: tracking cancer specific T cells in vivo with gold nanoparticles and CT imaging, ACS Nano, 2015, 9, 6363–6372 CrossRef CAS PubMed.
- X. Li, X. Wang and A. Ito, Tailoring inorganic nanoadjuvants towards next-generation vaccines, Chem. Soc. Rev., 2018, 47, 4954–4980 RSC.
- N. Zhao, L. Yan, X. Zhao, X. Chen, A. Li, D. Zheng, X. Zhou, X. Dai and F. J. Xu, Versatile types of organic/inorganic nanohybrids: from strategic design to biomedical applications, Chem. Rev., 2019, 119, 1666–1762 CrossRef CAS PubMed.
- M. Molina, M. Asadian-Birjand, J. Balach, J. Bergueiro, E. Miceli and M. Calderón, Stimuli-responsive nanogel composites and their application in nanomedicine, Chem. Soc. Rev., 2015, 44, 6161–6186 RSC.
- J. Shi, Y. Jiang, X. Wang, H. Wu, D. Yang, F. Pan, Y. Su and Z. Jiang, Design and synthesis of organic-inorganic hybrid capsules for biotechnological applications, Chem. Soc. Rev., 2014, 43, 5192–5210 RSC.
- J. Shen, W. Zhang, R. Qi, Z. W. Mao and H. Shen, Engineering functional inorganic-organic hybrid systems: advances in siRNA therapeutics, Chem. Soc. Rev., 2018, 47, 1969–1995 RSC.
- O. S. Wolfbeis, An overview of nanoparticles commonly used in fluorescent bioimaging, Chem. Soc. Rev., 2015, 44, 4743–4768 RSC.
- L. H. Reddy, J. L. Arias, J. Nicolas and P. Couvreur, Magnetic nanoparticles: design and characterization, toxicity and biocompatibility, pharmaceutical and biomedical applications, Chem. Rev., 2012, 112, 5818–5878 CrossRef CAS.
- D. Tarn, C. E. Ashley, M. Xue, E. C. Carnes, J. I. Zink and C. J. Brinker, Mesoporous silica nanoparticle nanocarriers: biofunctionality and biocompatibility, Acc. Chem. Res., 2013, 46, 792–801 CrossRef CAS PubMed.
- H. Koo, M. S. Huh, I.-C. Sun., S. H. Yuk, K. Choi, K. Kim and I. C. Kwon, In vivo targeted delivery of nanoparticles for theranosis, Acc. Chem. Res., 2011, 44, 1018–1028 CrossRef CAS PubMed.
- D. Rosenblum, N. Joshi, W. Tao, J. M. Karp and D. Peer, Progress and challenges towards targeted delivery of cancer therapeutics, Nat. Commun., 2018, 9, 1410 CrossRef PubMed.
- S. Mura, J. Nicolas and P. Couvreur, Stimuli-responsive nanocarriers for drug delivery, Nat. Mater., 2013, 12, 991–1003 CrossRef CAS PubMed.
- D. Roy, J. N. Cambre and B. S. Sumerlin, Future perspectives and recent advances in stimuli-responsive materials, Prog. Polym. Sci., 2010, 35, 278–301 CrossRef CAS.
- K. Knop, R. Hoogenboom, D. Fischer and U. S. Schubert, Poly(ethylene glycol) in drug delivery: pros and cons as well as potential alternatives, Angew. Chem., Int. Ed., 2010, 49, 6288–6308 CrossRef CAS PubMed.
- G. Pasut and F. M. Veronese, State of the art in PEGylation: the great versatility achieved after forty years of research, J. Controlled Release, 2012, 161, 461–472 CrossRef CAS PubMed.
- M. P. Monopoli, C. Åberg, A. Salvati and K. A. Dawson, Biomolecular coronas provide the biological identity of nanosized materials, Nat. Nanotechnol., 2012, 7, 779–786 CrossRef CAS PubMed.
- J. Fang, H. Nakamura and H. Maeda, The EPR effect: unique features of tumor blood vessels for drug delivery, factors involved, and limitations and augmentation of the effect, Adv. Drug Delivery Rev., 2011, 63, 136–151 CrossRef CAS PubMed.
- F. Dosio, S. Arpicco, B. Stella and E. Fattal, Hyaluronic acid for anticancer drug and nucleic acid delivery, Adv. Drug Delivery Rev., 2016, 97, 204–236 CrossRef CAS PubMed.
- W. Xia and P. S. Low, Folate-targeted therapies for cancer, J. Med. Chem., 2010, 53, 6811–6824 CrossRef CAS PubMed.
- S. L. Topalian, F. S. Hodi, J. R. Brahmer, S. N. Gettinger, D. C. Smith, D. F. McDermott, J. D. Powderly, R. D. Carvajal, J. A. Sosman, M. B. Atkins, P. D. Leming, D. R. Spigel, S. J. Antonia, L. Horn, C. G. Drake, D. M. Pardoll, L. Chen, W. H. Sharfman, R. A. Anders, J. M. Taube, T. L. McMiller, H. Xu, A. J. Korman, M. Jure-Kunkel, S. Agrawal, D. McDonald, G. D. Kollia, A. Gupta, J. M. Wigginton and M. Sznol, Safety, activity, and immune correlates of anti-PD-1 antibody in cancer, N. Engl. J. Med., 2012, 366, 2443–2454 CrossRef CAS.
- X. Luo, D. Tsai, M. Gu and M. Hong, Extraordinary optical fields in nanostructures: from sub-diffraction-limited optics to sensing and energy conversion, Chem. Soc. Rev., 2019, 48, 2458–2494 RSC.
- Q. Chen, Q. Hu, E. Dukhovlinova, G. Chen, S. Ahn, C. Wang, E. A. Ogunnaike, F. S. Ligler, G. Dotti and Z. Gu, Photothermal therapy promotes tumor infiltration and antitumor activity of CAR T cells, Adv. Mater., 2019, 31, 1900192 CrossRef PubMed.
- Y. Liu, P. Bhattarai, Z. Dai and X. Chen, Photothermal therapy and photoacoustic imaging via nanotheranostics in fighting cancer, Chem. Soc. Rev., 2018, 48, 2053–2108 RSC.
- J. N. Liu, W. Bu and J. Shi, Chemical design and synthesis of functionalized probes for imaging and treating tumor hypoxia, Chem. Rev., 2017, 117, 6160–6224 CrossRef CAS PubMed.
- Y. Guo, Y. Ran, Z. Wang, J. Cheng, Y. Cao, C. Yang, F. Liu and H. Rao, Magnetic-responsive and targeted cancer nanotheranostics by PA/MR bimodal imaging-guided photothermally triggered immunotherapy, Biomaterials, 2019, 219, 119370 CrossRef CAS PubMed.
- Y. Li, X. Zhang and C. Deng, Functionalized magnetic nanoparticles for sample preparation in proteomics and peptidomics analysis, Chem. Soc. Rev., 2013, 42, 8517–8539 RSC.
- Z. Zhou, Z. Shen and X. Chen, Tale of two magnets: an advanced magnetic targeting system, ACS Nano, 2020, 14, 7–11 CrossRef CAS PubMed.
- C. X. Li, Y. Zhang, X. Dong, L. Zhang, M. D. Liu, B. Li, M. K. Zhang, J. Feng and X. Z. Zhang, Artificially reprogrammed macrophages as tumor-tropic immunosuppression-resistant biologics to realize therapeutics production and immune activation, Adv. Mater., 2019, 31, 1807211 CrossRef PubMed.
- B. Ding, P. Zheng, P. Ma and J. Lin, Manganese oxide nanomaterials: synthesis, properties, and theranostic applications, Adv. Mater., 2020, 32, 1905823 CrossRef CAS PubMed.
- J. Wahsner, E. M. Gale, A. R. Rodriguez and P. Caravan, Chemistry of MRI contrast agents: current challenges and new frontiers, Chem. Rev., 2019, 119, 957–1057 CrossRef CAS PubMed.
- K. Zhu, Y. Ju, J. Xu, Z. Yang, S. Gao and Y. Hou, Magnetic nanomaterials: chemical design, synthesis, and potential applications, Acc. Chem. Res., 2018, 51, 404–413 CrossRef CAS PubMed.
- H. Lin, Y. Chen and J. Shi, Nanoparticle-triggered in situ catalytic chemical reactions for tumour-specific therapy, Chem. Soc. Rev., 2018, 47, 1938–1958 RSC.
- D. Jiang, D. Ni, Z. T. Rosenkrans, P. Huang, X. Yu and W. Cai, Nanozyme: new horizons for responsive biomedical applications, Chem. Soc. Rev., 2019, 48, 3683–3704 RSC.
- F. Gao, Y. Tan, W. Liu, M. Zou, C. Huang, C. Liu and X. Z. Zhang, Intra/extracellular lactic acid exhaustion for synergistic metabolic therapy and immunotherapy of tumors, Adv. Mater., 2019, 31, 1904639 CrossRef CAS PubMed.
- C. R. Domingo, A. Audige, S. Granja, W. C. Cheng, P. C. Ho, F. Baltazar, C. Stockmann and M. Mazzone, Immunity, hypoxia and metabolism – the ménage à trois of cancer: implications for immunotherapy, Physiol. Rev., 2020, 100, 1–102 CrossRef PubMed.
- Y. Nosaka and A. Y. Nosaka, Generation and detection of reactive oxygen species in photocatalysis, Chem. Rev., 2017, 117, 11302–11336 CrossRef CAS PubMed.
- H. Zhao, B. Zhao, L. Wu, H. Xiao, K. Ding, C. Zheng, Q. Song, L. Sun, L. Wang and Z. Zhang, Amplified cancer immunotherapy of a surface-engineered antigenic microparticle vaccine by synergistically modulating tumor microenvironment, ACS Nano, 2019, 13, 12553–12566 CrossRef CAS PubMed.
- Y. Dai, C. Xu, X. Sun and X. Chen, Nanoparticle design strategies for enhanced anticancer therapy by exploiting the tumour microenvironment, Chem. Soc. Rev., 2017, 46, 3830–3852 RSC.
- J. Xie, L. Gong, S. Zhu, Y. Yong, Z. Gu and Y. Zhao, Emerging strategies of nanomaterial-mediated tumor radiosensitization, Adv. Mater., 2018, 30, 1802244 Search PubMed.
- X. Wang, S. Song and H. Zhang, A redox interaction-engaged strategy for multicomponent nanomaterials, Chem. Soc. Rev., 2020, 49, 736–764 RSC.
- Y. Liu, Y. Jiang, M. Zhang, Z. Tang, M. He and W. Bu, Modulating hypoxia via nanomaterials chemistry for efficient treatment of solid tumors, Acc. Chem. Res., 2018, 51, 2502–2511 CrossRef CAS PubMed.
- Y. Shi and T. Lammers, Combining nanomedicine and immunotherapy, Acc. Chem. Res., 2019, 52, 1543–1554 CrossRef CAS PubMed.
- B. T. Mai, S. Fernandes, P. B. Balakrishnan and T. Pellegrino, Nanosystems based on magnetic nanoparticles and thermo- or pH responsive polymers: an update and future perspectives, Acc. Chem. Res., 2018, 51, 999–1013 CrossRef CAS PubMed.
- G. Yang, S. Z. F. Phua, A. K. Bindra and Y. Zhao, Degradability and clearance of inorganic nanoparticles for biomedical applications, Adv. Mater., 2019, 31, 1805730 CrossRef PubMed.
- N. Zhao, X. Lin, Q. Zhang, Z. Ji and F. J. Xu, Redox-triggered gatekeeper-enveloped starlike hollow silica nanoparticles
for intelligent delivery systems, Small, 2015, 11, 6467–6479 CrossRef CAS PubMed.
- X. Lin, N. Zhao, P. Yan, H. Hu and F. J. Xu, The shape and size effects of polycation functionalized silica nanoparticles on gene transfection, Acta Biomater., 2015, 11, 381–392 CrossRef CAS PubMed.
- P. Yan, N. Zhao, H. Hu, X. Lin, F. Liu and F. J. Xu, A facile strategy to functionalize gold nanorods with polycation brushes for biomedical applications, Acta Biomater., 2014, 10, 3786–3794 CrossRef CAS PubMed.
- X. Chen, Q. Zhang, J. Li, M. Yang, N. Zhao and F. J. Xu, Rattle-structured rough nanocapsules with in-situ-formed gold nanorod cores for complementary gene/chemo/photothermal therapy, ACS Nano, 2018, 12, 5646–5656 CrossRef CAS PubMed.
- H. Jiang, Q. Wang and X. Sun, Lymph node targeting strategies to improve vaccination effcacy, J. Controlled Release, 2017, 267, 47–56 CrossRef CAS PubMed.
- K. T. Gause, A. K. Wheatley, J. Cui, Y. Yan, S. J. Kent and F. Caruso, Immunological principles guiding the rational design of particles for vaccine delivery, ACS Nano, 2017, 11, 54–68 CrossRef CAS PubMed.
- A. Albanese, P. S. Tang and W. C. Chan, The effect of nanoparticle size, shape, and surface chemistry on biological systems, Annu. Rev. Biomed. Eng., 2012, 14, 1–16 CrossRef CAS PubMed.
- M. F. Bachmann and G. T. Jennings, Vaccine delivery: a matter of size, geometry, kinetics and molecular patterns, Nat. Rev. Immunol., 2010, 10, 787–796 CrossRef CAS PubMed.
- X. Li, B. R. Sloat, N. Yanasarn and Z. Cui, Relationship between the size of nanoparticles and their adjuvant activity: data from a study with an improved experimental design, Eur. J. Pharm. Biopharm., 2011, 78, 107–116 CrossRef CAS PubMed.
- Y. Fan and J. J. Moon, Nanoparticle drug delivery systems designed to improve cancer vaccines and immunotherapy, Vaccines, 2015, 3, 662–685 CrossRef CAS PubMed.
- Q. Zhou, Y. Zhang, J. Du, Y. Li, Y. Zhou, Q. Fu, J. Zhang, X. Wang and L. Zhan, Different-sized gold nanoparticle activator/antigen increases dendritic cells accumulation in liver-draining lymph nodes and CD8+ T cell responses, ACS Nano, 2016, 10, 2678–2692 CrossRef CAS PubMed.
- Y. Z. Zhang, J. Lazarovits, W. Poon, B. Quyang, L. N. M. Nguyen, B. R. Kingston and W. C. W. Chan, Nanoparticle size influences antigen retention and presentation in lymph node follicles for humoral immunity, Nano Lett., 2019, 19, 7226–7235 CrossRef CAS PubMed.
- S. E. Gratton, P. A. Ropp, P. D. Pohlhaus, J. C. Luft, V. J. Madden, M. E. Napier and J. M. DeSimone, The effect of particle design on cellular internalization pathways, Proc. Natl. Acad. Sci. U. S. A., 2008, 105, 11613–11618 CrossRef CAS PubMed.
- K. Niikura, T. Matsunaga, T. Suzuki, S. Kobayashi, H. Yamaguchi, Y. Orba, A. Kawaguchi, H. Hasegawa, K. Kajino, T. Ninomiya, K. Ijiro and H. Sawa, Gold nanoparticles as a vaccine platform: influence of size and shape on immunological responses in vitro and in vivo, ACS Nano, 2013, 7, 3926–3938 CrossRef CAS PubMed.
- P. L. Abbaraju, M. Jambhrunkar, Y. Yang, Y. Liu, Y. Lu and C. Yu, Asymmetric mesoporous silica nanoparticles as potent and safe immunoadjuvants provoke high immune responses, Chem. Commun., 2018, 54, 2020–2023 RSC.
- P. Cai, X. Zhang, M. Wang, Y. L. Wu and X. Chen, Combinatorial nano-bio interfaces, ACS Nano, 2018, 12, 5078–5084 CrossRef CAS PubMed.
- F. Chen, X. Zhuang, L. Lin, P. Yu, Y. Wang, Y. Shi, G. Hu and Y. Sun, New horizons in tumor microenvironment biology: challenges and opportunities, BMC Med., 2015, 13, 45 CrossRef PubMed.
- R. R. Langley and I. J. Fidler, The seed and soil hypothesis revisited the role of tumor-stroma interactions in metastasis to different organs, Int. J. Cancer, 2011, 128, 2527–2535 CrossRef CAS.
- L. Gu and D. J. Mooney, Biomaterials and emerging anticancer therapeutics: engineering the microenvironment, Nat. Rev. Cancer, 2016, 16, 56–66 CrossRef CAS PubMed.
- T. L. Whiteside, The tumor microenvironment and its role in promoting tumor growth, Oncogene, 2008, 27, 5904–5912 CrossRef CAS PubMed.
- K. Shimizu, T. Iyoda, M. Okada, S. Yamasaki and S. I. Fujii, Immune suppression and reversal of the suppressive tumor microenvironment, Int. Immunol., 2018, 30, 445–454 CrossRef CAS PubMed.
- D. Bayik, D. Tross and D. M. Klinman, Factors influencing the differentiation of human monocytic myeloid-derived suppressor cells into inflammatory macrophages, Front. Immunol., 2018, 9, 698 CrossRef PubMed.
- A. Mantovani, F. Marchesi, A. Malesci, L. Laghi and P. Allavena, Tumour-associated macrophages as treatment targets in oncology, Nat. Rev. Clin. Oncol., 2017, 14, 399–416 CrossRef CAS PubMed.
- L. Cassetta and J. W. Pollard, Targeting macrophages: therapeutic approaches in cancer, Nat. Rev. Drug Discovery, 2018, 17, 887–904 CrossRef CAS PubMed.
- S. Zanganeh, G. Hutter, R. Spitler, O. Lenkov, M. Mahmoudi, A. Shaw, J. S. Pajarinen, H. Nejadnik, S. Goodman, M. Moseley, L. M. Coussens and H. E. Daldrup-Link, Iron oxide nanoparticles inhibit tumour growth by inducing pro-inflammatory macrophage polarization in tumour tissues, Nat. Nanotechnol., 2016, 11, 986–994 CrossRef CAS PubMed.
- Z. Gu, T. Liu, J. Tang, Y. Yang, H. Song, Z. K. Tuong, J. Fu and C. Yu, Mechanism of iron oxide-induced macrophage activation: the impact of composition and the underlying signaling pathway, J. Am. Chem. Soc., 2019, 141, 6122–6126 CrossRef CAS PubMed.
- X. Liu, B. Yan, Y. Li, X. Ma, W. Jiao, K. Shi, T. Zhang, S. Chen, Y. He, X. Liang and H. Fan, Graphene oxide-grafted magnetic nanorings mediated magnetothermodynamic therapy favoring reactive oxygen species-related immune response for enhanced antitumor efficacy, ACS Nano, 2020, 14, 1936–1950 CrossRef CAS PubMed.
- G. Yang, L. Xu, Y. Chao, J. Xu, X. Sun, Y. Wu, R. Peng and Z. Liu, Hollow MnO2 as a tumor-microenvironment-responsive biodegradable nano-platform for combination therapy favoring antitumor immune responses, Nat. Commun., 2017, 8, 902 CrossRef PubMed.
- M. Song, T. Liu, C. Shi, X. Zhang and X. Chen, Bioconjugated manganese dioxide nanoparticles enhance chemotherapy response by priming tumor-associated macrophages toward M1-like phenotype and attenuating tumor hypoxia, ACS Nano, 2015, 10, 633–647 CrossRef PubMed.
- L. He, T. Nie, X. Xia, T. Liu, Y. Huang, X. Wang and T. Chen, Designing bioinspired 2D MoSe2 nanosheet for efficient photothermal-triggered cancer immunotherapy with reprogramming tumor-associated macrophages, Adv. Funct. Mater., 2019, 29, 1901240 CrossRef.
- Z. Lei, W. Zhu, S. Xu, J. Ding, J. Wan and P. Wu, Hydrophilic MoSe2 nanosheets as effective photothermal therapy agents and their application in smart devices, ACS Appl. Mater. Interfaces, 2016, 8, 20900–20908 CrossRef CAS PubMed.
- D. Li, M. Zhang, F. Xu, Y. Chen, B. Chen, Y. Chang, H. Zhong, H. Jin and Y. Huang, Biomimetic albumin-modified gold nanorods for photothermo-chemotherapy and macrophage polarization
modulation, Acta Pharm. Sin. B, 2018, 8, 74–84 CrossRef PubMed.
- Y. Zhang, X. Bush, B. Yan and J. A. Chen, Gemcitabine nanoparticles promote antitumor immunity against melanoma, Biomaterials, 2019, 189, 48–59 CrossRef CAS PubMed.
- R. Deng, M. Zou, D. Zheng, S. Peng, W. Liu, X. Bai, H. Chen, Y. Sun, P. Zhou and X. Zhang, Nanoparticles from cuttlefish ink inhibit tumor growth by synergizing immunotherapy and photothermal therapy, ACS Nano, 2019, 13, 8618–8629 CrossRef CAS PubMed.
- P. Chakraborty, S. Chatterjee, A. Ganguly, P. Saha, A. Adhikary, T. Das, M. Chatterjee and S. K. Choudhuri, Reprogramming of TAM toward proimmunogenic type through regulation of MAP kinases using a redox-active copper chelate, J. Leukocyte Biol., 2012, 91, 609–619 CrossRef CAS PubMed.
- S. Chatterjee, A. Mookerjee, J. M. Basu, P. Chakraborty, A. Ganguly, A. Adhikary, D. Mukhopadhyay, S. Ganguli, R. Banerjee, M. Ashraf, J. Biswas, P. K. Das, G. Sa, M. Chatterjee, T. Das and S. K. Choudhuri, A novel copper chelate modulates tumor associated macrophages to promote anti-tumor response of T cells, PLoS One, 2009, 4, e7048 CrossRef PubMed.
- N. Abbaspour, R. Hurrell and R. Kelishadi, Review on iron and its importance for human health, J. Res. Med. Sci., 2014, 19, 164–174 Search PubMed.
- K. Jomova and M. Valko, Importance of iron chelation in free radical-induced oxidative stress and human disease, Curr. Pharm. Des., 2011, 17, 3460–3473 CrossRef CAS PubMed.
- Y. Hu, S. Mignani, J. P. Majoral, M. Shen and X. Shi, Construction of iron oxide nanoparticle-based hybrid platforms for tumor imaging and therapy, Chem. Soc. Rev., 2018, 47, 1874–1900 RSC.
- C. Ansari, G. A. Tikhomirov, S. H. Hong, R. A. Falconer, P. M. Loadman, J. H. Gill, R. Castaneda, F. K. Hazard, L. Tong, O. D. Lenkov, D. W. Felsher, J. Rao and H. E. Daldrup-Link, Development of novel tumor-targeted theranostic nanoparticles activated by membrane-type matrix metalloproteinases for combined cancer magnetic resonance imaging and therapy, Small, 2014, 10, 566–575 CrossRef CAS PubMed.
- A. Tarangelo and S. J. Dixon, An iron age for cancer therapy, Nat. Nanotechnol., 2016, 11, 921–922 CrossRef CAS PubMed.
- S. Zhong, J. Xu, P. Li and H. Tsukamoto, Caveosomal oxidative stress causes Src-p21ras activation and lysine 63 TRAF6 protein polyubiquitination in iron-induced M1 hepatic macrophage activation, J. Biol. Chem., 2012, 287, 32078–32084 CrossRef CAS PubMed.
- A. Takaoka, H. Yanai, S. Kondo, G. Duncan, H. Negishi, T. Mizutani, S. Kano, K. Honda, Y. Ohba, T. W. Mak and T. Taniguchi, Integral role of IRF-5 in the gene induction programme activated by toll-like receptors, Nature, 2005, 434, 243–249 CrossRef CAS PubMed.
- Q. Chen, L. Feng, J. Liu, W. Zhu, Z. Dong, Y. Wu and Z. Liu, Intelligent albumin-MnO2 nanoparticles as pH-/H2O2-responsive dissociable nanocarriers to modulate tumor hypoxia for effective combination therapy, Adv. Mater., 2016, 28, 7129–7136 CrossRef CAS PubMed.
- P. Prasad, C. R. Gordijo, A. Z. Abbasi, A. Maeda, A. Ip, A. M. Rauth, R. S. DaCosta and X. Y. Wu, Multifunctional albumin-MnO2 nanoparticles modulate solid tumor microenvironment by attenuating hypoxia, acidosis, vascular endothelial growth factor and enhance radiation response, ACS Nano, 2014, 8, 3202–3212 CrossRef CAS PubMed.
- C. Zhang, L. Yan, Z. Gu and Y. Zhao, Strategies based on metal-based nanoparticles for hypoxic-tumor radiotherapy, Chem. Sci., 2019, 10, 6932–6943 RSC.
- Y. Wang, X. Li, Y. Mo, C. Fan, L. Tang, F. Xiong, C. Guo, B. Xiang, M. Zhou, J. Ma, X. Huang, X. Wu, Y. Li, G. Li, Z. Zeng and W. Xiong, Effects of tumor metabolic microenvironment on regulatory T cells, Mol. Cancer, 2018, 17, 115–168 CrossRef PubMed.
- V. L. Silva and W. T. Al-Jamal, Exploiting the cancer niche: tumor-associated macrophages and hypoxia as promising synergistic targets for nano-based therapy, J. Controlled Release, 2017, 253, 82–96 CrossRef CAS PubMed.
- K. Movahedi, S. Schoonooghe, D. Laoui, I. Houbracken, W. Waelput, K. Breckpot, L. Bouwens, T. Lahoutte, P. De Baetselier, G. Raes, N. Devoogdt and J. A. Van Ginderachter, Nanobody-based targeting of the macrophage mannose receptor for effective in vivo imaging of tumor-associated macrophages, Cancer Res., 2012, 72, 4165–4177 CrossRef CAS PubMed.
- A. M. K. Law, F. Valdes-Mora and D. Gallego-Ortega, Myeloid-derived suppressor cells as a therapeutic target for cancer, Cells, 2020, 9, 561 CrossRef PubMed.
- M. P. Plebanek, D. Bhaumik, P. J. Bryce and C. S. Thaxton, Scavenger receptor type B1 and lipoprotein nanoparticle inhibit myeloid-derived suppressor cells, Mol. Cancer Ther., 2018, 17, 686–697 CrossRef CAS PubMed.
- M. Kong, J. Tang, Q. Qiao, T. Wu, Y. Qi, S. Tan, X. Gao and Z. Zhang, Biodegradable hollow mesoporous silica nanoparticles for regulating tumor microenvironment and enhancing antitumor efficiency, Theranostics, 2017, 7, 3276–3292 CrossRef CAS PubMed.
- J. Martin-Liberal, M. O. D. Olza, C. Hierro, A. Gros, J. Rodon and J. Tabernero, The expanding role of immunotherapy, Cancer Treat. Rev., 2017, 54, 74–86 CrossRef CAS PubMed.
- Y. Yang, Cancer immunotherapy: harnessing the immune system to battle cancer, J. Clin. Invest., 2015, 125, 3335–3337 CrossRef PubMed.
- A. Sukari, M. Nagasaka, A. A. Hadidi and L. G. Lum, Cancer immunology and immunotherapy, Anticancer Res., 2016, 36, 5593–5606 CrossRef CAS PubMed.
- M. F. Sanmamed and L. Chen, A paradigm shift in cancer immunotherapy: from enhancement to normalization, Cell, 2018, 175, 313–326 CrossRef CAS PubMed.
- S. H. van der Burg, R. Arens, F. Ossendorp, T. van Hall and C. J. M. Melief, Vaccines for established cancer: overcoming the challenges posed by immune evasion, Nat. Rev. Cancer, 2016, 16, 219–233 CrossRef CAS PubMed.
- W. A. Li and D. J. Mooney, Materials based tumor immunotherapy vaccines, Curr. Opin. Immunol., 2013, 25, 238–245 CrossRef CAS PubMed.
- C. J. Melief, T. V. Hall, R. Arens, F. Ossendorp and S. H. Burg, Therapeutic cancer vaccines, J. Clin. Invest., 2015, 125, 3401–3412 CrossRef PubMed.
- H. G. Kelly, S. J. Kent and A. K. Wheatley, Immunological basis for enhanced immunity of nanoparticle vaccines, Expert Rev. Vaccines, 2019, 18, 269–280 CrossRef CAS PubMed.
- D. V. Krysko, A. D. Garg, A. Kaczmarek, O. Krysko, P. Agostinis and P. Vandenabeele, Immunogenic cell death and DAMPs in cancer therapy, Nat. Rev. Cancer, 2012, 12, 860–875 CrossRef CAS PubMed.
- O. Kepp, L. Senovilla, I. Vitale, E. Vacchelli, S. Adjemian, P. Agostinis, L. Apetoh, F. Aranda, V. Barnaba, N. Bloy, L. Bracci, K. Breckpot, D. Brough, A. Buqué, M. G. Castro, M. Cirone, M. I. Colombo, I. Cremer, S. Demaria, L. Dini, A. G. Eliopoulos, A. Faggioni, S. C. Formenti, J. Fučíková, L. Gabriele, U. S. Gaipl, J. Galon, A. Garg, F. Ghiringhelli, N. A. Giese, Z. S. Guo, A. Hemminki, M. Herrmann, J. W. Hodge, S. Holdenrieder, J. Honeychurch, H. Hu, X. Huang, T. M. Illidge, K. Kono, M. Korbelik, D. V. Krysko, S. Loi, P. R. Lowenstein, E. Lugli, Y. Ma, F. Madeo, A. A. Manfredi, I. Martins, D. Mavilio, L. Menger, N. Merendino, M. Michaud, G. Mignot, K. L. Mossman, G. Multhoff, R. Oehler, F. Palombo, T. Panaretakis, J. Pol, E. Proietti, J. Ricci, C. Riganti, P. Rovere-Querini, A. Rubartelli, A. Sistigu, M. J. Smyth, J. Sonnemann, R. Spisek, J. Stagg, A. Q. Sukkurwala, E. Tartour, A. Thorburn, S. H. Thorne, P. Vandenabeele, F. Velotti, S. T. Workenhe, H. Yang, W. Zong, L. Zitvogel, G. Kroemer and L. Galluzzi, Consensus guidelines for the detection of immunogenic cell death, OncoImmunology, 2014, 3, e955691 CrossRef PubMed.
- G. Kroemer, L. Galluzzi, O. Kepp and L. Zitvogel, Immunogenic cell death in cancer therapy, Annu. Rev. Immunol., 2013, 31, 51–72 CrossRef CAS PubMed.
- A. D. Garg and P. Agostinis, ER stress, Autophagy and immunogenic cell death in photodynamic therapy-induced anti-cancer immune responses, Photochem. Photobiol. Sci., 2014, 13, 474–487 RSC.
- Y. Li, X. Li, F. Zhou, A. Doughty, A. R. Hoover, R. E. Nordquist and W. R. Chen, Nanotechnology-based photoimmunological therapies for cancer, Cancer Lett., 2019, 442, 429–438 CrossRef CAS PubMed.
- Z. Zhou, J. Song, L. Nie and X. Chen, Reactive oxygen species generating systems meeting challenges of photodynamic cancer therapy, Chem. Soc. Rev., 2016, 45, 6597–6626 RSC.
- B. Hernández, Y. Yu, F. Ossendorp, M. Korbelik and S. Oliveira, Preclinical and clinical evidence of immune responses triggered in oncologic photodynamic therapy: clinical recommendations, J. Clin. Med., 2020, 9, 333 CrossRef PubMed.
- X. Yu, D. Gao, L. Gao, J. Lai, C. Zhang, Y. Zhao, L. Zhong, B. Jia, F. Wang, X. Chen and Z. Liu, Inhibiting metastasis and preventing tumor relapse by triggering host immunity with tumor-targeted photodynamic therapy using photosensitizer-loaded functional nanographenes, ACS Nano, 2017, 11, 10147–10158 CrossRef CAS PubMed.
- K. Lu, T. Aung, N. Guo, R. Weichselbaum and W. Lin, Nanoscale metal-organic frameworks for therapeutic, imaging, and sensing applications, Adv. Mater., 2018, 30, 1707634 CrossRef PubMed.
- K. Lu, C. He and W. Lin, Nanoscale metal–organic framework for highly effective photodynamic therapy of resistant head and neck cancer, J. Am. Chem. Soc., 2014, 136, 16712–16715 CrossRef CAS PubMed.
- K. Lu, C. He and W. Lin, A chlorin-based nanoscale metal–organic framework for photodynamic therapy of colon cancers, J. Am. Chem. Soc., 2015, 137, 7600–7603 CrossRef CAS PubMed.
- K. Lu, C. He, N. Guo, C. Chan, K. Ni, R. R. Weichselbaum and W. Lin, Chlorin-based nanoscale metal–organic framework systemically rejects colorectal cancers via synergistic photodynamic therapy and checkpoint blockade immunotherapy, J. Am. Chem. Soc., 2016, 138, 12502–12510 CrossRef CAS PubMed.
- G. Lan, K. Ni, Z. Xu, S. S. Veroneau, Y. Song and W. Lin, Nanoscale metal–organic framework overcomes hypoxia for photodynamic therapy primed cancer immunotherapy, J. Am. Chem. Soc., 2018, 140, 5670–5673 CrossRef CAS PubMed.
- C. Qian, J. Yu, Y. Chen, Q. Hu, X. Xiao, W. Sun, C. Wang, P. Feng, Q. D. Shen and Z. Gu, Light-activated hypoxia-responsive nanocarriers for enhanced anticancer therapy, Adv. Mater., 2016, 28, 3313–3320 CrossRef CAS.
- C. Zhang, W. Bu, D. Ni, S. Zhang, Q. Li, Z. Yao, J. Zhang, H. Yao, Z. Wang and J. Shi, Synthesis of iron nanometallic glasses and their application in cancer therapy by a localized Fenton reaction, Angew. Chem., Int. Ed., 2016, 55, 2101–2106 CrossRef CAS PubMed.
- H. Lee, H. Lee, D. L. Sedlak and C. Lee, pH-Dependent reactivity of oxidants formed by iron and copper-catalyzed decomposition of hydrogen peroxide, Chemosphere, 2013, 92, 652–658 CrossRef CAS.
- M. B. Gawande, A. Goswami, F. Felpin, T. Asefa, X. Huang, R. Silva, X. Zou, R. Zboril and R. S. Varma, Cu and Cu-based nanoparticles: synthesis and applications in catalysis, Chem. Rev., 2016, 116, 3722–3811 CrossRef CAS PubMed.
- T. Wang, H. Zhang, Y. Han, H. Liu, F. Ren, J. Zeng, Q. Sun, Z. Li and M. Gao, Light-enhanced O2-evolving nanoparticles boost photodynamic therapy to elicit antitumor immunity, ACS Appl. Mater. Interfaces, 2019, 11, 16367–16379 CrossRef CAS PubMed.
- A. Ito, M. Shinkai, H. Honda, K. Yoshikawa, S. Saga, T. Wakabayashi, J. Yoshida and T. Kobayashi, Heat shock protein 70 expression induces antitumor immunity during intracellular hyperthermia using magnetite nanoparticles, Cancer Immunol. Immunother., 2003, 52, 80–88 CrossRef CAS.
- A. Ito, H. Honda and T. Kobayashi, Cancer immunotherapy based on intracellular hyperthermia using magnetite nanoparticles: a novel concept of “heat-controlled necrosis” with heat shock protein expression, Cancer Immunol. Immunother., 2006, 55, 320–328 CrossRef CAS PubMed.
- L. Hou, Y. Yan, C. Tian, Q. Huang, X. Fu, Z. Zhang, H. Zhang, H. Zhang and Z. Zhang, Single-dose in situ storage for intensifying anticancer efficacy via combinatorial strategy, J. Controlled Release, 2020, 319, 438–449 CrossRef CAS PubMed.
- E. E. Sweeney, J. Cano-Mejia and R. Fernandes, Photothermal therapy generates a thermal window of immunogenic cell death in neuroblastoma, Small, 2018, 14, 1800678 CrossRef.
- X. Sun, L. Xing, C. C. Ling and G. C. Li, The effect of mild temperature hyperthermia on tumour hypoxia and blood perfusion: relevance for radiotherapy, vascular targeting and imaging, Int. J. Hyperthermia, 2010, 26, 224–231 CrossRef CAS PubMed.
- J. Dang, H. He, D. Chen and L. Yin, Manipulating tumor hypoxia toward enhanced photodynamic therapy (PDT), Biomater. Sci., 2017, 5, 1500–1511 RSC.
- Z. Wang, F. Zhang, D. Shao, Z. Chang, L. Wang, H. Hu, X. Zheng, X. Li, F. Chen, Z. Tu, M. Li, W. Sun, L. Chen and W. F. Dong, Janus nanobullets combine photodynamic therapy and magnetic hyperthermia to potentiate synergetic anti-metastatic immunotherapy, Adv. Sci., 2019, 6, 1901690 CrossRef CAS.
- M. Chang, Z. Hou, M. Wang, M. Wang, P. Dang, J. Liu, M. Shu, B. Ding, A. A. Kheraif, C. Li and J. Lin, Cu2MoS4/Au heterostructures with enhanced catalase-like activity and photoconversion efficiency for primary/metastatic tumors eradication by phototherapy-induced immunotherapy, Small, 2020, 16, 1907146 CrossRef CAS.
- W. Jiang, L. Yin, H. Chen, A. V. Paschall, L. Zhang, W. Fu, W. Zhang, T. Todd, K. S. Yu, S. Zhou, Z. Zhen, M. Butler, L. Yao, F. Zhang, Y. Shen, Z. Li, A. Yin, H. Yin, X. Wang, F. Y. Avci, X. Yu and J. Xie, NaCl nanoparticles as a cancer therapeutic, Adv. Mater., 2019, 31, 1904058 CrossRef CAS.
- K. A. Fitzgerald and J. C. Kagan, Toll-like receptors and the control of immunity, Cell, 2020, 180, 1044–1066 CrossRef CAS.
- M. A. Cheever, Twelve immunotherapy drugs that could cure cancers, Immunol. Rev., 2008, 222, 357–368 CrossRef CAS.
- A. Iwasaki and R. Medzhitov, Toll-like receptor control of the adaptive immune responses, Nat. Immunol., 2004, 5, 987–995 CrossRef CAS PubMed.
- A. M. Krieg, Therapeutic potential of Toll-like receptor 9 activation, Nat. Rev. Drug Discovery, 2006, 5, 471–484 CrossRef CAS PubMed.
- A. M. Krieg, CpG motifs in bacterial DNA and their immune effects, Annu. Rev. Immunol., 2002, 20, 709–760 CrossRef CAS PubMed.
- Q. Xia, C. Gong, F. Gu, Z. Wang, C. Hu, L. Zhang, L. Qiang, X. Ding, S. Gao and Y. Gao, Functionalized multi-walled carbon nanotubes for targeting delivery of immunostimulatory CpG oligonucleotides against prostate cancer, J. Biomed. Nanotechnol., 2018, 14, 1613–1626 CrossRef CAS PubMed.
- J. Ming, J. Zhang, Y. Shi, W. Yang, J. Li, D. Sun, S. Xiang, X. Chen, L. Chen and N. Zheng, A trustworthy CpG nanoplatform for highly safe and efficient cancer photothermal combined immunotherapy, Nanoscale, 2020, 12, 3916–3930 RSC.
- F. Heil, H. Hemmi, H. Hochrein, F. Ampenberger, C. Kirschning, S. Akira, G. Lipford, H. Wagner and S. Bauer, Species-specific recognition of single-stranded RNA via toll-like receptor 7 and 8, Science, 2004, 303, 1526–1529 CrossRef CAS PubMed.
- A. J. Grippin, B. Wummer, T. Wildes, K. Dyson, V. Trivedi, C. Yang, M. Sebastian, H. R. Mendez-Gomez, S. Padala, M. Grubb, M. Fillingim, A. Monsalve, E. J. Sayour, J. Dobson and D. A. Mitchell, Dendritic cell-activating magnetic nanoparticles enable early prediction of antitumor response with magnetic resonance imaging, ACS Nano, 2019, 13, 13884–13898 CrossRef CAS PubMed.
- Z. Hu, P. A. Ott and C. J. Wu, Towards personalized, tumour-specific, therapeutic vaccines for cancer, Nat. Rev. Immunol., 2018, 18, 168–182 CrossRef CAS PubMed.
- M. H. M. G. M. Brok, R. P. M. Sutmuller, R. Voort, E. J. Bennink, C. G. Figdor, T. J. M. Ruers and G. J. Adema, In situ tumor ablation creates an antigen source for the generation of antitumor immunity, Cancer Res., 2004, 64, 4024–4029 CrossRef PubMed.
- F. R. Carbone and M. J. Bevan, Induction of ovalbumin-specific cytotoxic T cells by in
vivo peptide immunization, J. Exp. Med., 1989, 169, 603–612 CrossRef CAS PubMed.
- K. Chamoto, T. Takeshima, D. Wakita, T. Ohkuri, S. Ashino, T. Omatsu, H. Shirato, H. Kitamura, Y. Togashi and T. Nishimura, Combination immunotherapy with radiation and CpG-based tumor vaccination for the eradication of radio- and immuno-resistant lung carcinoma cells, Cancer Sci., 2009, 100, 934–939 CrossRef CAS PubMed.
- P. Romero, J. Banchereau, N. Bhardwaj, M. Cockett, M. L. Disis, G. Dranoff, E. Gilboa, S. A. Hammond, R. Hershberg, A. J. Korman, P. Kvistborg, C. Melief, I. Mellman, A. K. Palucka, I. Redchenko, H. Robins, F. Sallusto, T. Schenkelberg, S. Schoenberger, J. Sosman, O. Tureci, B. V. Eynde, W. Koff and G. Coukos, The human vaccines project: a roadmap for cancer vaccine development, Sci. Transl. Med., 2016, 8, 334 Search PubMed.
- A. V. Kroll, R. H. Fang, Y. Jiang, J. Zhou, X. Wei, C. L. Yu, J. Gao, B. T. Luk, D. Dehaini, W. Gao and L. Zhang, Nanoparticulate delivery of cancer cell membrane elicits multiantigenic antitumor immunity, Adv. Mater., 2017, 29, 1703969 CrossRef PubMed.
- O. Joffre, M. A. Nolte, R. Sporri and E. S. C. Reis, Inflammatory signals in dendritic cell activation and the induction of adaptive immunity, Immunol. Rev., 2009, 227, 234–247 CrossRef CAS.
- X. Yan, M. Zhou, S. Yu, Z. Jin and K. Zhao, An overview of biodegradable nanomaterials and applications in vaccines, Vaccine, 2020, 38, 1096–1104 CrossRef CAS PubMed.
- J. Wang, H. Chen, T. Hang, Y. Yu, G. Liu, G. He, S. Xiao, B. Yang, C. Yang, F. Liu, J. Tao, M. X. Wu and X. Xie, Physical activation of innate immunity by spiky particles, Nat. Nanotechnol., 2018, 13, 1078–1086 CrossRef CAS PubMed.
- M. Hosseini, M. H. Fatahaliha, F. J. Niaragh, J. Majidi and M. Yousefi, The use of nanoparticles as a promising therapeutic approach in cancer immunotherapy, Artif. Cells, Nanomed., Biotechnol., 2016, 44, 1051–1061 CAS.
- T. L. Nguyen, Y. Choi and J. Kim, Mesoporous silica as a versatile platform for cancer immunotherapy, Adv. Mater., 2019, 31, 1803953 CrossRef PubMed.
- Y. Yang, Y. Lu, P. L. Abbaraju, J. Zhang, M. Zhang, G. Xiang and C. Yu, Multi-shelled dendritic mesoporous organosilica hollow spheres: roles of composition and architecture in cancer immunotherapy, Angew. Chem., Int. Ed., 2017, 56, 8446–8450 CrossRef CAS PubMed.
- B. Ding, S. Shao, C. Yu, B. Teng, M. Wang, Z. Cheng, K. Wong, P. Ma and J. Lin, Large-pore mesoporous-silica-coated upconversion nanoparticles as multifunctional immunoadjuvants with ultrahigh photosensitizer and antigen loading efficiency for improved cancer photodynamic immunotherapy, Adv. Mater., 2018, 30, 1802479 CrossRef PubMed.
- F. Fontana, M. Shahbazi, D. Liu, H. Zhang, E. Mäkilä, J. Salonen, J. T. Hirvonen and H. A. Santos, Multistaged nanovaccines based on porous silicon@acetalated dextran@cancer cell membrane for cancer immunotherapy, Adv. Mater., 2017, 29, 1603239 CrossRef PubMed.
- L. Luo, M. Z. Iqbal, C. Liu, J. Xing, O. U. Akakuru, Q. Fang, Z. Li, Y. Dai, A. Li, Y. Guan and A. Wu, Engineered nano-immunopotentiators efficiently promote cancer immunotherapy for inhibiting and preventing lung metastasis of melanoma, Biomaterials, 2019, 223, 119464 CrossRef CAS PubMed.
- P. M. Santos and L. H. Butterfield, Dendritic Cell-based cancer vaccines, J. Immunol., 2018, 200, 443–449 CrossRef CAS PubMed.
- A. Lanzavecchia and F. Sallusto, Antigen decoding by T lymphocytes: from synapses to fate determination, Nat. Immunol., 2001, 2, 487–492 CrossRef CAS PubMed.
- R. Yang, J. Xu, L. Xu, X. Sun, Q. Chen, Y. Zhao, R. Peng and Z. Liu, Cancer cell membrane-coated adjuvant nanoparticles with mannose modification for effective anticancer vaccination, ACS Nano, 2018, 12, 5121–5129 CrossRef CAS PubMed.
- W. Gao, R. H. Fang, S. Thamphiwatana, B. T. Luk, J. Li, P. Angsantikul, Q. Zhang, C. M. Hu and L. Zhang, Modulating antibacterial immunity via bacterial membrane-coated nanoparticles, Nano Lett., 2015, 15, 1403–1409 CrossRef CAS PubMed.
- D. Zhang, T. Wu, X. Qin, Q. Qiao, L. Shang, Q. Song, C. Yang and Z. Zhang, Intracellularly generated immunological gold nanoparticles for combinatorial photothermal therapy and immunotherapy against tumor, Nano Lett., 2019, 19, 6635–6646 CrossRef CAS PubMed.
- W. Liu, M. Zou, T. Liu, J. Zeng, X. Li, W. Yu, C. Li, J. Ye, W. Song, J. Feng and X. Zhang, Cytomembrane nanovaccines show therapeutic effects by mimicking tumor cells and antigen presenting cells, Nat. Commun., 2019, 10, 3199 CrossRef PubMed.
- J. Reiser and A. Banerjee, Effector, memory, and dysfunctional CD8(+) T cell fates in the antitumor immune response, J. Immunol. Res., 2016, 8941260 Search PubMed.
- J. Lei, H. Wang, D. Zhu, Y. Wan and L. Yin, Combined effects of avasimibe immunotherapy, doxorubicin chemotherapy, and metal-organic frameworks nanoparticles on breast cancer, J. Cell. Physiol., 2019, 235, 4814–4823 CrossRef PubMed.
- S. Feins, W. Kong, E. F. Williams, M. C. Milone and J. A. Fraietta, An introduction to chimeric antigen receptor (CAR) T-cell immunotherapy for human cancer, Am. J. Hematol., 2019, 94, S3–S9 CrossRef CAS PubMed.
- M. Sadelain, R. Brentjens and I. Rivière, The basic principles of chimeric antigen receptor design, Cancer Discovery, 2013, 3, 388–398 CrossRef CAS PubMed.
- P. Vormittag, R. Gunn, S. Ghorashian and F. S. Veraitch, A guide to manufacturing CAR T cell therapies, Curr. Opin. Biotechnol, 2018, 53, 164–181 CrossRef CAS PubMed.
- J. Guasch, C. A. Muth, J. Diemer, H. Riahinezhad and J. P. Spatz, Integrin-assisted T-cell activation on nanostructured hydrogels, Nano Lett., 2017, 17, 6110–6116 CrossRef CAS PubMed.
- J. Guasch, M. Hoffmann, J. Diemer, H. Riahinezhad, S. Neubauer, H. Kessler and J. P. Spatz, Combining adhesive nanostructured surfaces and costimulatory signals to increase T cell activation, Nano Lett., 2018, 18, 5899–5904 CrossRef CAS PubMed.
- F. S. Majedi, M. M. Hasani-Sadrabadi, T. J. Thauland, S. Li, L. Bouchard and M. J. Butte, Augmentation of T-cell activation by oscillatory forces and engineered antigen-presenting cells, Nano Lett., 2019, 19, 6945–6954 CrossRef CAS PubMed.
- W. Nie, W. Wei, L. Zuo, C. Lv, F. Zhang, G. Lu, F. Li, G. Wu, L. L. Huang, X. Xi and H. Xie, Magnetic nanoclusters armed with responsive PD-1 antibody synergistically improved adoptive T-cell therapy for solid tumors, ACS Nano, 2019, 13, 1469–1478 CrossRef CAS PubMed.
- Y. Liu, J. Guo and L. Huang, Modulation of tumor microenvironment for immunotherapy: focus on nanomaterial-based strategies, Theranostics, 2020, 10, 3099–3117 CrossRef PubMed.
- S. K. Wculek, F. J. Cueto, A. M. Mujal, I. Melero, M. F. Krummel and D. Sancho, Dendritic cells in cancer immunology and immunotherapy, Nat. Rev. Immunol., 2020, 20, 7–24 CrossRef CAS PubMed.
- M. S. Duthie, H. P. Windish, C. B. Fox and S. G. Reed, Use of defined TLR ligands as adjuvants within human vaccines, Immunol. Rev., 2011, 239, 178–196 CrossRef CAS PubMed.
- T. J. Moyer, A. C. Zmolek and D. J. Irvine, Beyond antigens and adjuvants: formulating future vaccines, J. Clin. Invest., 2016, 126, 799–808 CrossRef PubMed.
- P. Korangath, J. D. Barnett, A. Sharma, E. T. Henderson, J. Stewart, S. H. Yu, S. K. Kandala, C. T. Yang, J. S. Caserto, M. Hedayati, T. D. Armstrong, E. Jaffee, C. Gruettner, X. C. Zhou, W. Fu, C. Hu, S. Sukumar, B. W. Simons and R. Ivkov, Nanoparticle interactions with immune cells dominate tumor retention and induce T cell-mediated tumor suppression in models of breast cancer, Sci. Adv., 2020, 6, 1601 CrossRef PubMed.
- H. Vallhov, S. Gabrielsson, M. Strømme, A. Scheynius and A. E. Garcia-Bennett, Mesoporous silica particles induce size dependent effects on human dendritic cells, Nano Lett., 2007, 7, 3576–3582 CrossRef CAS PubMed.
- R. L. Sabado, S. Balan and N. Bhardwaj, Dendritic cell-based immunotherapy, Cell Res., 2017, 27, 74–95 CrossRef CAS PubMed.
- Y. Lu, Y. Yang, Z. Gu, J. Zhang, H. Song, G. Xiang and C. Yu, Glutathione-depletion mesoporous organosilica nanoparticles as a self-adjuvant and co-delivery platform for enhanced cancer immunotherapy, Biomaterials, 2018, 175, 82–92 CrossRef CAS PubMed.
- F. Duan, X. Feng, X. Yang, W. Sun, Y. Jin, H. Liu, K. Ge, Z. Li and J. Zhang, A simple and powerful co-delivery system based on pH-responsive metal-organic frameworks for enhanced cancer immunotherapy, Biomaterials, 2017, 122, 23–33 CrossRef CAS PubMed.
- H. Jiang, Q. Wang, L. Li, Q. Zeng, H. Li, T. Gong, Z. Zhang and X. Sun, Turning the old adjuvant from gel to nanoparticles to amplify CD8+ T cell responses, Adv. Sci., 2018, 5, 1700426 CrossRef PubMed.
- P. Zhang, Y. Chiu, L. H. Tostanoski and C. M. Jewell, Polyelectrolyte multilayers assembled entirely from immune signals on gold nanoparticle templates promote antigen-specific T cell response, ACS Nano, 2015, 9, 6465–6477 CrossRef CAS PubMed.
- A. Schlitzer, N. McGovern and F. Ginhoux, Dendritic cells and monocyte-derived cells: two complementary and integrated functional systems, Cell Dev. Biol., 2015, 41, 9–22 CAS.
- S. G. Reed, S. Bertholet, R. N. Coler and M. Friede, New horizons in adjuvants for vaccine development, Trends Immunol., 2009, 30, 23–32 CrossRef CAS PubMed.
- S. Gallucci, M. Lolkema and P. Matzinger, Natural adjuvants: endogenous activators of dendritic cells, Nat. Med., 1999, 5, 1249–1255 CrossRef CAS PubMed.
- P. L. Abbaraju, A. K. Meka, H. Song, Y. Yang, M. Jambhrunkar, J. Zhang, C. Xu, M. Yu and C. Yu, Asymmetric silica nanoparticles with tunable head-tail structures enhance hemocompatibility and maturation of immune cells, J. Am. Chem. Soc., 2017, 139, 6321–6328 CrossRef CAS PubMed.
- H. Wang, X. Pan, X. Wang, W. Wang, Z. Huang, K. Gu, S. Liu, F. Zhang, H. Shen, Q. Yuan, J. Ma, W. Yuan and H. Liu, Degradable carbon-silica nanocomposite with immunoadjuvant property for dual-modality photothermal/photodynamic therapy, ACS Nano, 2020, 14, 2847–2859 CrossRef CAS PubMed.
- T. R. Vargas, I. Benoit-Lizon and L. Apetoh, Rationale for stimulator of interferon genes–targeted cancer immunotherapy, Eur. J. Cancer, 2017, 75, 86–97 CrossRef PubMed.
- C. Wang, Y. Guan, M. Lv, R. Zhang, Z. Guo, X. Wei, X. Du, J. Yang, T. Li, Y. Wan, X. Su, X. Huang and Z. Jiang, Manganese increases the sensitivity of the cGAS-STING pathway for double-stranded DNA and is required for the host defense against DNA viruses, Immunity, 2018, 48, 675–687 CrossRef CAS PubMed.
- L. Hou, C. Tian, Y. Yan, L. Zhang, H. Zhang and Z. Zhang, Manganese-based nanoactivator optimizes cancer immunotherapy via enhancing innate immunity, ACS Nano, 2020, 14, 3927–3940 CrossRef CAS PubMed.
- J. A. Cintolo, J. Datta, S. J. Mathew and B. J. Czerniecki, Dendritic cell-based vaccines: barriers and opportunities, Future Oncol., 2012, 8, 1273–1299 CrossRef CAS PubMed.
- Y. Liu, Y. Han, H. Dong, X. Wei, D. Shi and Y. Li, Ca2+-mediated surface polydopamine engineering to program dendritic cell maturation, ACS Appl. Mater. Interfaces, 2019, 12, 4163–4173 CrossRef PubMed.
- W. Yue, L. Chen, L. Yu, B. Zhou, H. Yin, W. Ren, C. Liu, L. Guo, Y. Zhang, L. Sun, K. Zhang, H. Xu and Y. Chen, Checkpoint blockade and nanosonosensitizer augmented noninvasive sonodynamic therapy combination reduces tumour growth and metastases in mouse, Nat. Commun., 2019, 10, 2025 CrossRef PubMed.
- R. Ge, C. Liu, X. Zhang, W. Wang, B. Li, J. Liu, Y. Liu, H. Sun, D. Zhang, Y. Hou, H. Zhang and B. Yang, Photothermal-activatable Fe3O4 superparticle nanodrug carriers with PD-L1 immune checkpoint blockade for anti-metastatic cancer immunotherapy, ACS Appl. Mater. Interfaces, 2018, 10, 20342–20355 CrossRef CAS PubMed.
- L. Nuhn, S. D. Koker, S. V. Lint, Z. Zhong, J. P. Catani, F. Combes, K. Deswarte, Y. Li, B. N. Lambrecht, S. Lienenklaus, N. N. Sanders, S. A. David, J. Tavernier and B. G. Geest, Nanoparticle-conjugate TLR7/8 agonist localized immunotherapy provokes safe antitumoral responses, Adv. Mater., 2018, 30, 1803397 CrossRef PubMed.
- Y. Mi, C. Smith, F. Yang, Y. Qi, K. Roche, J. Serody, B. Vincent and A. Wang, A dual immunotherapy nanoparticle improves T-cell activation and cancer immunotherapy, Adv. Mater., 2018, 30, 1706098 CrossRef PubMed.
- H. Ruan, Q. Hu, D. Wen, Q. Chen, G. Chen, Y. Lu, J. Wang, H. Cheng, W. Lu and Z. Gu, A dual-bioresponsive drug-delivery depot for combination of epigenetic modulation and immune checkpoint blockade, Adv. Mater., 2019, 31, 1806957 CrossRef PubMed.
- Q. Chen, G. Chen, J. Chen, J. Shen, X. Zhang, J. Wang, A. Chan and Z. Gu, Bioresponsive protein complex of aPD1 and aCD47 antibodies for enhanced immunotherapy, Nano Lett., 2019, 19, 4879–4889 CrossRef CAS PubMed.
- X. Ye, X. Liang, Q. Chen, Q. Miao, X. Chen, X. Zhang and L. Mei, Surgical tumor-derived personalized photothermal vaccine formulation for cancer immunotherapy, ACS Nano, 2019, 13, 2956–2968 CrossRef CAS PubMed.
- Y. Q. Ye, J. Q. Wang, Q. Y. Hu, G. M. Hochu, H. L. Xin, C. Wang and Z. Gu, Synergistic transcutaneous immunotherapy enhances antitumor immune responses through delivery of checkpoint inhibitors, ACS Nano, 2016, 10, 8956–8963 CrossRef CAS PubMed.
- Y. K. Li, M. Fang, J. Zhang, J. Wang, Y. Song, J. Shi, W. Li, G. Wu, J. H. Ren, Z. Wang, W. P. Zou and L. Wang, Hydrogel dual delivered celecoxib and anti-PD-1 synergistically improve antitumor immunity, Oncoimmunology, 2016, 5, e1074374 CrossRef PubMed.
- Y. Chao, G. Chen, C. Liang, J. Xu, Z. Dong, X. Han, C. Wang and Z. Liu, Iron nanoparticles for low-power local magnetic hyperthermia in combination with immune checkpoint blockade for systemic antitumor therapy, Nano Lett., 2019, 19, 4287–4296 CrossRef CAS PubMed.
- Y. Chao, L. Xu, C. Liang, L. Feng, J. Xu, Z. Dong, L. Tian, X. Yi, K. Yang and Z. Liu, Combined local immunostimulatory radioisotope therapy and systemic immune checkpoint blockade imparts potent antitumour responses, Nat. Biomed. Eng., 2018, 2, 611–621 CrossRef CAS PubMed.
- X. Han, R. Wang, J. Xu, Q. Chen, C. Liang, J. Chen, J. Zhao, J. Chu, Q. Fan, E. Archibong, L. Jiang, C. Wang and Z. Liu, In situ thermal ablation of tumors in combination with nano-adjuvant and immune checkpoint blockade to inhibit cancer metastasis and recurrence, Biomaterials, 2019, 224, 119490 CrossRef CAS PubMed.
- L. Luo, C. Zhu, H. Yin, M. Jiang, J. Zhang, B. Qin, Z. Luo, X. Yuan, J. Yang, W. Li, Y. Du and J. You, Laser immunotherapy in combination with perdurable PD-1 blocking for the treatment of metastatic tumors, ACS Nano, 2018, 12, 7647–7662 CrossRef CAS PubMed.
- Z. Zhang, Q. Wang, Q. Liu, Y. Zheng, C. Zheng, K. Yi, Y. Zhao, Y. Gu, Y. Wang, C. Wang, X. Zhao, L. Shi, C. Kang and Y. Liu, Dual-locking nanoparticles disrupt the PD-1/PD-L1 pathway for efficient cancer immunotherapy, Adv. Mater., 2019, 31, 1905751 CrossRef CAS PubMed.
- M. A. Postow, R. Sidlow and M. D. Hellmann, Immune-related adverse events associated with immune checkpoint blockade, N. Engl. J. Med., 2018, 378, 158–168 CrossRef CAS PubMed.
- A. K. Kosmides, J. W. Sidhom, A. Fraser, C. A. Bessell and J. P. Schneck, Dual targeting nanoparticle stimulates the immune system to inhibit tumor growth, ACS Nano, 2017, 11, 5417–5429 CrossRef CAS PubMed.
- R. Meir, K. Shamalov, T. Sadan, M. Motiei, G. Yaari, C. J. Cohen and R. Popovtzer, Fast image-guided stratification using anti programmed death ligand 1 gold nanoparticles for cancer immunotherapy, ACS Nano, 2017, 11, 11127–11134 CrossRef CAS PubMed.
- Q. Chen, C. Wang, X. Zhang, G. Chen, Q. Hu, H. Li, J. Wang, D. Wen, Y. Zhang, Y. Lu, G. Yang, C. Jiang, J. Wang, G. Dotti and Z. Gu,
In situ sprayed bioresponsive immunotherapeutic gel for post-surgical cancer treatment, Nat. Nanotechnol., 2019, 14, 89–97 CrossRef CAS PubMed.
- C. Wang, L. Xu, C. Liang, J. Xiang, R. Peng and Z. Liu, Immunological responses triggered by photothermal therapy with carbon nanotubes in combination with anti-CTLA-4 therapy to inhibit cancer metastasis, Adv. Mater., 2014, 26, 8154–8162 CrossRef CAS PubMed.
- X. Dong, R. Cheng, S. Zhu, H. Liu, R. Zhou, C. Zhang, K. Chen, L. Mei, C. Wang, C. Su, X. Liu, Z. Gu and Y. Zhao, A heterojunction structured WO2.9-WSe2 nanoradiosensitizer increases local tumor ablation and checkpoint blockade immunotherapy upon low radiation dose, ACS Nano, 2020, 14, 5400–5416 CrossRef CAS PubMed.
- K. Ni, T. Luo, G. Lan, A. Culbert, Y. Song, T. Wu, X. Jiang and W. Lin, Nanoscale metal-organic frameworks mediate photodynamic therapy and deliver CpG oligodeoxynucleotides to enhance antigen presentation and cancer immunotherapy, Angew. Chem., Int. Ed., 2019, 59, 1108–1112 CrossRef PubMed.
- Y. Shao, B. Liu, Z. Di, G. Zhang, L. Sun, L. Li and C. Yan, Engineering of upconverted metal-organic frameworks for near-infrared light-triggered combinational photodynamic/chemo-/immunotherapy against hypoxic tumors, J. Am. Chem. Soc., 2020, 142, 3939–3946 CrossRef CAS PubMed.
- K. Ni, T. Aung, S. Li, N. Fatuzzo, X. Liang and W. Lin, Nanoscale metal-organic framework mediates radical therapy to enhance cancer immunotherapy, Chemistry, 2019, 5, 1892–1913 CrossRef CAS PubMed.
- J. Y. Zeng, M. Z. Zou, M. Zhang, X. S. Wang, X. Zeng, H. Cong and X. Z. Zhang, π-Extended benzoporphyrin-based metal-organic framework for inhibition of tumor metastasis, ACS Nano, 2018, 12, 4630–4640 CrossRef CAS PubMed.
- W. Yu, Y. Wang, J. Zhu, L. Jin, B. Liu, K. Xia, J. Wang, J. Gao, C. Liang and H. Tao, Autophagy inhibitor enhance ZnPc/BSA nanoparticle induced photodynamic therapy by suppressing PD-L1 expression in osteosarcoma immunotherapy, Biomaterials, 2019, 192, 128–139 CrossRef CAS PubMed.
- H. Liu, Y. Hu, Y. Sun, C. Wan, Z. Zhang, X. Dai, Z. Lin, Q. He, Z. Yang, P. Huang, Y. Xiong, J. Gao, X. Chen, Q. Chen, J. F. Lovell, Z. Xu, H. Jin and K. Yang, Co-delivery of bee venom melittin and a photosensitizer with an organic-inorganic hybrid nanocarrier for photodynamic therapy and immunotherapy, ACS Nano, 2019, 13, 12638–12652 CrossRef CAS PubMed.
- M. Chang, M. Wang, M. Wang, M. Shu, B. Ding, C. Li, M. Pang, S. Cui, Z. Hou and J. Lin, A multifunctional cascade bioreactor based on hollow structured Cu2MoS4 for synergetic cancer chemo-dynamic therapy/starvation therapy/phototherapy/immunotherapy with remarkably enhanced efficacy, Adv. Mater., 2019, 31, 1905271 CrossRef CAS PubMed.
- W. Xie, W. W. Deng, M. Zan, L. Rao, G. T. Yu, D. M. Zhu, W. T. Wu, B. Chen, L. W. Ji, L. Chen, K. Liu, S. S. Guo, H. M. Huang, W. F. Zhang, X. Zhao, Y. Yuan, W. Dong, Z. J. Sun and W. Liu, Cancer cell membrane camouflaged nanoparticles to realize starvation therapy together with checkpoint blockades for enhancing cancer therapy, ACS Nano, 2019, 13, 2849–2857 CrossRef CAS PubMed.
- J. Pan, P. Hu, Y. Guo, J. Hao, D. Ni, Y. Xu, Q. Bao, H. Yao, C. Wei, Q. Wu and J. Shi, Combined magnetic hyperthermia and immune therapy for primary and metastatic tumor treatments, ACS Nano, 2020, 14, 1033–1044 CrossRef CAS PubMed.
- K. Lu, C. He, N. Guo, C. Chan, K. Ni, G. Lan, H. Tang, C. Pelizzari, Y. Fu, M. T. Spiotto, R. R. Weichselbaum and W. Lin, Low-dose X-ray radiotherapy-radiodynamic therapy via nanoscale metal-organic frameworks enhance checkpoint blockade immunotherapy, Nat. Biomed. Eng., 2018, 2, 600–610 CrossRef CAS PubMed.
- K. Ni, G. Lan, C. Chan, B. Quigley, K. Lu, T. Aung, N. Guo, P. La Riviere, R. R. Weichselbaum and W. Lin, Nanoscale metal-organic frameworks enhance radiotherapy to potentiate checkpoint blockade immunotherapy, Nat. Commun., 2018, 9, 2351 CrossRef PubMed.
- H. Zhao, J. Xu, Y. Li, X. Guan, X. Han, Y. Xu, H. Zhou, R. Peng, J. Wang and Z. Liu, Nanoscale coordination polymer based nanovaccine for tumor immunotherapy, ACS Nano, 2019, 13, 13127–13135 CrossRef CAS PubMed.
- X. Han, S. Shen, Q. Fan, G. Chen, E. Archibong, G. Dotti, Z. Liu, Z. Gu and C. Wang, Red blood cell-derived nanoerythrosome for antigen delivery with enhanced cancer immunotherapy, Sci. Adv., 2019, 5, 6870 CrossRef PubMed.
- C. Sun, R. Mezzadra and T. N. Schumacher, Regulation and function of the PD-L1 checkpoint, Immunity, 2018, 48, 434–452 CrossRef CAS PubMed.
- Y. G. Bleyer and S. Ghosh, A novel link between inflammation and cancer, Caner Cell, 2016, 30, 829–830 CrossRef PubMed.
- S. Hameed, S. Mo, G. Mustafa, S. Z. Bajwa, W. S. Khan and Z. Dai, Immunological consequences of nanoparticle-mediated antitumor photoimmunotherapy, Adv. Ther., 2019, 2, 1900101 Search PubMed.
- Q. Chen, L. Xu, C. Liang, C. Wang, R. Peng and Z. Liu, Photothermal therapy with immune-adjuvant nanoparticles together with checkpoint blockade for effective cancer immunotherapy, Nat. Commun., 2016, 7, 13193 CrossRef CAS PubMed.
- Q. Cao, W. Wang, M. Zhou, Q. Huang, X. Wen, J. Zhao, S. Shi, K. Geng, F. Li, H. Hatakeyama, C. Xu, D. P. Worms, W. Peng, D. Zhou, A. K. Sood and C. Li, Induction of antitumor immunity in mouse by the combination of nanoparticle-based photothermolysis and anti-PD-1 checkpoint inhibition, J. Nanomed. Nanotechnol., 2020, 25, 102169 CrossRef CAS PubMed.
- J. Xu, L. Xu, C. Wang, R. Yang, Q. Zhang, X. Han, Z. Dong, W. Zhu, R. Peng and Z. Liu, Near-infrared-triggered photodynamic therapy with multitasking upconversion nanoparticles in combination with checkpoint blockade for immunotherapy of colorectal cancer, ACS Nano, 2017, 11, 4463–4474 CrossRef CAS PubMed.
- A. P. Acharya, M. Sinha, M. L. Ratay, X. Ding, S. C. Balmert, C. J. Workman, Y. Wang, D. A. A. Vignali and S. R. Little, Localized multi-component delivery platform generates local and systemic anti-tumor immunity, Adv. Funct. Mater., 2017, 27, 1604366 CrossRef.
- T. L. Nguyen, B. G. Cha, Y. Choi, J. Im and J. Kim, Injectable dual-scale mesoporous silica cancer vaccine enabling effcient delivery of antigen/adjuvant-loaded nanoparticles to dendritic cells recruited
in local macroporous scaffold, Biomaterials, 2020, 239, 119859 CrossRef CAS PubMed.
- C. S. Chiang, Y. J. Lin, R. Lee, Y. H. Lai, H. W. Cheng, C. H. Hsieh, W. C. Shyu and S. Y. Chen, Combination of fucoidan-based magnetic nanoparticles and immunomodulators enhances tumour-localized immunotherapy, Nat. Nanotechnol., 2018, 13, 746–754 CrossRef CAS PubMed.
- Y. Wang, L. Zhang, Z. Xu, L. Miao and L. Huang, mRNA vaccine with antigen-specific checkpoint bockade induces an enhanced immune response against established melanoma, Mol. Ther., 2018, 26, 420–434 CrossRef CAS PubMed.
- L. Chen, L. Zhou, C. Wang, Y. Han, Y. Lu, J. Liu, X. Hu, T. Yao, Y. Lin, S. Liang, S. Shi and C. Dong, Tumor-targeted drug and CpG delivery system for phototherapy and docetaxel-enhanced immunotherapy with polarization toward M1-type macrophages on triple negative breast cancers, Adv. Mater., 2019, 31, 1904997 CrossRef CAS PubMed.
Footnote |
† Both authors contributed equally to this work. |
|
This journal is © the Partner Organisations 2020 |