DOI:
10.1039/D0QM00201A
(Research Article)
Mater. Chem. Front., 2020,
4, 1764-1772
Synergy of PVP and ethanol to synthesize Ni3S4 quantum dots for high-performance asymmetric supercapacitors†
Received
1st April 2020
, Accepted 18th April 2020
First published on 20th April 2020
Abstract
Ni3S4 quantum dots (QDs) have great potential for supercapacitors due to their unique quantum effects, high specific surface area, high water solubility and good stability, but the current preparation process is cumbersome and toxic. Here, we highlight a facile and environmentally-friendly synthesis of Ni3S4 QDs for the first time by virtue of the synergy of polyvinylpyrrolidone (PVP) and ethanol. The synergistic mechanism was revealed by using XRD to investigate the effect of the synthesis solvent. When QDs were used as a supercapacitor electrode material they exhibited excellent electrochemical properties, and the specific capacitance at 1 A g−1 was 1440 F g−1. In addition, Ni3S4 QDs and activated carbon (AC) are assembled into a Ni3S4 QD//AC asymmetric supercapacitor (ASC), which delivered a maximum energy density of 60.4 W h kg−1. This work provides new ideas for the preparation of QDs and opens up new concepts for the synthesis of nickel sulfide.
1. Introduction
In the fields of mobile electronic devices and hybrid vehicles, the demand for energy and electricity has witnessed a continuous increase, which has triggered a boom in the research of electrode materials for advanced energy storage equipment, for instance, lithium-ion batteries and supercapacitors.1 Among various energy storage devices, lithium-ion batteries have greatly improved people's lives and have recently won the Nobel Prize.2,3 However, they suffer from low power density, while supercapacitors can provide high power in an instant, and also have a higher energy density than a conventional dielectric capacitor.4,5
It is well known that the capacitance and charge storage capacity of supercapacitors are highly dependent on the nature of the electrode material. Currently, various types of electrode materials have been studied, such as sulfides,6,7 oxides,8,9 hydroxides,10 polymers11 and carbon materials.12 Among various electrode materials, transition metal sulfides have been extensively studied due to their unique chemical and physical properties.13 Among them, Ni3S4 is favored by researchers for its higher theoretical specific capacitance and good rate performance.14 In addition, due to the rich sources and low price of Ni3S4, it can meet the increasing demand of energy storage systems. Although various morphologies of Ni3S4 have been studied as electrode materials for supercapacitors such as rose,15 polyhedron16 and composite materials,17 their performance needs to be further improved.
For supercapacitors, the structure and morphology of electrode materials have a very important influence on their capacitance performance. Increasing the specific surface area of the material can enhance the redox reaction between the active material and the electrolyte.18 With the dimensionality reduced to zero, the proportion of atoms exposed on the surface of the QDs increases. Because the surface atom coordination is insufficient, the surface atom activity increases. These highly active surface atoms have a significant effect on the properties of the QDs, such as high catalytic activity, and strong reduction and oxidation.19–21 Making nickel sulfide into QDs can improve the electrochemical performance, including increasing the specific surface area, improving the electrochemical activity, and giving more charge transport routes. Preparation of nickel sulfide QDs has been reported, which usually involves toxic solvents and requires complex nitrogen protection and injection, for example, NiS2 QDs synthesized in oleylamine, which demands a nitrogen atmosphere,22 and Ni3S4 QDs synthesized in ethylene glycol;23 NiS2 QD–C3N4 composite materials have also been synthesized, but this method cannot synthesize pure NiS2 QDs.24 Therefore, developing a simple and environmentally-friendly synthetic method will promote the application of nickel sulfide QDs in supercapacitors.
The particles reduce the mutual contact between themselves by electrostatically adsorbing a surfactant, thereby reducing the size of the product. Common neutral surfactant PVP has good biocompatibility, and is non-toxic and harmless and widely used in many fields. Because the unpaired electrons of the nitrogen atom in PVP participate in the pyrrole ring conjugated system, the electron cloud is biased toward the oxygen atom, so the nitrogen atom is weakly positively charged.25 Not only does the nitrogen atom weaken the formation of hydrogen bonds, but it can also adsorb on negatively charged materials. At the same time, due to the long molecular chain of PVP, it has a good dispersion effect on the adsorbed material.
To put the above proposal into practice, PVP-induced Ni3S4 QDs were synthesized in a facile and environmentally-friendly fashion in one step. A mixed solution of water and ethanol was used as the solvent without the need for nitrogen protection and injection. The prepared Ni3S4 QDs exhibited a high specific capacitance of 1440 F g−1 at 1 A g−1. In addition, the assembled Ni3S4//AC-ASC exhibited an energy density of 60.4 W h kg−1 at a power density of 400 W kg−1. In the end, the device was successfully used in practice, and an LED was lit. This work demonstrates that Ni3S4 QDs can be used as an excellent supercapacitor electrode material and provides a simple and environmentally friendly new method for preparing QDs.
2. Experimental
2.1. Synthesis of Ni3S4 QDs
The reagents used were of analytical grade and were used without treatment. 3 mmol NiCl2·6H2O, 4 mmol Na2S2O3·5H2O, 15 mmol urea, and 0.5 g PVP (K-30) were dissolved in 20 mL of distilled water and ultrasonically assisted to dissolve, and then 20 mL of absolute ethanol was added and stirred uniformly. The mixed solution was transferred to a 50 mL round bottom flask and magnetically stirred, and then heated in an oil bath at 120 °C for 6 h. The small amount of air remaining in the flask is negligible. After that, the flask was taken out and cooled in cold water. We added 80 mL of acetone to the product solution and let it stand for 8 h to allow the Ni3S4 QDs to precipitate naturally. Thereafter, the supernatant was slowly poured out, 20 mL of distilled water was added, and the precipitate was uniformly dispersed by ultrasonic waves. We added another 50 mL of acetone and let it stand for 8 h to precipitate. The Ni3S4 QDs were cleaned 5 times in this way, and finally placed in a 60 °C vacuum drying oven for 12 h (the product is labeled P1).
In order to explore the formation mechanism of Ni3S4 QDs, we changed the solvent (20 mL water and 20 mL ethanol) to 40 mL of water. The other reaction conditions remained unchanged, and the synthesized product was labeled as P2.
2.2. Material characterization
The crystal structure was determined by X-ray diffraction (XRD, 9 kW/SmartLab, Japan). The elemental valence state was analyzed by X-ray photoelectron spectroscopy (XPS, 250Xi/ESCALAB, USA). The morphological structure was obtained by transmission electron microscopy (TEM, JEM-2100, Japan) and scanning electron microscopy (SEM, HITACHI S-4800, Japan). The surface area and pore size distribution of the product are measured by BET (Autosorb-IQ, USA).
2.3. Electrochemical measurements
Preparation of the working electrode.
The prepared electrode material, acetylene black, and polytetrafluoroethylene (PTFE) were mixed at a mass ratio of 7
:
2
:
1 to prepare a film with a thickness of about 0.1 mm, and dried at 60 °C for 5 hours. The film was fixed on nickel foam with a tableting machine.26 The weight of active material is about 2 mg. Each electrode had a geometric surface area of about 1 cm2.
Electrochemical performance test.
We used the electrode material, AC (Vulcan XC-72 carbon, USA) and a Hg/HgO electrode as a working electrode, counter electrode and reference electrode, tested in 6 M KOH. Electrochemical testing was performed on a Chenhua CHI660E electrochemical workstation and cycling stability testing was performed on a LAND CT2001A.
The specific capacitance of the electrode material can be calculated based on eqn (1):27
|  | (1) |
where
C is the specific capacitance in F g
−1;
I is the discharge current in A g
−1;
t is the discharge time in s;
m is the mass of the electrode material in g; and Δ
V is the voltage difference in V.
The mass ratio of the positive and negative materials of the asymmetric capacitor is calculated by eqn (2) to ensure charge balance:28
| 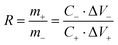 | (2) |
The following equation calculates the energy density (E) and power density (P):29
|  | (3) |
|  | (4) |
where
E is the energy density (W h kg
−1) and
P is the power density (W kg
−1).
3. Results and discussion
3.1. Structure and morphology
The as-obtained P1 (in water and ethanol mixed solvent) and P2 (in water medium) were firstly characterized by XRD, which is exhibited in Fig. 1a. Clearly, as compared to the standard PDF card, it can be seen that P1 is Ni3S4 and P2 is Ni2(OH)2(CO3). Among them, the peak of the XRD is broad due to the small size of the QDs. It's well known that Ni2+ (for example Ni(OH)2, NiCO3, NiO etc.) and S atoms are easily sulfided in the same system. However, the Ni2+ precursor with S2O32− reacting in water medium was not sulfided but yielded P2 Ni2(OH)2(CO3). Here, we proposed a reaction mechanism, which is shown in Fig. 1b. Given urea has a lower decomposition temperature than S2O32−, urea-decomposed CO32− and OH− would first combine with Ni2+ to form Ni2(OH)2(CO3). Afterwards, the surface of Ni2(OH)2(CO3) adsorbs O atoms of PVP through hydrogen bonding, hindering Ni2(OH)2(CO3) being sulfurized by S2O32−.30 By contrast, when ethanol was added to the solvent, ethanol and PVP formed hydrogen bonds, which blocked the adsorption between PVP and Ni2(OH)2(CO3) and facilitated the reaction between Ni2(OH)2(CO3) and S2O32−, finally resulting in Ni3S4. Also, the N atoms of PVP were electrostatically adsorbed on the surface of Ni3S4, which would prevent Ni3S4 agglomeration.31 Moreover, due to the steric hindrance effect of N atoms, Ni3S4 wrapped with nitrogen could confine Ni3S4 to be QDs. The chemical reaction equations involved were as follows:32,33 | CO(NH2)2 + 3H2O → 2NH4+ + CO2 + 2OH− | (R1) |
| CO2 + 2OH− → CO32− + H2O | (R2) |
| Ni2+ + CO32− + 2OH− → Ni2(OH)2(CO3) | (R3) |
| Ni2(OH)2(CO3) + 8S2O32− → 2NiS + 6SO42− + 8S + 2OH− + CO32− | (R4) |
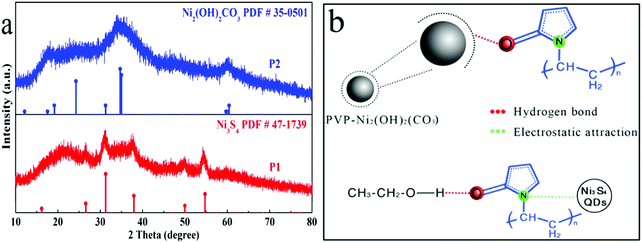 |
| Fig. 1 (a) XRD patterns of P1 and P2, and (b) schematic illustration of the synergy between PVP and ethanol. | |
XPS was used to detect the oxidation state of the elements of the Ni3S4 QDs. In the full scan spectrum of the sample (Fig. 2a), the elements Ni, S, C, N and O were detected. C, N and O were derived from air adsorption. The measured Ni/S atomic ratio was 3/4.4. In Fig. 2b, the binding energy of 856.3 eV and 874.1 eV indicated the presence of Ni2+, and the binding energy of 861.8 eV and 879.9 eV indicated the presence of Ni3+.34,35 For the XPS spectrum of S 2p (Fig. 2c), the binding energies at 161 eV and 162.5 eV suggested the presence of Ni–S chemical bonds and S2−. The binding energy at 168.7 eV was attributed to a high oxidation state of sulfur, such as S4O62−,36 which could be due to the surface oxidization of Ni3S4 QDs by air.
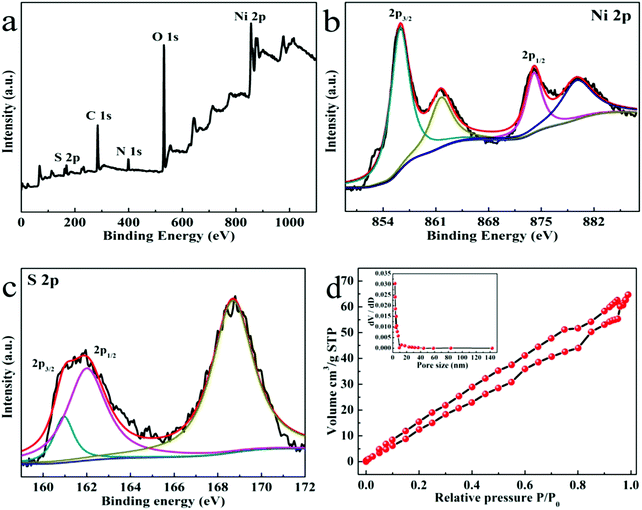 |
| Fig. 2 (a–c) XPS: full survey scan spectrum, Ni, and S, and (d) nitrogen adsorption/desorption isotherms and BJH pore size distribution curves for Ni3S4 QDs. | |
Since the surface area and pore size distribution will affect the performance of the supercapacitor, a larger specific surface area will provide more active sites, and an appropriate pore size will facilitate the transmission and diffusion of electrolyte ions. Therefore, Ni3S4 QDs were tested for the nitrogen adsorption–desorption and pore size distribution as exhibited in Fig. 2d, which showed that the isotherms of the Ni3S4 QDs were type IV of the IUPAC classification, promoting the diffusion and transport of electrolyte ions.37 The pore size distribution of the Ni3S4 QDs calculated by the BJH method shows that the pore size distribution was mainly below 5 nm. The measured BET surface area of the Ni3S4 QDs was 98.8 m2 g−1, and the average pore diameter was 4 nm, demonstrating the great advance of Ni3S4 QDs as electrode materials.
The morphology of Ni2(OH)2(CO3) revealed by SEM showed a porous structure (Fig. 3a) which was due to the adsorption of PVP on the surface of Ni2(OH)2(CO3) preventing the agglomeration of the material. As displayed in Fig. 3b of the TEM image, Ni3S4 QDs of about 2–3 nm were well-dispersed, which was further verified by the inset of Fig. 3b. As can be seen, Ni3S4 QDs in ethanol dispersions showed no precipitation after being left for 1 month, indicating that the QDs have good stability. The HRTEM image of the Ni3S4 QDs (Fig. 3c) showed recognizable lattice fringes, suggesting good crystallinity, which was consolidated by the SAED image (Fig. 3d). The atomic molar ratio of Ni and S in the Ni3S4 QDs measured using the EDS spectrum (Fig. 3e) is 42.5
:
57.5, which was also consistent with XPS and the chemical formula. In Fig. 3f and g, the EDS mapping of the Ni3S4 QDs clearly showed the uniform distribution of Ni and S elements. According to the above test results, it was proved that this method can effectively synthesize Ni3S4 QDs.
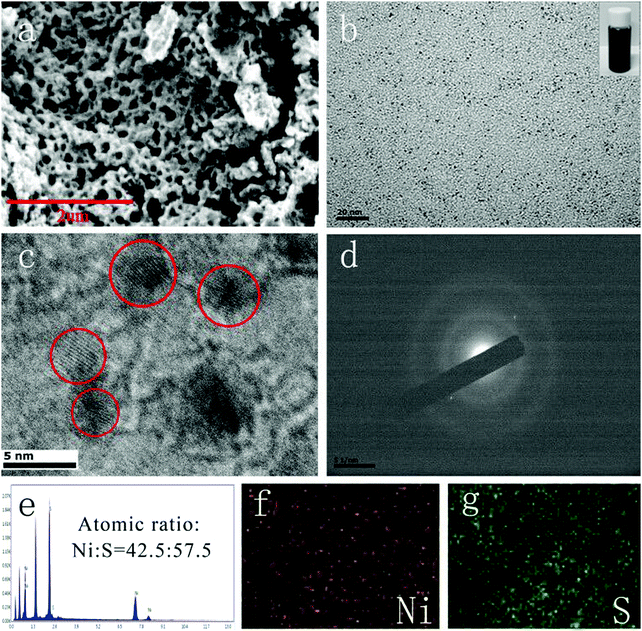 |
| Fig. 3 (a) SEM image of Ni2(OH)2(CO3); Ni3S4 QDs: (b) TEM image, (c) HRTEM image, (d) SAED image, (e) EDS image, and (f and g) mapping patterns. | |
3.2. Electrochemical properties of Ni3S4 QDs
The cyclic voltammetry (CV) curve of Fig. 4a shows the pseudocapacitor characteristics of the Ni3S4 QDs. At the same time, as the scan rate increased, the shape of the CV curve was basically unchanged, demonstrating the superior rate performance of the Ni3S4 QDs. According to the galvanostatic charge–discharge (GCD) curve of the Ni3S4 QDs (Fig. 4b), it can be seen that the Ni3S4 QD energy storage worked together with the electric double layer capacitance and the pseudocapacitance, but the specific capacitance contributed by the electric double layer capacitance was very small.38 The specific capacitance of the Ni3S4 QDs was calculated according to Fig. 4b and eqn (1). When the current density was 1, 3, 5, 8, 10, 15, 20, and 25 A g−1, the specific capacitance was 1440, 1213, 1085, 952, 882, 744, 628, and 500 F g−1. The specific capacitance decrease with the increase of the current density was attributed to the fact that there was not enough time for the redox reaction. Fig. 4c shows the electrochemical impedance spectroscopy (EIS) spectra of the Ni3S4 QDs, and the inset is the equivalent circuit diagram (frequency range: 1–105 Hz, potential amplitude: 5 mV). It can be seen from the figure that the charge transfer resistance of the Ni3S4 QDs was very small at only 0.71 Ω. The slope of the Ni3S4 QDs was the largest in the low frequency region, which indicated that the surface diffusion rate of the electrolyte on the QDs was fast due to the large specific surface area of the QDs.39Fig. 4d shows the cycle stability test results of the Ni3S4 QDs at 10 A g−1. As the number of cycles increased, the specific capacitance of the material gradually decreased. After 10k tests, the specific capacitance of the Ni3S4 QDs was maintained at 78% of the initial capacity, suggesting excellent cycle stability. Furthermore, the cycle performance of the prepared Ni3S4 QDs was compared with previously reported work as follows: CoNi2S4 (49%, 2000 cycles at 4 A g−1),40 Zn0.76Co0.24S (86.4%, 2000 cycles at 5 A g−1),41 CuCo2S4 (90.1%, 5000 cycles at 3 A g−1),42 NiS/CoS/NiCo2S4 (60%, 500 cycles at 5 mV s−1),43 and CoS (95%, 1300 cycles at 10 A g−1).44 The Ni3S4 QDs exhibited obvious advantages of a larger number of cycles and longer service life. According to the above analysis, PVP and ethanol induced Ni3S4 QDs are excellent electrode materials for supercapacitors.
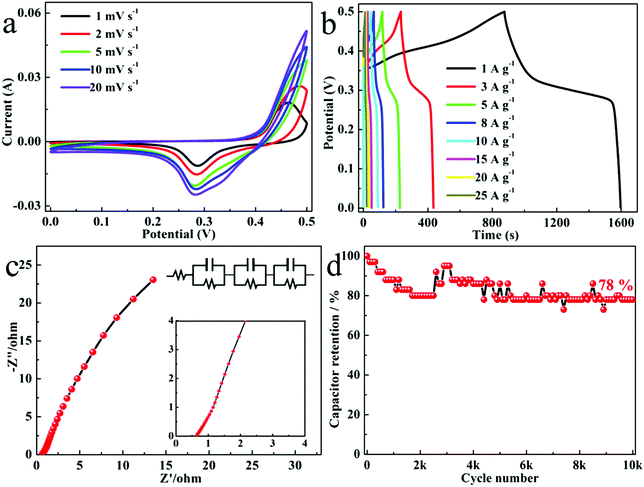 |
| Fig. 4 Ni3S4 QDs: (a) CV curve, (b) GCD curve, (c) Nyquist plot of EIS, and (d) cyclic stability. | |
3.3. Electrochemical performance of the Ni3S4 QD//AC-ASC
As is known, an ASC can help increase the voltage range and energy density. To make Ni3S4 QDs convenient for practical applications, a Ni3S4 QD//AC-ASC was assembled using Ni3S4 QDs and AC as the positive and negative electrodes, and tested in a 6 M KOH solution. AC (YEC-8A) was purchased from Fuzhou Yihuan Carbon Co., Ltd, China. The mass ratio of the positive electrode and the negative electrode was determined according to eqn (2). In fact, the essence of eqn (2) is charge balance theory and the calculated m(Ni3S4 QDs)
:
m(AC) = 1
:
2.2. As shown in Fig. 5a of the CV curves of the ASC in different voltage ranges, the ASC can stably expand to 1.6 V. When it was greater than 1.6 V, electrochemical polarization would occur, manifested by electrolyzed water and bubbles. Based on the non-rectangular CV curve of the ASC (Fig. 5b), it can be seen that the reactions are mainly redox reactions. When the scan rate increased, the shape of the CV curve didn’t change, indicating good rate performance. The symmetrical GCD curve (Fig. 5c) showed that the ASC had high Coulombic efficiency. As shown in Fig. 5d of the energy density and power density calculated from eqn (3) and (4) and the GCD curves, the ASC had a power density of 400 W kg−1 at an energy density of 60.4 W h kg−1. Even when the power density was 16 kW kg−1, the energy density can still maintain 23.6 W h kg−1. The performance of our assembled ASC devices was superior to many previously reported nickel sulfide ASCs, such as Ni3S4//AC,15 Ni3S4@rGO-20//rGO,16 Ni3S4-MoS2//AC,17 Ni3S4 QD/NF//AC/NF,23 TRGO//NCC2,32 NG/Ni-S-1//AC,45 Ni3S2@β-NiS//AC,46 Ni3S2@PPy//AC,47 and Co2+ doped Ni3S4//AC.48Fig. 5e shows the EIS spectrum of the ASC (frequency range: 1–105 Hz, potential amplitude: 5 mV), which showed that the equivalent series resistance was only 0.85 Ω, indicating that the internal resistance and charge transfer resistance of the ASC are very low, which is conducive to electron transport. In order to evaluate whether the ASC device can be used for a long time, we performed 10k constant current charge and discharge cycle tests under a voltage window of 1.6 V and a current density of 10 A g−1. As shown in Fig. 5f, the specific capacitance kept 76% of the initial value, indicating that the ASC has good cycle stability, which was compared with the previously reported work as follows: Ni3S4//AC (93%, 5000 cycles at 2 A g−1),15 TRGO//NCC2 (83%, 6000 cycles at 12 A g−1),32 3DNC@MnCo2S4//AC (82%, 5000 cycles at 10 A g−1),49 CuCo2S4/GA//AC (70.4%, 5000 cycles at 2 A g−1),50 NiCo2S4/GA//AC (71.6%, 5000 cycles at 2 A g−1),51 and ZnCo2S4//AC (98.7%, 2000 cycles at 6 A g−1).52 Two ASC devices connected in series can successfully illuminate an LED light, which can be well applied in practice. Considering the simple and environmentally-friendly synthesis method and its high energy density, our synthesis method holds great promise in the application of high energy storage devices.
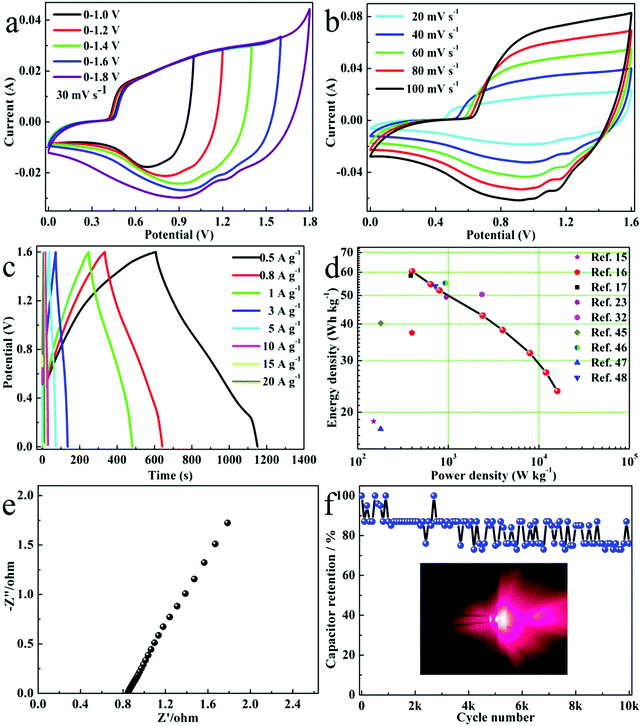 |
| Fig. 5 Ni3S4 QD//AC-ASC: (a) CV curve in different voltage ranges, (b) CV curve with different scan rates, (c) GCD curve, (d) relationship between the energy density and power density, (e) EIS spectrum and (f) cyclic stability. | |
4. Conclusions
In summary, by taking advantage of the synergy of PVP and ethanol, we facilely synthesized Ni3S4 QDs, during which the whole process not only did not require nitrogen protection but also was environmentally-friendly. In addition, insight into the synergistic mechanism was explored. When Ni3S4 QDs were used as the electrode material, they delivered a high specific capacitance of 1440 F g−1 at 1 A g−1 and superior cycle stability. The ASC with Ni3S4 QDs as the positive electrode and AC as the negative electrode showed a high energy density of 60.4 W h kg−1 at a power density of 400 W kg−1, which successfully drove an electronic device, demonstrating that the as-synthesized Ni3S4 QDs witnessed great advances for supercapacitors. This work provides a simple and environmentally-friendly method for designing metal-sulfide QDs as electrode materials.
Conflicts of interest
There are no conflicts to declare.
Acknowledgements
This work was supported by the National Natural Science Foundation of China (Grant No. 51771001, 21471001 and 21575001); Independent Research and Development Project of Anhui Province (201904a07020001); and the Open Project of Key Laboratory of Structure and Functional Regulation of Hybrid Materials (Anhui University), Ministry of Education.
References
- D.-W. Wang, F. Li and H.-M. Cheng, Hierarchical porous nickel oxide and carbon as electrode materials for asymmetric supercapacitor, J. Power Sources, 2008, 185, 1563–1568 CrossRef CAS.
- R. F. Service, Lithium-ion battery development takes Nobel, Science, 2019, 366, 292 CrossRef CAS PubMed.
- D. Castelvecchi and E. Stoye, Chemistry Nobel honours world-changing batteries, Nature, 2019, 574, 308 CrossRef CAS PubMed.
- X. Hu, Z. Deng, J. Suo and Z. Pan, A high rate, high capacity and long life (LiMn2O4 + AC)/Li4Ti5O12 hybrid battery–supercapacitor, J. Power Sources, 2009, 187, 635–639 CrossRef CAS.
- N. W. Duffy, W. Baldsing and A. G. Pandolfo, The nickel–carbon asymmetric supercapacitor—Performance, energy density and electrode mass ratios, Electrochim. Acta, 2008, 54, 535–539 CrossRef CAS.
- L.-l. Liu, K. P. Annamalai and Y.-s. Tao, A hierarchically porous CuCo2S4/graphene composite as an electrode material for supercapacitors, New Carbon Mater., 2016, 31, 336–342 CrossRef CAS.
- H. Tang, J. Wang, H. Yin, H. Zhao, D. Wang and Z. Tang, Growth of polypyrrole ultrathin films on MoS2 monolayers as high-performance supercapacitor electrodes, Adv. Mater., 2015, 27, 1117–1123 CrossRef CAS PubMed.
- R. BoopathiRaja, M. Parthibavarman and A. Nishara Begum, Hydrothermal induced novel CuCo2O4 electrode for high performance supercapacitor applications, Vacuum, 2019, 165, 96–104 CrossRef CAS.
- M. Chen, J. Wang, H. Tang, Y. Yang, B. Wang, H. Zhao and D. Wang, Synthesis of multi-shelled MnO2 hollow microspheres via an anion-adsorption process of hydrothermal intensification, Inorg. Chem. Front., 2016, 3, 1065–1070 RSC.
- J. Cao, Q. Mei, R. Wu and W. Wang, Flower-like nickel–cobalt layered hydroxide nanostructures for super long-life asymmetrical supercapacitors, Electrochim. Acta, 2019, 321, 134711 CrossRef CAS.
- D. A. L. Almeida and N. G. Ferreira, Fabrication of binary composites from polyaniline deposits on carbon fibers heat treated at three different temperatures: structural and electrochemical analyses for potential application in supercapacitors, Mater. Chem. Phys., 2020, 239, 122101 CrossRef CAS.
- A. M. Saleem, R. Andersson, V. Desmaris and P. Enoksson, Integrated on-chip solid state capacitor based on vertically aligned carbon nanofibers, grown using a CMOS temperature compatible process, Solid-State Electron., 2018, 139, 75–79 CrossRef CAS.
- J. Du, Q. Yan, Y. Li, K. Cheng, K. Ye, K. Zhu, J. Yan, D. Cao, X. Zhang and G. Wang, Hierarchical copper cobalt sulfides nanowire arrays for high-performance asymmetric supercapacitors, Appl. Surf. Sci., 2019, 487, 198–205 CrossRef CAS.
- M. Chuai, K. Zhang, X. Chen, Y. Tong, H. Zhang and M. Zhang, Effect of nondegeneracy on Ni3−xCoxS4 for high performance supercapacitor, Chem. Eng. J., 2020, 381, 122682 CrossRef CAS.
- H. Wang, M. Liang, D. Duan, W. Shi, Y. Song and Z. Sun, Rose-like Ni3S4 as battery-type electrode for hybrid supercapacitor with excellent charge storage performance, Chem. Eng. J., 2018, 350, 523–533 CrossRef CAS.
- Q. Hu, X. Zou, Y. Huang, Y. Wei, Ya Wang, F. Chen, B. Xiang, Q. Wu and W. Li, Graphene oxide-drove transformation of NiS/Ni3S4 microbars towards Ni3S4 polyhedrons for supercapacitor, J. Colloid Interface Sci., 2019, 559, 115–123 CrossRef PubMed.
- W. Luo, G. Zhang, Y. Cui, Y. Sun, Q. Qin, J. Zhang and W. Zheng, One-step extended strategy for the ionic liquid-assisted synthesis of Ni3S4–MoS2 heterojunction electrodes
for supercapacitors, J. Mater. Chem. A, 2017, 5, 11278–11285 RSC.
- J. Wang, H. Tang, H. Ren, R. Yu, J. Qi, D. Mao, H. Zhao and D. Wang, pH-Regulated Synthesis of Multi-Shelled Manganese Oxide Hollow Microspheres as Supercapacitor Electrodes Using Carbonaceous Microspheres as Templates, Adv. Sci., 2014, 1, 1400011 CrossRef PubMed.
- L. Tan, Z. Su, R. Yang, J. Tao, D. Zhao, Z. Zhang and F. Wen, Oxygen evolution catalytic performance of quantum dot nickel-iron double hydroxide/reduced graphene oxide composites, Mater. Lett., 2018, 231, 24–27 CrossRef CAS.
- F. Hasanpour, M. Nekoeinia, A. Semnani and S. Shojaei, NiMnO3 nanoparticles anchored on graphene quantum dot: Application in sensitive electroanalysis of dobutamine, Microchem. J., 2018, 142, 17–23 CrossRef CAS.
- T. K. Nideep, M. Ramya, V. P. N. Nampoori and M. Kailasnath, The size dependent thermal diffusivity of water soluble CdTe quantum dots using dual beam thermal lens spectroscopy, Phys. E, 2020, 116, 113724 CrossRef CAS.
- W. Chen, X. Zhang, L.-E. Mo, Z. Feng, S. Chen, X. Zhang, Y. Zhang and L. Hu, Ligands induced NiS2 quantum dots for synchronous high specific capacity and robust stability of advanced electrochemical energy storage, Chem. Eng. J., 2019, 375, 121981 CrossRef CAS.
- W. Chen, X. Zhang, Y. Peng, L.-E. Mo, Z. Li, Y. Zhang, X. Zhang and L. Hu, One-pot scalable synthesis of pure phase Ni3S4 quantum dots as a versatile electrode for high performance hybrid supercapacitors and lithium ion batteries, J. Power Sources, 2019, 438, 227004 CrossRef CAS.
- F. Xue, M. Liu, C. Cheng, J. Deng and J. Shi, Localized NiS2 Quantum Dots on g-C3N4 Nanosheets for Efficient Photocatalytic Hydrogen Production from Water, ChemCatChem, 2018, 10, 5441–5448 CrossRef CAS.
- N. K. Toru Takagishi, Interaction of Polyvinylpyrrolidone with Methyl Orange and Its Homologs in Aqueous Solution: Thermodynamics of the Binding Equilibria and Their Temperature Dependences, J. Polym. Sci., Polym. Chem. Ed., 1973, 11, 1889–1900 CrossRef.
- H. Wang, J. Yan, R. Wang, S. Li, D. J. L. Brett, J. Key and S. Ji, Toward high practical capacitance of Ni(OH)2 using highly conductive CoB nanochain supports, J. Mater. Chem. A, 2017, 5, 92–96 RSC.
- H. T. T. Thanh, P. A. Le, M. D. Thi, T. Le Quang and T. N. Trinh, Effect of gel polymer electrolyte based on polyvinyl alcohol/polyethylene oxide blend and sodium salts on the performance of solid-state supercapacitor, Bull. Mater. Sci., 2018, 41, 145 CrossRef.
- B. J. Reddy, P. Vickraman and A. S. Justin, Asymmetric supercapacitor device performance based on microwave synthesis of N-doped graphene/nickel sulfide nanocomposite, J. Mater. Sci., 2019, 54, 6361–6373 CrossRef CAS.
- U. Evariste, G. Jiang, B. Yu, Y. Liu and P. Ma, Electrodeposition of Manganese-Nickel-Cobalt Sulfides on Reduced Graphene Oxide/Nickel Foam for High-Performance Asymmetric Supercapacitors, J. Electron. Mater., 2020, 49(2), 922–930 CrossRef CAS.
- G. Wang, Y. Ma, J. Mu, Z. Zhang, X. Zhang, L. Zhang, H. Che, Y. Bai, J. Hou and H. Xie, Monodisperse polyvinylpyrrolidone-coated CoFe2O4 nanoparticles: Synthesis, characterization and cytotoxicity study, Appl. Surf. Sci., 2016, 365, 114–119 CrossRef CAS.
- S. Kumari, A. A. Khan, A. Chowdhury, A. K. Bhakta, Z. Mekhalif and S. Hussain, Efficient and highly selective adsorption of cationic dyes and removal of ciprofloxacin antibiotic by surface modified nickel sulfide nanomaterials: Kinetics, isotherm and adsorption mechanism, Colloids Surf., A, 2019, 124264 Search PubMed.
- S. Ghosh, J. Sharath Kumar, N. Chandra Murmu, R. Sankar Ganesh, H. Inokawa and T. Kuila, Development of carbon coated NiS2 as positive electrode material for high performance asymmetric supercapacitor, Composites, Part B, 2019, 177, 107373 CrossRef CAS.
- S. Liu, K. S. Hui, K. N. Hui, V. V. Jadhav, Q. X. Xia, J. M. Yun, Y. R. Cho, R. S. Mane and K. H. Kim, Facile Synthesis of Microsphere Copper Cobalt Carbonate Hydroxides Electrode for Asymmetric Supercapacitor, Electrochim. Acta, 2016, 188, 898–908 CrossRef CAS.
- F. Chen, H. Wang, S. Ji, V. Linkov and R. Wang, Core–shell structured Ni3S2@Co(OH)2 nano-wires grown on Ni foam as binder-free electrode for asymmetric supercapacitors, Chem. Eng. J., 2018, 345, 48–57 CrossRef CAS.
- Y. Shen, K. Zhang, B. Chen, F. Yang, K. Xu and X. Lu, Enhancing the electrochemical performance of nickel cobalt sulfides hollow nanospheres by structural modulation for asymmetric supercapacitors, J. Colloid Interface Sci., 2019, 557, 135–143 CrossRef CAS PubMed.
- F. Wang, J. Zheng, J. Ma, K. Zhou and Q. Wang, One-step facile route to glucose/copper cobalt sulfide nanorod for high-performance asymmetric supercapacitors, J. Nanopart. Res., 2019, 21, 189 CrossRef.
- B. Duan, X. Gao, X. Yao, Y. Fang, L. Huang, J. Zhou and L. Zhang, Unique elastic N-doped carbon nanofibrous microspheres with hierarchical porosity derived from renewable chitin for high rate supercapacitors, Nano Energy, 2016, 27, 482–491 CrossRef CAS.
- L. Xie, Z. Hu, C. Lv, G. Sun, J. Wang, Y. Li, H. He, J. Wang and K. Li, CoxNi1−x double hydroxide nanoparticles with ultrahigh specific capacitances as supercapacitor electrode materials, Electrochim. Acta, 2012, 78, 205–211 CrossRef CAS.
- T. K. Enock, C. K. King’ondu, A. Pogrebnoi and Y. A. C. Jande, Biogas-slurry derived mesoporous carbon for supercapacitor applications, Mater. Today Energy, 2017, 5, 126–137 CrossRef.
- W. Du, Z. Zhu, Y. Wang, J. Liu, W. Yang, X. Qian and H. Pang, One-step synthesis of CoNi2S4 nanoparticles for supercapacitor electrodes, RSC Adv., 2014, 4, 6998 RSC.
- J. Yang, Y. Zhang, C. Sun, G. Guo, W. Sun, W. Huang, Q. Yan and X. Dong, Controlled synthesis of zinc cobalt sulfide nanostructures in oil phase and their potential applications in electrochemical energy storage, J. Mater. Chem. A, 2015, 3, 11462–11470 RSC.
- Y. Zhu, X. Ji, H. Chen, L. Xi, W. Gong and Y. Liu, The investigation of the electrochemically supercapacitive performances of mesoporous CuCo2S4, RSC Adv., 2016, 6, 84236–84241 RSC.
- D. Li, X. Zhang, L. Pei, C. Dong, J. Shi and Y. Xu, High-performance supercapacitors and non-enzymatic electrochemical glucose sensor based on tremella-like NiS/CoS/NiCo2S4 hierarchical structure, Inorg. Chem. Commun., 2019, 110, 107581 CrossRef CAS.
- J. Li, D. Chen and Q. Wu, Facile synthesis of CoS porous nanoflake for high performance supercapacitor electrode materials, J. Energy Storage, 2019, 23, 511–514 CrossRef.
- Y. Luo, W. Que, C. Yang, Y. Tian, Y. Yang and X. Yin, Nitrogen-doped graphene/multiphase nickel sulfides obtained by Ni-C3N3S3 (metallopolymer) assisted synthesis for high-performance hybrid supercapacitors, Electrochim. Acta, 2019, 301, 332–341 CrossRef CAS.
- W. Li, S. Wang, L. Xin, M. Wu and X. Lou, Single-crystal β-NiS nanorod arrays with a hollow-structured Ni3S2 framework for supercapacitor applications, J. Mater. Chem. A, 2016, 4, 7700–7709 RSC.
- L. Long, Y. Yao, M. Yan, H. Wang, G. Zhang, M. Kong, L. Yang, X. Liao, G. Yin and Z. Huang, Ni3S2@polypyrrole composite supported on nickel foam with improved rate capability and cycling durability for asymmetric supercapacitor device applications, J. Mater. Sci., 2016, 52, 3642–3656 CrossRef.
- Y. Li, J. Li, M. Wang, Y. Liu and H. Cui, High rate performance and stabilized cycle life of Co2+-doped nickel sulfide nanosheets synthesized by a scalable method of solid-state reaction, Chem. Eng. J., 2019, 366, 33–40 CrossRef CAS.
- M. Hua, F. Cui, Y. Huang, Y. Zhao, J. Lian, J. Bao, B. Zhang, S. Yuan and H. Li, Crafting nanosheet-built MnCo2S4 disks on robust N-doped carbon matrix for hybrid supercapacitors, Electrochim. Acta, 2019, 323, 134770 CrossRef CAS.
- Z. Tian, X. Wang, B. Li, H. Li and Y. Wu, High rate capability electrode constructed by anchoring CuCo2S4 on graphene aerogel skeleton toward quasi-solid-state supercapacitor, Electrochim. Acta, 2019, 298, 321–329 CrossRef CAS.
- B. Li, Z. Tian, H. Li, Z. Yang, Y. Wang and X. Wang, Self-supporting graphene aerogel electrode intensified by NiCo2S4 nanoparticles for asymmetric supercapacitor, Electrochim. Acta, 2019, 314, 32–39 CrossRef CAS.
- C. Cheng, X. Zhang, C. Wei, Y. Liu, C. Cui, Q. Zhang and D. Zhang, Mesoporous hollow ZnCo2S4 core–shell nanospheres for high performance supercapacitors, Ceram. Int., 2018, 44, 17464–17472 CrossRef CAS.
Footnote |
† Electronic supplementary information (ESI) available. See DOI: 10.1039/d0qm00201a |
|
This journal is © the Partner Organisations 2020 |
Click here to see how this site uses Cookies. View our privacy policy here.