DOI:
10.1039/C9QM00658C
(Review Article)
Mater. Chem. Front., 2020,
4, 821-836
Carbon dots: a booming material for biomedical applications
Received
24th October 2019
, Accepted 12th December 2019
First published on 14th December 2019
Abstract
As one of the most promising nanomaterials for biomedical applications, carbon dots (CDs) hold great potential in the field of bioimaging, biosensing and biotherapy due to their low cytotoxicity, high water solubility, favorable biocompatibility, good photostability, tunable fluorescence emission and excitation. In this review, we will provide an update on the latest research of CDs on the synthetic routes, chemical modifications, optical properties and biomedical applications. We will mainly discuss their applications in bioimaging of normal and cancer stem cells and tumour cells, two-photon fluorescence imaging, in vivo imaging, biosensing, and cancer therapy including photothermal therapy, photodynamic therapy and chemotherapy. At the end, current challenges and future perspectives of CDs in biomedical applications will also be discussed. We hope that this review will provide valuable insights to inspire new discoveries on CDs and draw a roadmap towards a broader range of biomedical applications.
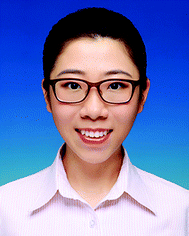
Wen Su
| Wen Su received her Master's degree in analytical chemistry from Beijing Normal University in 2017. Currently, she is a PhD student under the supervision of Prof. Louzhen Fan at Beijing Normal University. Her research interests focus on the synthesis and biomedical applications of fluorescent carbon nanomaterials. |
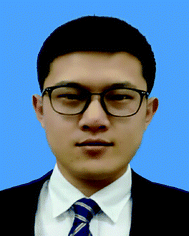
Hao Wu
| Hao Wu received his Master's degree in applied chemistry in 2018 at Northeast Agricultural University. After graduation, he continued to pursue his PhD under the supervision of Prof. Louzhen Fan at Beijing Normal University. His research interests focus on the synthesis and biomedical applications of carbon quantum dots. |
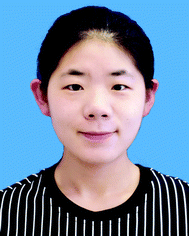
Huimin Xu
| Huimin Xu obtained her BS degree in Chemistry from Shanxi Normal University in 2019. Now, she is a Master's student under the supervision of Prof. Louzhen Fan at Beijing Normal University. Her research interests focus on the synthesis and biomedical applications of carbon quantum dots. |
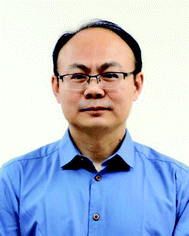
Yunchao Li
| Yunchao Li is now a chemistry professor of Department of Chemistry, Beijing Normal University. He received his PhD in physical chemistry in 2005 under the supervision of Prof. Yongfang Li from Institute of Chemistry, the Chinese Academy of Sciences, Beijing. His research interests are focused on the synthesis of new nanomaterials and design of new biosensors. |
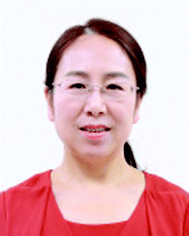
Xiaohong Li
| Xiaohong Li is now a chemistry professor of Department of Chemistry, Beijing Normal University. She received her PhD in physical chemistry in 2004 under the supervision of Prof. Yongfang Li from Institute of Chemistry, the Chinese Academy of Sciences, Beijing. Her research interests are focused on biosensors for environmental pollutants. |
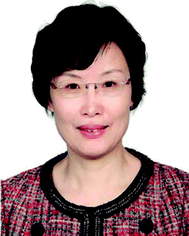
Louzhen Fan
| Louzhen Fan is now a chemistry professor of Department of Chemistry, Beijing Normal University. She received her PhD in physical chemistry in 1998 under the supervision of Prof. Daoben Zhu and Prof. Yongfang Li from Institute of Chemistry, the Chinese Academy of Sciences, Beijing. Her research interests are focused on the synthesis of novel carbon and metal nanomaterials and their applications in biology and energy. |
1. Introduction
Carbon dots (CDs) typically refer to a fascinating type of zero-dimensional (0D) carbon nanomaterials with lateral sizes below 10 nm and a lattice spacing of 0.21 and 0.34 nm corresponding to the (100) and (002) lattice planes of graphite.1,2 Graphene quantum dots (GQDs) and carbon quantum dots (CQDs) are two classic types of CDs. GQDs are specially small graphene fragments consisting of single- or few-layer graphene sheets, while CQDs always possess obvious crystal lattices, demonstrating intrinsic state luminescence and the quantum confinement effect.3 It has significant meaning to regulate the wavelength of photoluminescence (PL) through the control of the CQD size.4 The first discovery of fluorescent carbon nanoparticles could be traced to 2004 during the purification of single walled carbon nanotubes by Scrivens and co-workers.5 In 2006, these quantum-sized nanoparticles with intense photoluminescence in both solution and the solid state were first coined as “carbon dots” by Sun and co-workers.6 Since then, considerable attention has been focused on the biomedical applications of CDs due to their low cytotoxicity, high water solubility, favorable biocompatibility, good photostability, high sensitivity and selectivity for target analytes, tunable fluorescence emission and excitation, high quantum yields, and relatively large Stokes shifts.7,8 Furthermore, the intriguing properties of CDs allow them to fulfill the crucial requirements of optical and photoelectronic devices, such as light-emitting diodes, photovoltaic devices and photodetectors.8–11 CDs, as a great candidate for bioimaging, exhibit broadband absorption and emission covering the deep ultraviolet, visible and near-infrared as well as efficient multi-photon up-conversion emission, which can be regulated by the surface functional species, size, shape and heteroatom doping.12–15 The high water solubility of CDs is a significant characteristic for materials in biological systems. CDs prepared by hydrothermal and solvothermal methods are functionalized with various types of surface groups, especially oxygen related carboxyl and hydroxyl groups, imparting excellent water solubility without further surface modification.16 The reactive groups at the rim of CDs greatly facilitate facile surface passivation and functionalization, which makes them suitable for drug delivery.
The optical and surface chemical properties of CDs make them promising candidates for theranostic applications, such as bioimaging,17 biosensing,18 and drug delivery, both in vitro and in vivo.19,20 In diagnostics, the fluorescence molecular bioimaging technique plays a vital role in tumour detection at early stages, which allows the noninvasive, highly sensitive and special observation of pathological and physiological events.21 In comparison to existing imaging materials, such as semiconductor quantum dots (QDs), organic dyes and fluorescent proteins, CDs have the advantages of low cytotoxicity, excellent biocompatibility, stable photoluminescence, facile functionalization and remarkable resistance to photobleaching.22,23 On the basis of the abovementioned benefits of CDs, various bioimaging applications in vivo and in vitro have been recently developed.24–30 In addition, driven by the high sensitivity, low-cost and utility, CDs have been extensively applied in the development of next-generation optical biosensors for not only elemental and biomarker analysis but also at the single cell level through monitoring the change of the fluorescence signals, termed as fluorescence enhancement (turn-on) or fluorescence quenching (off-state).31–33 Surface and/or edge modification of CDs is one of the applicable strategies for achieving targeted ability in photodynamic therapy (PDT), photothermal therapy (PTT) and chemotherapy.20,34–36 Effective and low-cost surface modification would be fundamental to future clinical translation of the current targeting technology.36–39
There have been already some reviews focusing on different aspects of CDs, such as their synthesis, surface functionalization, PL properties and biological applications.21,22,40,41 In this review, we will provide an update on the recent developments in the synthesis, optical properties, and biological applications of CDs. Following that, bioimaging of normal and cancer stem cells and tumour cells, two-photon (TP) fluorescence imaging and in vivo imaging, biosensing, and cancer therapy including PTT, PDT and chemotherapy (Fig. 1) will be discussed in detail. By comprehensively understanding the structure–function relationships of CDs, the present state and development prospects of CDs in biomedical applications are proposed.
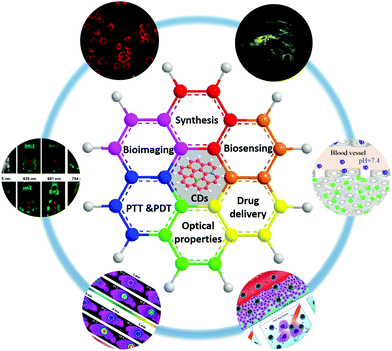 |
| Fig. 1 Schematic illustration of the topic of this review, showing the recent trends in applications of CDs in biomedicine, including bioimaging, biosensing, and cancer therapy. Reprinted with permission from ref. 20, 53, 54, 74, 82 and 143. Copyright 2017 American Chemical Society. Copyright 2012 and 2018 John Wiley and Sons. Copyright 2012 and 2017 Royal Society of Chemistry. Copyright 2016 Springer. | |
2. Preparation of CDs
In 2004, fluorescent carbon nanoparticles were first isolated by Scrivens et al. through the purification of single-walled carbon nanotubes.5 Since then, various synthetic methods have been developed for the preparation of CDs with different size and surface functional groups. Typically, CDs can be synthesized by either top-down or bottom-up approaches. They are based on cutting the graphite matrix into nanosized particles and the chemical fusion of small aromatic molecules, respectively. For the applications of CDs in optoelectronic devices, the bottom-up approach is commonly regarded as a suitable method for synthesizing bandgap fluorescent CDs.42,43 As for biological applications, both methods are widely used for the preparation of CDs. Particularly, the top-down method can facilely deliver good water-soluble CDs for biological applications. The top-down method involves oxidative cleavage,44 reductive cutting,45 physical grinding,46 laser ablation,6,47 electrochemical oxidation48 and hydrothermal cutting,49 making it possible to obtain CDs from a wide range of carbon materials, such as graphite,50 graphene oxide,51 and carbon nanotubes.52 Our laboratory, for the first time, prepared water-soluble, uniform sized GQDs that can directly and easily penetrate into stem cells without affecting their viability, proliferation or differentiation capacity by electrochemical exfoliation of graphite followed by hydrazine reduction at room temperature.53 Lately, a novel imaging probe, pH-responsive fluorescent graphene quantum dots (pRF-GQDs), was prepared by electrolysis of graphite rods in sodium p-toluenesulfonate acetonitrile solution. The resulting pRF-GQDs with minimal toxicity exhibited a sharp fluorescence transition between green and blue at pH 6.8, a pH matching the acidic extracellular microenvironment in solid tumours. We found that this unique fluorescence switching property allows distinguishing tumours from normal tissues.54 The top-down methods have the advantages of abundant starting materials and simple operation. Moreover, the GQDs prepared via top-down methods usually contain functional groups at the edges of the GQDs, thus facilitating the subsequent modification of the GQDs.55 Nevertheless, the bottom-up methods offer the opportunity to control CDs with well-defined size, shape, and stable properties through the selection of the precursors and modulation of the synthetic conditions. Previous work reported that CDs can be prepared by step-wise solution chemistry of organic molecules,56 polymerization,57 fullerene cage opening and carbonizing some special organic precursors.58 Beyond that, renewable biomass carbon sources were developed as a sort of raw materials for the preparation of CDs.59,60 Our laboratory reported GQDs synthesized by pyrolysis of citric acid (CA) at 200 °C for 20 min and subsequent functionalization with carboxyl groups via conjugation of acetic acid moieties. Such GQDs possessed exceptionally high loading capacity for IR780 (an effective theranostic agent) via π–π stacking interactions, while the hydrophilic carboxyl groups around the edge of the GQDs could covalently conjugate folic acid (FA) to enhance the tumour-targeting ability and avoid photodamage to surrounding normal tissues during PTT (Fig. 2a).20 The transmission electron micrograph (TEM) and high-resolution TEM (HRTEM) image indicated that most of the GQDs exhibited uniform atomic arrangements with a high degree of crystallinity. The carbon hexagon structures were arranged orderly and the well-crystallized carbon areas almost occupied the whole dot, demonstrating that GQDs may consist of a large and intact sp2-domain (Fig. 2b). Wu et al. synthesized GQDs with NIR fluorescence emission in the range 800–850 nm by the facile pyrolysis of L-glutamic acid.60 The as-prepared GQDs possessed strong excitation-dependent photoluminescence with a high quantum yield (QY: 54.5%) in solution, which were developed for biosensing and in vitro and in vivo bioimaging.
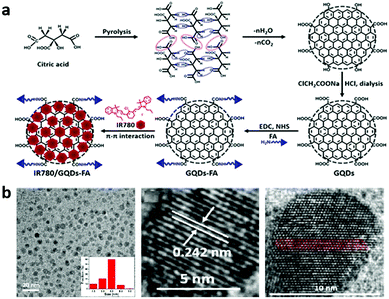 |
| Fig. 2 (a) Schematic illustrating the preparation of IR780/GQD-FA. Reprinted with permission from ref. 20. Copyright 2017 American Chemical Society. (b) TEM image and HRTEM image with the measured lattice spacing and the sp2 domain of the GQDs. Reprinted with permission from ref. 20. Copyright 2017 American Chemical Society. | |
In order to broaden the applications of CDs, further chemical modifications are required. Many applications of CDs depend on effective strategies to conjugate specific materials onto the surface of CDs. In principle, CDs can be chemically stable and biocompatible after the attachment of biomolecules and drugs.
Polyethylene glycol (PEG) and polyethylenimine (PEI) are two of the most commonly used reagents for surface passivation of CDs due to their great biocompatibility and resistance to nonspecific binding, which always show stronger PL emission and offer a vital intermediate for further modification.61 Recently, Shen et al. reported the preparation of PEG-modified GQDs by the connection of carboxyl groups of GQDs and hydroxyl groups of PEG via a facile one-pot hydrothermal approach.62 It should be noted that the as-prepared GQDs exhibited strong blue PL emission under an 808 nm laser, which functioned as a new type of upconversion fluorescence material for applications in bioscience. As reported by Wang et al., PEI-functionalized GQDs (PEI-GQDs) were synthesized from used coffee grounds as a raw material.63 The hydroxyl groups on the surface of the GQDs were replaced by PEI through a low-temperature hydrothermal route. The as-obtained PEI-GQDs revealed an efficient fluorescence property and low cytotoxicity in applications of bioimaging and sensing of heavy metals. Moreover, the attachment of multiple ligands (such as antibodies, peptides, proteins, and nucleic acids) to the surface of CDs can be designed for site-specific delivery, and fluorescence targeting, tracking, and imaging both in vitro and in vivo. For instance, Lee and coworkers presented a microwave irradiation technique for the synthesis of thiol-terminated CDs from glycerol (SH-g CDs), applied for conjugation with maleimide-attached targeting molecules, which can specially bind to glioma and cervical cancer cells for targeting cancers. The SH-g CD labeled aptamer will provide a useful targeting imaging probe for various cancers and diseases.64 Folate receptor (FR) can be used as a target for monitoring overexpressed molecules in cancer cells. Among targeting ligands, FA possesses high affinity toward FR.65 The targeting of cancer cells through detecting FR has attracted great attention. More recently, our laboratory reported the synthesis of FA functionalized GQDs, covalently conjugating FA by a 1-ethyl-3-(3-dimethylaminopropyl)carbodiimide/N-hydroxy-succinimide condensation reaction to enhance the tumour targeting ability and photothermal conversion efficiency.20 The results indicated that the conjugates offered new perspectives for imaging early diagnosis and monitoring of cancers.
3. Optical properties
Typically, CDs show characteristic optical absorption in the UV-region (230 to 320 nm) with a tail extending into the visible range due to the light absorption by the π-conjugated electrons in the sp2 atomic framework and the functional groups connected at the edge. The broad peak at ∼230 nm is ascribed to the π–π* transition of aromatic C
C bonds, whereas the shoulder at 300 nm is attributed to the n–π* transition of C
O bonds or other connected groups.40,66 The absorption characteristics of CDs are mainly influenced by the size of π-domains, shape, composition and surface functional groups.
Multicolored luminescent CDs have been synthesized with different approaches, ranging from deep ultraviolet to red or even NIR absorption and emission.67,68 As the particle size increased, CDs demonstrated red-shifted absorption bands from blue to red fluorescence, no matter how broad or narrow the absorption.42,69 Our laboratory reported the first demonstration of bright multicolor bandgap fluorescent CQDs (MCBF-CQDs) from blue to red with a QY up to 75% for blue fluorescence.42 These MCBF-CQDs exhibited strong excitonic absorption bands in the UV-vis absorption spectra (Fig. 3a) centered at about 350, 390, 415, 480, and 500 nm for blue (B-), green (G-), yellow (Y-), orange (O-), and red BF-CQDs (R-BF-CQDs), respectively. As shown in Fig. 3b, the fluorescence peaks of the MCBF-CQDs were centered at about 430 (B-), 513 (G-), 535 (Y-), 565 (O-), and 604 nm (R-BF-CQDs), respectively. The red shifted fluorescence colors from blue to red are consistent with the red-shifted excitonic absorption bands, indicating the band-edge nature of the optical transitions in the MCBF-CQDs. TEM characterization revealed that all of the five BF-CQDs demonstrated uniform and narrowly distributed nanoparticles with average sizes of about 1.95 (B-), 2.41 (G-), 3.78 (Y-), 4.90 (O-), and 6.68 nm (R-BF-CQDs). Recently, our laboratory reported high color-purity, narrow excitonic absorption and emission peak and multicolored (from blue to red) emission triangular CQDs (T-CQDs) with a QY up to 54–72%.69Fig. 3c presents the absorption spectra of the narrow bandwidth emission T-CQDs (NBE-T-CQDs) with strong and narrow excitonic absorption peaks centered at 460 (B-), 498 (G-), 521 (Y-), and 582 nm (R-NBE-T-CQDs), which were quite different from those of previously reported CQDs with ultrabroad absorption bands.7–18,23,26 The fluorescence spectra of the NBE-T-CQDs (Fig. 3d) also unveiled sharp excitonic emission peaks centered at 472 (B-), 507 (G-), 538 (Y-), and 598 nm (R-NBE-T-CQDs) with extremely narrow full width at half maximum (FWHM) values of only 30, 29, 30, and 30 nm, respectively. Bright multicolored emission of blue, green, yellow, and red was observed from each NBET-CQD solution with gradually increasing sizes from 1.9 nm to 2.4, 3.0, and 3.9 nm, respectively, which can be explained by the quantum confinement effect.7,16–18 Apart from the influence of the particle size, surface engineering, such as changing the surface state or functional groups, may also induce an absorption and emission wavelength red shift. In 2018, Qu et al. reported the red-shifted emission of CDs with increasing surface oxidation.70 The major absorption band of the CDs in H2O occurs at a wavelength of 540 nm with a long tail extending into the NIR region (Fig. 3e, yellow line). In contrast, the CDs in dimethyl sulfoxide (DMSO) exhibit a redshifted main absorption band at 619 nm, and an additional NIR absorption peak at 715 nm (Fig. 3e, red line). Under excitation at 561 nm, the emission of the CDs in H2O occurred in the red region (λmax = 624 nm) with a PL QY of 6% and there was enhanced red fluorescence with a PL QY of 26% (λem = 640 nm) in DMSO (Fig. 3f). Similarly, under excitation at 732 nm (Fig. 3g), owing to the NIR absorption band, these CDs also exhibited efficient NIR emission at 760 nm with a PL QY of 10% in DMSO but very weak NIR emission at about 760 nm in H2O. The as-synthesized CDs demonstrated NIR absorption and enhanced NIR fluorescence of CDs through surface engineering. Recently, our laboratory reported efficient red emission electron-donating group passivated CQDs at 637, 642, and 645 nm, respectively.71 The preparation of full-color light-emitting CDs was reported, which exhibited excitation-independent behaviors.13 Their comparative studies revealed the relationship between the degree of surface oxidation of the CDs and their PL red shifts, excluding quantum size effects.
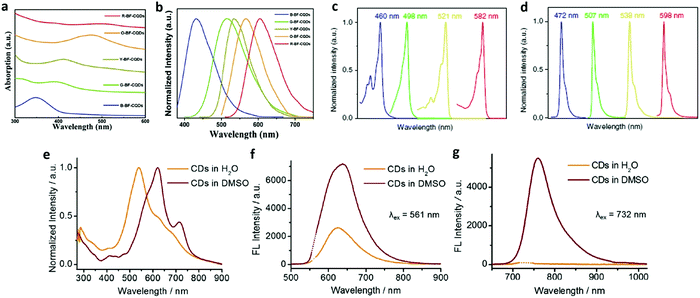 |
| Fig. 3 (a) UV-vis absorption and (b) normalized fluorescence of B-BF-CQDs, G-BF-CQDs, Y-BF-CQDs, O-BF-CQDs, and R-BF-CQDs. Reprinted with permission from ref. 42. Copyright 2016 John Wiley and Sons. The normalized UV-vis absorption (c) and PL (d) spectra of B-, G-, Y-, and R-NBE-T-CQDs, respectively. Reprinted with permission from ref. 69. Copyright 2018 Springer. (e) Absorption spectra of CDs in H2O and DMSO. Fluorescence spectra of CDs in H2O (f) and DMSO (g) under excitation with a 561 and 732 nm laser. Reprinted with permission from ref. 70. Copyright 2018 John Wiley and Sons. | |
For biological applications, the following optical properties are quite important. CDs with long-wavelength excitation/absorption and emission can be considered a good candidate for not only bioimaging but cancer therapy as well. Compared with a higher-energy photon, a lower-energy photon with a longer wavelength has been shown to penetrate deeper into the sample. Therefore, red and infrared radiation in the tissue transparency window can penetrate more deeply through tissue and minimize photodamage in comparison with short wavelength excitation light.72 And TP excitation penetrates more deeply into the tissue than single-photon excitation.15,73 Far-red and NIR emissive CDs have made great advances due to the low interference from autofluorescence with the tissues, minimum tissue absorption, and deep tissue penetration in comparison with fluorescence imaging in the visible light region.74,75 Moreover, bandgap fluorescent CDs, whose maximum excitation wavelength agrees well with the corresponding excitonic absorption peak, have make a great contribution to high QY, which is convenient for observation.
4. Biomedical applications of CDs
Assessing the biocompatibility of CDs before considering their biological application is important for any further advancement, which includes in vitro and in vivo tests. The in vitro toxicity is also known as the cytotoxicity while in vivo tests choose the whole organism as a research object. Until now, researchers have evaluated the in vitro cytotoxicity of various CDs. Typically, in vitro cytotoxicity experiments involve cellular viability experiments via certain assays such as MTT, CCK8, WST-1, etc.Table 1 presents a summary of in vitro cytotoxicity studies of CDs. Generally, CDs are acknowledged to be a nanomaterial with low cytotoxicity. The in vivo toxicity of CDs is usually observed after injection of CDs. To date, there are few studies about the in vivo toxicity of CDs with different injection doses. In 2018, Wang et al. investigated the biosafety of HBCDs in mice.76 They studied in vivo imaging of tumors and major organs at different time points post-i.v. injection of HBCDs. Compared with the control group without any treatment, no evident histological alterations were observed. Their results illustrated the low side effects and excellent biocompatibility of HBCDs in vivo. The body weights of mice were recorded during different treatments with HBCDs and no sudden change was found, indicating the low biotoxicity of HBCDs for in vivo treatment. Recently, some researchers studied the distribution of CDs in mice after injection. In 2019, Singh et al. investigated the in vivo toxicity of nitrogen doped CQDs (NCQDs).77In vivo toxic effects were evaluated for 30 days in Swiss albino mice at two different concentrations (5.0 mg per kg body weight (BW) and 10.0 mg per kg BW) of NCQDs. Their results of haematological, serum biochemical, antioxidant and histopathological parameters demonstrated that the NCQDs presented excellent biocompatibility.77
Table 1 Summary of in vitro cytotoxicity studies of CDs
CD type |
Cell model |
Assays |
Incubation time [h] |
Toxicity |
Ref. |
GQDs |
HeLa |
MTT |
24 |
90% viability at 1000 μg mL−1 |
87
|
S-GQDs |
HeLa |
MTT |
72 |
>95% viability at 1000 μg mL−1 |
88
|
N-GQDs |
HeLa |
CCK-8 |
24 |
96% viability at 200 μg mL−1 |
89
|
BN-GQDs |
HeLa |
CCK-8 |
24 |
90% viability at 400 μg mL−1 |
90
|
GQDs |
HeLa |
WST-1 |
24 |
95% viability at 200 μg mL−1 |
91
|
A549 |
85% viability at 640 μg mL−1 |
g-CNQDs |
U251 cells |
MTT |
24 |
92% viability at 300 μg mL−1 |
92
|
pRF-GQDs |
HeLa, MCF-7 |
CCK-8 |
24 |
>90% viability at 100 μg mL−1 |
54
|
IR780/GQD-FA |
HeLa |
CCK-8 |
12 |
>90% viability at 30 μg mL−1 |
20
|
(GQD/DBM)3EuPhen/GQD |
MCF-7 |
MTT |
24 |
>90% viability at 100 μg mL−1 |
82
|
RF-GQDs |
HeLa |
MTT |
24 |
>85% viability at 1000 μg mL−1 |
93
|
GQDs |
NSCs, CPCs |
MTT |
72 |
>80% viability at 100 μg mL−1 |
53
|
CD-Asp |
C6 glioma |
MTT |
48 |
>90% viability at 200 μg mL−1 |
94
|
N-GQDs |
HeLa |
MTS |
24 |
83% viability at 1000 μg mL−1 |
95
|
N-GQDs |
HeLa |
MTT |
24 |
>85% viability at 100 μg mL−1 |
96
|
FA-GdN@CQD-MWCNT |
MCF-7 |
MTT |
24 |
>90% viability at 600 μg mL−1 |
97
|
HFn/CD |
MCF-7 |
MTT |
48 |
>90% viability at 200 μg mL−1 |
98
|
Bioimaging techniques play a critical role in both fundamental research and clinical settings, allowing detailed observation of biological processes,78–80 which considerably facilitates the intuitive study of cell morphology and cell physiological processes. In cancer diagnostics, bioimaging is especially useful for the early detection of tumours and identification of metastasis as well as the resurgence of cancer. As a new class of bioimaging agents, CDs offer substantial advantages over inorganic semiconductor QDs and organic dyes in in vitro imaging owing to the unique combination of several key merits.81,82 Therefore, CDs have been regarded as a sensitive biological probe for both in vivo and in vitro imaging.81,83,84
In vitro imaging
Given the significance of cancer research, most of the CD-based studies focus on cancer cell imaging, which was first reported in 2011.85,86 The work unveiled that GQDs with stable fluorescence emission hold promising potential in bioimaging. Since then, attempts have been made for cancer cell imaging with various CDs, such as of MCF-7 cells,99,100 T47D cells,101 and HeLa cells.93,102 CDs with TP emission are urgently needed in cancer cell imaging, owing to the avoidance of harmful UV or blue excitation and the reduction of photodamage in biotissues.15 A facile approach was reported for the preparation of high-efficiency TP emission CDs with unique features of pure red emission (λmax ≈ 640 nm), high QY (22.9%), low cytotoxicity, high photothermal conversion efficiency (43.9% under irradiation with a 671 nm laser) and TP excited fluorescence.103 These red emissive CDs (R-CDs) were verified to be capable of TP fluorescence bioimaging in living cells (Fig. 4). As seen in TP mode, red emission from MCF-7 and HeLa cells can be observed under irradiation with an 850 nm fs pulse laser, clearly indicating the abilities of the R-CDs as TP bioimaging agents. Quantification of the fluorescence intensity profiles unveiled the stronger emission in the nucleoli regions, indicating that the R-CDs were capable of nucleolus staining. Because of the nucleolus being an RNA-rich region, the observed nucleolus staining implies that the R-CDs have higher affinity to RNA than DNA and other substances in the cytoplasm. Besides, the TP absorption cross-section is an important evaluation parameter for evaluating the luminescence efficiency. Nitrogen-doped GQDs (N-GQDs) have been used as efficient TP fluorescent probes for HeLa cell imaging.104 The HeLa cells became illuminated when imaged under irradiation at 800 nm. The TP absorption cross-section of the N-GQDs reached 48 000 Göppert–Mayer units, which far surpassed that of organic dyes and was comparable to that of high-performance semiconductor QDs.
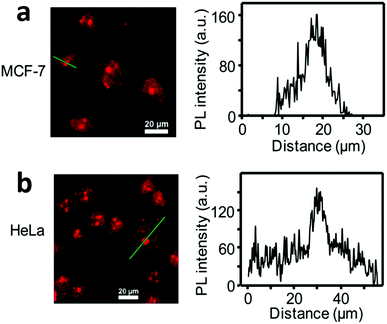 |
| Fig. 4 TP confocal fluorescence images of (a) living MCF-7 cells and (b) living HeLa cells, and corresponding quantitative figure along the green line (λex = 850 nm fs pulse laser, λem = 600–700 nm, scale bar = 20 μm). Reprinted with permission from ref. 103. Copyright 2016 American Chemical Society. | |
Stem cells are a class of multipotent cells with self-replication ability, which can give rise to tissue progenitor cells and in turn differentiate into tissue-forming cells.105–107 When stem cells or their progeny regenerate tissues and organs, there is a critical need to delineate the relative contribution to the regenerated tissues and organs from the delivered cells versus the host cells. Therefore, nontoxic and long-term stem cell imaging is urgently needed in the field of regenerative therapy for understanding their contribution to, migration to and fate in the regenerating tissues.102,108–112 We demonstrated the unique advantages of the GQDs mentioned above for highly efficient labeling of three different kinds of stem cells, including cardiac progenitor cells (CPCs), pancreas progenitor cells (PPCs) and neurospheres cells (NSCs), opening up great opportunities for their biomedical applications.
As shown in the confocal fluorescence images (Fig. 5a–f), the morphology of stem cells could be clearly discerned with the internalized GQDs.53 Even after being treated with GQDs, no significant changes were observed in the differentiation potential, metabolic activity, proliferation and viability of the cells. For illustrative purposes, we took two other fluorescent substances for comparison, including C60 nanoparticles and CdS QDs. Interestingly, the fluorescent C60 nanoparticles could not be internalized by stem cells, and could easily penetrate into cancer cells.113 In the case of CdS QDs, they were proved to be cytotoxic to stem cells. It was the first experimental observation of CDs as a fluorescent imaging agent in long-term stem cell imaging. The as-synthesized CDs are expected to have promising biomedical applications in comparison with previously reported carbon-based fluorescent nanomaterials, particularly in tracking differentiation of various cell lineages, apoptosis and proliferation, due to the scope for further straightforward functionalization. We further investigated the uptake mechanism and biocompatibility of such GQDs with human neural stem cells (hNSCs).107 It was confirmed that the GQDs were indeed internalized by the hNSCs via the endocytosis mechanism and were located in the cytoplasm. The GQDs did not affect the self-renewal and expression of the cell type-specific marker in hNSCs. Single cells dissociated from the GQD-labeled hNSCs formed large neurospheres after 12 days in normal culture medium and cells expressing the cell type-specific marker for hNSCs and nestin were identified.
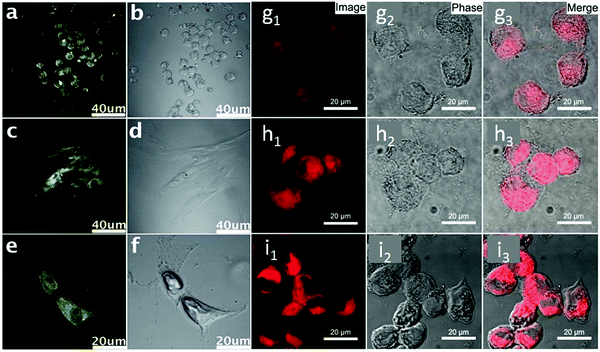 |
| Fig. 5 Confocal fluorescence microscopy images of stem cells of NSCs (a), PPCs (c) and CPCs (e) with fluorescent GQDs incorporated at an excitation wavelength of 405 nm and the corresponding images under bright field (b, d and f). Reprinted with permission from ref. 53. Copyright 2012 Royal Society of Chemistry. Confocal fluorescence microscopy images of pancreatic CSCs incubated with (g) 10 μM, (h) 25 μM and (i) 50 μM Fe3+ for 5 h, followed by further incubation with RBD-GQDs (25 mg L−1) for 0.5 h. Reprinted with permission from ref. 117. Copyright 2015 American Chemical Society. | |
As a subpopulation of stem-like cells, cancer stem cells (CSCs) exhibit the characteristics of both stem cells and cancer cells.114 They are capable of self-renewing inside a tumour and generating heterogeneous lineages of cancer cells, driving cancer progression, metastasis and drug resistance.115,116 CSCs could cause tumour relapse due to their strong self-renewal ability, and are considered a possible new target of cancer therapies. CSC labeling poses considerable challenges due to their particularity. Our laboratory successfully synthesized Rhodamine B derivative-functionalized GQDs (RBD-GQDs) and firstly explored their feasibility as CSC imaging agents.117 As shown in Fig. 5g–i, the RBD-GQDs exhibited strong orange-red intracellular fluorescence, indicating that the RBD-GQDs could penetrate the pancreatic CSC membrane.
In vivo imaging
Apart from in vitro cell imaging, CDs are increasingly used for in vivo cell imaging and have provided fruitful results. In 2009, a study about the feasibility of CDs as a fluorescent contrast agent in mice was first carried out (Fig. 6).118 Afterwards, a variety of CDs have been synthesized and used for in vivo imaging.74,119,120 Excitation with longer wavelengths is highly preferred for in vivo imaging due to deeper light penetration into the specimens. However, most previously reported CDs emitted only blue to green fluorescence. CDs for in vivo imaging emitting in the red or NIR region are still challenging. Tumour selective imaging in vivo regardless of the origin and location but not in normal tissues is also a major task. Our laboratory reported IR780/GQD-FA with an emission peak at 800 nm, which also was successfully applied for in vivo fluorescence imaging by intravenous injection.20Fig. 7 shows that the IR780/GQD-FA accumulated in the HeLa tumour area through folate-based active targeting and a significant fluorescence signal was observed in the tumour area in comparison with other tissues. The fluorescence intensity of IR780/GQD-FA accumulated in the tumour areas and peaked at 24 h post-injection. These are fluorescence imaging agents for cancer diagnosis in living systems. Mice were sacrificed 24 h post-injection, and the excised tumour exhibited strong fluorescence intensity, whereas the normal organs revealed very low signals, which was better than the in vivo behavior of many other previously reported CDs.
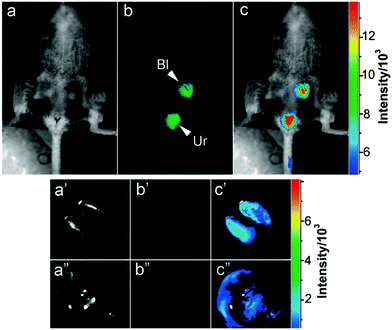 |
| Fig. 6 Intravenous injection of CDs: (a) bright field, (b) as-detected fluorescence (Bl, bladder; Ur, urine), and (c) color-coded images. The same order is used for the images of the dissected kidneys (a′–c′) and liver (a′′–c′′). Reprinted with permission from ref. 118. Copyright 2009 American Chemical Society. | |
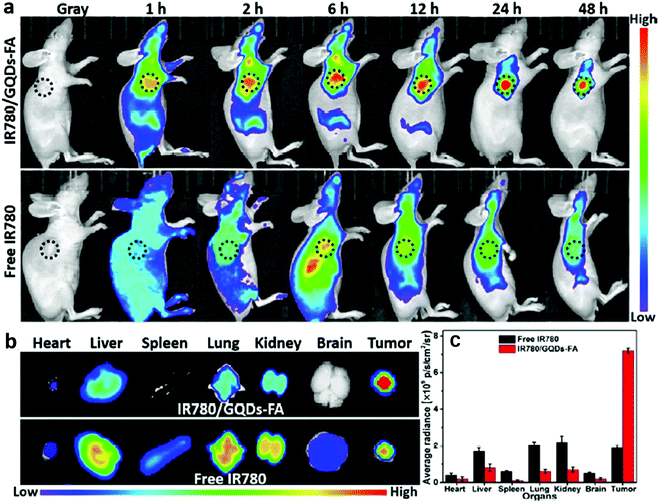 |
| Fig. 7 (a) NIR fluorescence imaging of tumour-bearing mice intravenously injected with free IR780 or IR780/GQD-FA during 48 h. The tumour is circled with a dotted line. (b) Ex vivo NIR fluorescence imaging of dissected organs at 24 h post-injection. (c) Mean fluorescence intensity of the excised organs from tumour-bearing mice treated with free IR780 or IR780/GQD-FA. Reprinted with permission from ref. 20. Copyright 2017 American Chemical Society. | |
Although great progress in fluorescence imaging technique of CDs has been made in the past several years, the spatial resolution is still far from satisfactory in in vivo imaging. It is necessary to develop multi-modal imaging probes through incorporation of other imaging modalities, such as photoacoustic (PA) imaging and magnetic resonance imaging.121 Recently, PA imaging has emerged as a novel method that allows imaging beyond the optical diffusion limit by integrating optical excitation with ultrasonic detection based on the PA effect, providing deeper tissue-imaging penetration and higher spatial resolution.122 Fluorescence and PA images of the tumor area at different time points were observed in comparison with other tissues. During the entire imaging process, the PA signals were relatively constant with prolonged circulation in blood vessels, indicating that CDs can be considered a candidate for PA imaging.
For in vivo imaging study, the radioactive imaging technique of CDs represents one of the most meaningful approaches due to the excellent imaging effect of radionuclides.123,124 In 2019, PEG and FA co-functionalized GQDs were successfully synthesized to simultaneously improve the biocompatibility and tumour-targeting ability of GQDs, and labeled GQD-PEG-FA with 131I (131I-GQD-PEG-FA) for biological behavior evaluation.125 The single photon emission computed tomography (SPECT) imaging of 131I-GQD-PEG-FA was investigated while the uptake of 131I-GQD-PEG-FA at tumour sites could be clearly examined via SPECT imaging (Fig. 8). The T/B and T/M values of the 131I-GQD-PEG-FA in this work were as high as 2.67 and 6.27 at 24 h after injection, suggesting promising clinical application.
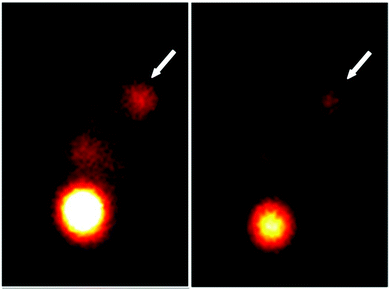 |
| Fig. 8 SPECT images of 131I-GQD-PEG-FA in tumour bearing mice (the arrows point at tumour sites). Reprinted with permission from ref. 125. Copyright 2019 Springer. | |
Biosensing
Biomolecular analysis plays an important role in clinical treatment security, food safety, environmental monitoring and disease diagnosis.126 Biosensors have been widely applied for analyzing small biological molecules, metal ions and proteins. In the past few years, inorganic semiconductor-based biosensors have been broadly investigated in numerous studies, exhibiting high fluorescence QY and photostability.127 However, they were found to cause health issues due to their heavy metal composition, which was unsuitable for in vivo applications.128,129 Recently, CD-based biosensors have received much attention. CDs have been demonstrated to exhibit excellent fluorescence performance with specific surface functional groups, which provide opportunities to connect with target analytes via various kinds of interactions such as electrostatic interactions, π–π conjugation or electron transfer, resulting in fluorescence turn-on or turn-off effects of CDs. Moreover, CDs deliver excellent electrical conductivity, good dispersibility, and a large specific surface area, which are advantageous for biological molecule loading on their surface, rendering them excellent fluorescent probes for the detection of analytes of interest including inorganic ions and small molecules in biological samples. For instance, those RBD-GQDs were also used as an Fe3+ turn-on fluorescence sensor, with a detection limit of 0.02 μM (Fig. 5g–i).117 The RBD-GQDs exhibited orange-red fluorescence, which was increased by the addition of Fe3+ ions due to the spirolactam ring of RBD being switched by Fe3+ ions. Further research demonstrated that the RBD-GQDs could penetrate the CSC membrane and be responsive to intracellular Fe3+ ions. In addition to the detection of metal ions, CDs can also be applied to the detection of other molecules. In 2015, a mitochondria-targetable nanoprobe based on triphenylphosphonium modified CDs with high sensitivity and selectivity was reported for peroxynitrite sensing in living cells.130 The fluorescence of the CDs was quenched in the presence of peroxynitrite due to the process of photoinduced electron transfer. Furthermore, this nanoprobe exhibits a universal and good mitochondria-targetable property towards many kinds of cancer cells. In 2016, Wang et al. reported a deliberately designed ON–OFF–ON fluorescent probe for the detection of glutathione (GSH) and temperature based on aggregation-induced fluorescence quenching and recovery, respectively.131 After adding GSH, the fluorescence of the CDs was then quenched strongly via the interaction between the surface groups and GSH, which led to the fluorescence switching to the OFF state. Increasing the temperature resulted in the destruction of this interaction and resulted in the fluorescence switching to the ON state. Furthermore, biosensing plays an indispensable role in the diagnosis and detection of cancer. Our laboratory proposed a new type of water-soluble, multicolor fluorescent GQDs which are responsive to all pH from 1 to 14, observable with the naked eye.108 The fluorescence of the resulting GQDs was also found to be responsive to temperature changes, demonstrating great potential as a dual probe of pH and temperature in complicated environments such as biological media (Fig. 9a–f). As an acid microenvironment exists in solid tumours regardless of their origin, CDs which respond to pH could be ideal for tumour detection. Our laboratory proposed pRF-GQDs for the early detection of tumours in 2017,54 which were prepared by electrolysis of graphite rods in sodium p-toluenesulfonate acetonitrile solution. As shown in Fig. 9g and h, the pRF-GQDs exhibited a sharp fluorescence transition between green and blue at pH 6.8, a pH matching the acidic extracellular microenvironment in solid tumours. Therefore, the pRF-GQDs had great potential to be used as a universal probe for fluorescence-guided cancer surgery and cancer diagnosis. Peng et al. made substantial contributions in the biosensing application of CDs. In 2018, they synthesized simultaneously hydrophilic and organophilic CDs by a facile one-pot solvothermal approach. The as-prepared CDs can be dissolved both in water and ethanol, exhibiting bright white and blue fluorescence under 365 nm UV illumination. Because the CDs exhibited high water solubility and good biocompatibility, they can be used for sensing Au3+ ions in aqueous solution and living cells, respectively.132 Other red emissive CDs were synthesized by using citric acid and neutral red as precursors, which demonstrated red fluorescence in both solution and the solid state. They were successfully applied for the detection of noble metal ions in PC12 cells and zebrafish.133
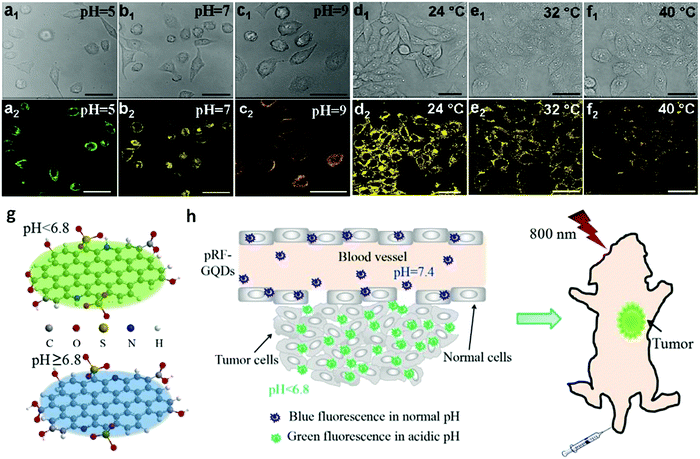 |
| Fig. 9 Cell imaging of HeLa cells at predetermined pH and temperature conditions (excitation at 405 nm). Bright field (a1, b1, and c1) and fluorescence field (a2, b2, and c2) of HeLa cells cultured at predetermined pH values. Bright field (d1, e1, and f1) and fluorescence field (d2, e2, and f2) imaging of HeLa cells at predetermined temperatures. Reprinted with permission from ref. 108. Copyright 2015 Royal Society of Chemistry. Schematic diagrams of pRF-GQDs at different pH values (g) and their application for tumour imaging (h). Reprinted with permission from ref. 54. Copyright 2017 Royal Society of Chemistry. | |
Tumor therapy
The development of safe and effective therapy methods plays a key role in cancer treatment, such as PDT, PTT and targeted chemotherapy. CDs can be chemically conjugated with targeting ligands, enabling them to effectively target tumours. The presence of the π-plane in the CDs allows chemotherapeutic drugs, photosensitizers and other therapeutic agents with aromatic rings to be tightly bound to the π-plane of the CDs, further increasing the targeting efficiency and drug loading capacity.
A PTT agent delivery platform was successfully designed on the basis of CDs. The IR780/GQD-FA mentioned above was also used for PTT in live mice under NIR irradiation (808 nm) with a high photothermal conversion efficiency of 87.9%.20 As presented in Fig. 10a, after being intravenously injected with saline or IR780/GQD-FA for 24 h, tumour-bearing nude mice were anesthetized and irradiated with an 808 nm laser for 5 min. The IR thermographic results revealed that the local tumour temperature reached 58.9 °C in the presence of IR780/GQD-FA upon 808 nm laser illumination for 5 min. As demonstrated in in vivo antitumour studies, IR780/GQD-FA in combination with laser irradiation exhibited obvious suppressive effects on tumour growth and the tumour almost disappears on day 15, while the volume of the tumours increased greatly in the saline group (Fig. 10b and c). As shown in Fig. 10d, there was no significant decrease in body weight of the mice treated with IR780/GQD-FA, indicating that IR780/GQD-FA is non-toxic at the given dose, which was also confirmed by hematoxylin and eosin (H&E) staining examination (Fig. 10e) and blood tests. In 2018, some heteroatom doped CDs were also used in PTT. Sulfur and nitrogen codoped NIR CDs were synthesized with intense absorption bands in the red to NIR region.134 The S, N-codoped CDs possessed a high photothermal conversion efficiency of 59% due to their unique NIR absorption feature. After intravenous injection, the S,N-codoped CDs were accumulated in tumour tissue via passive targeting. According to the IR thermographic characterization, the temperature in the tumour area rapidly reached 59–71 °C in the presence of the CDs under laser irradiation (5 min) (Fig. 11a). However, the maximum temperature of the tumour area in the control group (with intravenous phosphate buffered saline (PBS) injected) was only approximately 44 °C under the same irradiation conditions (Fig. 11b), demonstrating that the S,N-codoped CDs played a key role in heat generation. The antitumour studies showed that the CDs in combination with laser irradiation unveiled obvious suppressive effects on tumour growth and the tumour almost disappeared on day 10, while the volume of the tumours increased greatly in the PBS group (Fig. 11c). It should be mentioned that the mice in the PTT treatment group survived over 3 months without tumour reoccurrence, and the mice in the PBS group had an average life of 14–18 days (Fig. 11d). As shown in Fig. 11e, no significant signs of organ damage were observed in the PTT treatment group.
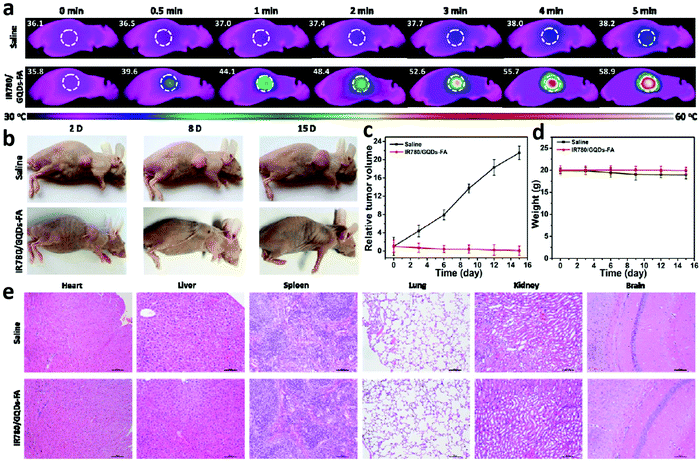 |
| Fig. 10 (a) Thermal images showing the temperature variations of tumours after intravenous injection of saline or IR780/GQD-FA with laser irradiation. (b) Representative images of tumour bearing mice after treatment. Tumour volume (c) and body weight (d) curves of tumour-bearing mice from the different treatment groups. (e) Histological evaluation of tissues from mice treated with saline and IR780/GQD-FA. Each organ was sliced for H&E staining. Reprinted with permission from ref. 20. Copyright 2017 American Chemical Society. | |
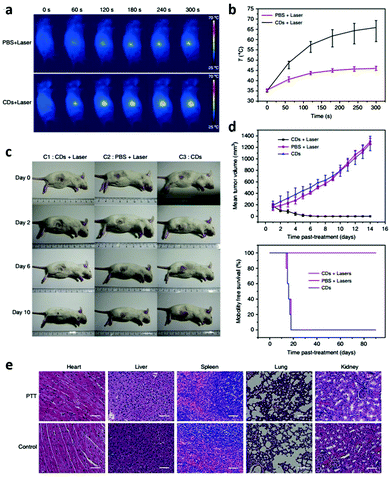 |
| Fig. 11 Photothermal therapy via intravenous injection based on CDs. (a) IR thermal images of mice with intravenous CDs injected at 10, 60, 120, 180, 240, and 300 s under irradiation at the tumour region with a 655 nm laser at 1 W cm−2. (b) Temperature at mouse tumour sites as a function of the irradiation duration. (c) Photographs that document H22 tumour development on several days in live mice under various treatment conditions. (d) Tumour growth curves of H22 tumours in mice and survival rates of the groups after therapy. (e) Hematoxylin and eosin (H&E)-stained slices of heart, liver, spleen, lung, and kidney tissues of mice after PTT and control treatments. Scale bar: 50 μm. Reprinted with permission from ref. 134. Copyright 2018 Springer Nature Publishing AG. | |
According to previous studies on the ability to efficiently generate reactive oxygen species (ROS), negligible drug resistance, low systemic toxicity, and flexible remote controllability and non-invasive procedures, PDT is considered to be a promising approach in cancer therapy.135,136 In recent research, tumour cells were killed by ROS produced by CDs in the presence of oxygen under irradiation.137,138 ROS production from CDs under irradiation is becoming a major concern in cancer therapy, which provides a unique opportunity to use CDs as PDT agents by enhancing their ability to kill tumour cells. For the first time, CDs were found to generate ROS under irradiation with blue light in vitro for application in PDT in 2011.137 However, the blue-emission CDs could hardly be used in clinical application due to their shallow tissue penetration. Accordingly, red-emission CDs were then introduced due to the non-invasive nature, minimum autofluorescence background of bio-samples and deep tissue penetration.139 Highly water-dispersible CDs (emission peak at 680 nm) were prepared using a hydrothermal method with polythiophene derivatives (PT2) as the carbon source.57 Through in vitro and in vivo studies, it was demonstrated that the CDs could be used as PDT agents with good biocompatibility and excellent ROS generation capability via a multistate sensitization process. It was first demonstrated that the CDs with a high ROS generating efficiency acted as a PDT agent for cancer treatment in vivo. Subsequently, other CDs with red emission were developed (emission peak at 640 nm) using polythiophene phenylpropionic acid as a precursor.140 More importantly, a high photothermal conversion efficiency of 38.5% under NIR light irradiation was also realized, which significantly broadened the applications of CDs in photothermal-synergistic therapy. Due to the oxygen dependence of PDT, hypoxia resulting from the rapid consumption of oxygen in the PDT process would reduce the therapeutic effect.141 Because the oxygen supply is reduced in the tumour microenvironment, hypoxia is commonly found in most solid tumours. Therefore, the inadequate oxygen supply in tumours prevents PDT from achieving its full therapeutic potential.142 In order to overcome these difficulties resulting from the hypoxia in the PDT process, magneto-fluorescent Mn-CDs were prepared by using manganese(II) phthalocyanine as a precursor.143 Significantly, the Mn-CDs can not only effectively produce ROS but highly catalyze the generation of oxygen as well (Fig. 12). Thanks to the catalysis of Mn-CDs, the tumour hypoxia was improved and enhanced PDT was then achieved. This work considerably broadened the scope of CDs in the biomedical field and provided a versatile strategy for PDT.
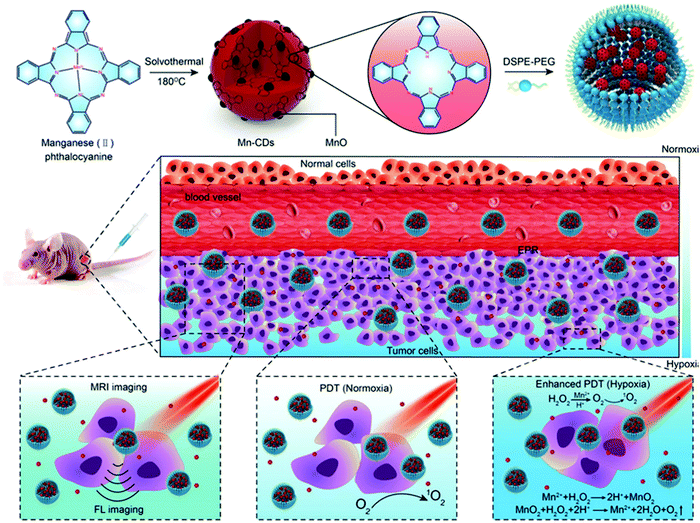 |
| Fig. 12 Schematic illustration of the Mn-CD assembly as an acidic H2O2-driven oxygenerator to enhance the anticancer efficiency of PDT in a solid tumour. Reprinted with permission from ref. 143. Copyright 2018 John Wiley and Sons. | |
Further significant therapeutic potential of CDs is their ability to serve as carriers of chemotherapeutic drugs to improve the delivery efficiency or offer benefits in the therapeutic effect.144 For instance, GQD-based drug delivery highlighted that attaching the tumour-targeting module biotin (vitamin K or vitamin B7) on the edge of GQDs specifically recognized the receptors overexpressed on cancer cells and led to the increased internalization of GQDs. This study explored a new biocompatible and cell-traceable drug delivery system, which was able to release DOX to cancer cells in a selective manner and minimize the anticancer drug systemic toxicity and undesirable side effects. Based on arginine–glycine–aspartic acid-modified GQDs loaded with DOX, a biocompatible and multifunctional drug delivery system for the targeted treatment of prostate cancer was developed.145 Surface modification of the GQD-based drug delivery system with RGD facilitated the internalization of GQDs and consequentially enhanced the availability and effect of the carried DOX.106
Tumour gene therapy describes the technique of delivering genetic materials to tumour cells in order to treat cancers. Similar to the requirements for drug delivery systems, effective carriers in gene therapy are capable of bypassing biological barriers to ensure delivery of the genetic materials into cells.146 While viral vectors are effective in gene delivery due to their natural ability to invade and deliver their genetic material, the barrier of immunogenicity induced by viral vectors has kept them far from safe for clinical use.147 Due to the traits of being an effective gene carrier, CDs have attracted great attention as non-viral vectors in gene therapy. A study in 2017 showed that CDs could be well applied in gene delivery.148 Non-toxic GQDs with two green and red emission colors were synthesized by Hummers’ method and the GQDs could be conjugated with the MPG-2H1 chimeric peptide and plasmid DNA (pDNA) by non-covalent interactions. The GQDs could allow efficient tracking and enhanced internalization of the plasmid harboring the firefly luciferase gene into HEK 293T cells and the results of confocal microscopy demonstrated that their construct can be considered as a nontoxic carrier with dual functions of gene delivery and nuclear targeting. This study suggests that CDs are a promising transfection vector that could lead to more in vitro and in vivo applications of CD-based gene therapy. Up to now, some CD-based non-viral vectors for delivery of genetic material have been investigated in in vivo and in vitro experiments. Based on their interesting properties, CDs have great potential as genetic material carriers in clinical studies.
5. Conclusions and perspectives
In this review, we summarize the recent research progress of CDs on the synthesis and optical properties with a critical survey which addressed in detail their advanced applications in biomedicine. The intriguing merits of CDs are highlighted in their applications for bioimaging including of stem cells and tumour cells, TP fluorescence imaging, red or NIR emission for in vivo imaging, biosensing, cancer therapy including photodynamic therapy, photothermal therapy and chemotherapy. There is no doubt that the development of CDs for biomedical applications has seen great progress within a short time. However, future research still faces challenges in terms of fundamental understanding and practical applications as will be stated below.
For bioimaging, due to their minimal photodamage, low interference from autofluorescence with biological tissues, minimum tissue absorption, and deep tissue penetration, NIR-II fluorescence properties of CDs (1000–1700 nm) are highly desired. Up to now, a number of studies have acquired efficient NIR-I emission CDs. In this regard, more in-depth research should be carried out to address this issue, which is expect to direct the rational design of CDs for bioimaging. In addition, radiotherapy has significantly increased patients’ overall survival and quality of life in the current clinic. Radioactive labelling of CDs is one of the most meaningful tasks which should be further explored in in vivo imaging. Moreover, radioactive labelled CDs can help us systematically investigate the pharmacokinetics, biodistribution, and toxicology of this type of nanomaterials.
For cancer therapy, recent efforts have been primarily focused on engineering imaging or therapeutic agents through conjugation of ligands that recognize “receptor” molecules expressed on cancer cells or in the tumour microenvironment. First, receptor molecules that are exclusively expressed on the surface of cancer cells are quite rare, as most of them are also present in normal tissues. As a result, the accumulation of cargo agents in normal tissues cannot be avoided. Second, cancer represents a group of highly heterogeneous diseases. Cancers of different tissue origin or intra-tumour location often have distinct genotypes and phenotypes. Consequently, they may not be targeted using a single ligand. Third, targeted imaging and drug delivery to tumours in the brain is further complicated by the blood–brain barrier (BBB), which prevents the penetration of most agents into the brain. Although there have been tremendous improvements in the clinical management of other tumours, the prognosis for most brain tumours remains dismal. Conventional intravenous chemotherapies, including those delivered via liposomes, are ineffective due to the limited entry across the BBB. Engineering drug delivery to the brain has been mainly attempted through traditional ligand-mediated targeted delivery, which has had limited success. The current approach to imaging intracranial tumours, which depends on the amount of contrast agent that penetrates through the leaky BBB, has been proved to be inadequate to detect infiltrative tumour cells in the region with the intact or less disruptive BBB. The development of high specificity and efficiency CDs for imaging and treatment of brain tumours remains a major challenge.
Despite these challenges, it is optimistic that the boom in carbon dots will advance their applications in bioimaging, biosensing and biotherapy. Through combinations of experimental and theoretical studies, more efforts should be made on developing biocompatible CDs and exploring their clinical applications.
Conflicts of interest
There are no conflicts to declare.
Acknowledgements
This work is supported by NSFC of China (21573019, 21872010). We also acknowledge the support from Key Laboratory of Radiopharmaceuticals and Key Laboratory of Theoretical and Computational Photochemistry, Ministry of Education.
Notes and references
- K. A. S. Fernando, S. Sahu, Y. Liu, W. K. Lewis, E. A. Guliants, A. Jafariyan, P. Wang, C. E. Bunker and Y. P. Sun, ACS Appl. Mater. Interfaces, 2015, 7, 8363–8376 CrossRef CAS PubMed.
- C. Q. Ding, A. W. Zhu and Y. Tian, Acc. Chem. Res., 2014, 47, 20–30 CrossRef CAS PubMed.
- H. J. Sun, L. Wu, W. L. Wei and X. G. Qu, Mater. Today, 2013, 16, 433–442 CrossRef CAS.
- H. T. Li, X. D. He, Z. H. Kang, H. Huang, Y. Liu, J. L. Liu, S. Y. Lian, C. H. A. Tsang, X. B. Yang and S. T. Lee, Angew. Chem., Int. Ed., 2010, 49, 4430–4434 CrossRef CAS PubMed.
- X. Xu, R. Ray, Y. L. Gu, H. J. Ploehn, L. Gearheart, K. Raker and W. A. Scrivens, J. Am. Chem. Soc., 2004, 126, 12736–12737 CrossRef CAS PubMed.
- Y. P. Sun, B. Zhou, Y. Lin, W. Wang, K. A. S. Fernando, P. Pathak, M. J. Meziani, B. A. Harruff, X. Wang, H. Wang, P. G. Luo, H. Yang, M. E. Kose, B. Chen, L. M. Veca and S. Y. Xie, J. Am. Chem. Soc., 2006, 128, 7756–7757 CrossRef CAS PubMed.
- R. Mohammadinejad, S. Karimi, S. Iravani and R. S. Varma, Green Chem., 2016, 18, 20–52 RSC.
- M. Baldrighi, M. Trusel, R. Tonini and S. Giordani, Front. Neurosci., 2016, 10, 250 Search PubMed.
- T. Meng, T. Yuan, X. H. Li, Y. C. Li, L. Z. Fan and S. Yang, Chem. Commun., 2019, 55, 6531–6534 RSC.
- Q. Guo, F. L. Yuan, B. Zhang, S. J. Zhou, J. Zhang, Y. M. Bai, L. Z. Fan, T. Hayat, A. Alsaedi and Z. A. Tan, Nanoscale, 2019, 11, 115–124 RSC.
- F. L. Yuan, Z. F. Xi, X. Y. Shi, Y. C. Li, X. H. Li, Z. N. Wang, L. Z. Fan and S. H. Yang, Adv. Opt. Mater., 2019, 7, 1801202 CrossRef.
- Y. B. Yan, J. Gong, J. Chen, Z. P. Zeng, W. Huang, K. Y. Pu, J. Y. Liu and P. Chen, Adv. Mater., 2019, 31, 1808283 CrossRef PubMed.
- H. Ding, S. B. Yu, J. S. Wei and H. M. Xiong, ACS Nano, 2016, 10, 484–491 CrossRef CAS PubMed.
- H. Ding, J. S. Wei, P. Zhang, Z. Y. Zhou, Q. Y. Gao and H. M. Xiong, Small, 2018, 14, 1800612 CrossRef PubMed.
- F. L. Yuan, Y. C. Li, X. H. Li, J. Zhu, L. Z. Fan, S. X. Zhou, Y. R. Zhang and J. B. Zhou, ACS Appl. Bio. Mater., 2018, 1, 853–858 CrossRef CAS.
- S. Y. Lim, W. Shen and Z. Q. Gao, Chem. Soc. Rev., 2015, 44, 362–381 RSC.
- A. L. Antaris, J. T. Robinson, O. K. Yaghi, G. Hong, S. Diao, R. Luong and H. Dai, ACS Nano, 2013, 7, 3644–3652 CrossRef CAS PubMed.
- S. J. Zhu, Q. N. Meng, L. Wang, J. H. Zhang, Y. B. Song, H. Jin, K. Zhang, H. C. Sun, H. Y. Wang and B. Yang, Angew. Chem., Int. Ed., 2013, 52, 3953–3957 CrossRef CAS PubMed.
- R. Wang, K. Q. Lu, Z. R. Tang and Y. J. Xu, J. Mater. Chem. A, 2017, 5, 3717–3734 RSC.
- S. H. Li, S. X. Zhou, Y. C. Li, X. H. Li, J. Zhu, L. Z. Fan and S. H. Yang, ACS Appl. Mater. Interfaces, 2017, 9, 22332–22341 CrossRef CAS PubMed.
- H. T. Lu, W. J. Li, H. F. Dong and M. L. Wei, Small, 2019, 0, 1902136 CrossRef PubMed.
- F. L. Yuan, S. H. Li, Z. T. Fan, X. Y. Meng, L. Z. Fan and S. H. Yang, Nano Today, 2016, 11, 565–586 CrossRef CAS.
- Y. Li, Y. Zhao, H. H. Cheng, Y. Hu, G. Q. Shi, L. M. Dai and L. T. Qu, J. Am. Chem. Soc., 2012, 134, 15–18 CrossRef CAS PubMed.
- L. Cao, X. Wang, M. J. Meziani, F. S. Lu, H. F. Wang, P. G. Luo, Y. Lin, B. A. Harruff, L. M. Veca, D. Murray, S. Y. Xie and Y. P. Sun, J. Am. Chem. Soc., 2007, 129, 11318–11319 CrossRef CAS PubMed.
- S. Pandit, P. Behera, J. Sahoo and M. De, ACS Appl. Bio. Mater., 2019, 2, 3393–3403 CrossRef CAS.
- T. Malina, K. Poláková, J. Skopalík, V. Milotová, K. Holá, M. Havrdová, K. B. Tománková, V. Čmiel, L. Šefc and R. Zbořil, Carbon, 2019, 152, 434–443 CrossRef CAS.
- S. Fasbender, L. Zimmermann, R. P. Cadeddu, M. Luysberg, B. Moll, C. Janiak, T. Heinzel and R. Haas, Sci. Rep., 2019, 9, 12028 CrossRef PubMed.
- A. B. Ganganboina and R. A. Doong, Sci. Rep., 2019, 9, 7214 CrossRef PubMed.
- D. Kim, J. M. Yoo, H. Hwang, J. Lee, S. H. Lee, S. P. Yun, M. J. Park, M. Lee, S. Choi, S. H. Kwon, S. Lee, S. H. Kwon, S. Kim, Y. J. Park, M. Kinoshita, Y.-H. Lee, S. Shin, S. R. Paik, S. J. Lee, S. Lee, B. H. Hong and H. S. Ko, Nat. Nanotechnol., 2018, 13, 812–818 CrossRef CAS PubMed.
- C. Wang, C. Y. Wu, X. J. Zhou, T. Han, X. Z. Xin, J. Y. Wu, J. Y. Zhang and S. W. Guo, Sci. Rep., 2013, 3, 2852 CrossRef PubMed.
- K. P. Carter, A. M. Young and A. E. Palmer, Chem. Rev., 2014, 114, 4564–4601 CrossRef CAS PubMed.
- D. Jariwala, V. K. Sangwan, L. J. Lauhon, T. J. Marks and M. C. Hersam, Chem. Soc. Rev., 2013, 42, 2824–2860 RSC.
- W. Wang, Y. C. Lu, H. Huang, A. J. Wang, J. R. Chen and J. J. Feng, Biosens. Bioelectron., 2015, 64, 517–522 CrossRef CAS PubMed.
- A. Razzazan, F. Atyabi, B. Kazemi and R. Dinarvand, Mater. Sci. Eng., C, 2016, 62, 614–625 CrossRef CAS PubMed.
- X. J. Gong, Q. Y. Zhang, Y. F. Gao, S. M. Shuang, M. M. F. Choi and C. Dong, ACS Appl. Mater. Interfaces, 2016, 8, 11288–11297 CrossRef CAS PubMed.
- X. Sui, C. Luo, C. Wang, F. W. Zhang, J. Y. Zhang and S. W. Guo, Nanomedicine, 2016, 12, 1997–2006 CrossRef CAS PubMed.
- T. Feng, X. Z. Ai, G. H. An, P. P. Yang and Y. L. Zhao, ACS Nano, 2016, 10, 4410–4420 CrossRef CAS PubMed.
- K. Yang, L. Z. Feng and Z. Liu, Adv. Drug Delivery Rev., 2016, 105, 228–241 CrossRef CAS PubMed.
- D. Q. Chen, C. A. Dougherty, K. Zhu and H. Hong, J. Controlled Release, 2015, 210, 230–245 CrossRef CAS PubMed.
- T. Yuan, T. Meng, P. He, Y. X. Shi, Y. C. Li, X. H. Li, L. Z. Fan and S. H. Yang, J. Mater. Chem. C, 2019, 7, 6820–6835 RSC.
- J. J. Du, N. Xu, J. L. Fan, W. Sun and X. J. Peng, Small, 2019, 15, 1805087 CrossRef PubMed.
- F. L. Yuan, Z. B. Wang, X. H. Li, Y. C. Li, Z. A. Tan, L. Z. Fan and S. H. Yang, Adv. Mater., 2017, 29, 1604436 CrossRef PubMed.
- F. Yuan, P. He, Z. F. Xi, X. H. Li, Y. C. Li, H. Z. Zhong, L. Z. Fan and S. H. Yang, Nano Res., 2019, 12, 1669–1674 CrossRef CAS.
- Z. M. Luo, G. Q. Qi, K. Y. Chen, M. Zou, L. H. Yuwen, X. W. Zhang, W. Huang and L. H. Wang, Adv. Funct. Mater., 2016, 26, 2739–2744 CrossRef CAS.
- S. H. Jin, D. H. Kim, G. H. Jun, S. H. Hong and S. Jeon, ACS Nano, 2013, 7, 1239–1245 CrossRef CAS PubMed.
- H. Yoon, Y. H. Chang, S. H. Song, E. S. Lee, S. H. Jin, C. Park, J. Lee, B. H. Kim, H. J. Kang, Y. H. Kim and S. Jeon, Adv. Mater., 2016, 28, 5255–5261 CrossRef CAS PubMed.
- H. S. Han, J. S. Lee, J. H. Ryu, K. M. Kim, J. L. Jones, J. Lim, S. Guillemet-Fritsch, H. C. Lee and S. Mhin, J. Phys. Chem. C, 2016, 120, 13667–13674 CrossRef CAS.
- Y. Li, Y. Hu, Y. Zhao, G. Q. Shi, L. Deng, Y. B. Hou and L. Qu, Adv. Mater., 2011, 23, 776–780 CrossRef CAS PubMed.
- D. Y. Pan, J. C. Zhang, Z. Li and M. H. Wu, Adv. Mater., 2010, 22, 734–738 CrossRef CAS PubMed.
- J. Joseph and A. A. Anappara, ChemPhysChem, 2017, 18, 292–298 CrossRef CAS PubMed.
- S. J. Zhuo, M. W. Shao and S. T. Lee, ACS Nano, 2012, 6, 1059–1064 CrossRef CAS PubMed.
- D. B. Shinde and V. K. Pillai, Chem. – Eur. J., 2012, 18, 12522–12528 CrossRef CAS PubMed.
- M. Zhang, L. L. Bai, W. H. Shang, W. J. Xie, H. Ma, Y. Y. Fu, D. C. Fang, H. Sun, L. Z. Fan, M. Han, C. M. Liu and S. H. Yang, J. Mater. Chem., 2012, 22, 7461–7467 RSC.
- Z. T. Fan, S. X. Zhou, C. Garcia, L. Z. Fan and J. B. Zhou, Nanoscale, 2017, 9, 4928–4933 RSC.
- L. L. Li, G. H. Wu, G. H. Yang, J. Peng, J. W. Zhao and J. J. Zhu, Nanoscale, 2013, 5, 4015–4039 RSC.
- J. Lu, P. S. E. Yeo, C. K. Gan, P. Wu and K. P. Loh, Nat. Nanotechnol., 2011, 6, 247 CrossRef CAS PubMed.
- J. C. Ge, M. H. Lan, B. J. Zhou, W. M. Liu, L. Guo, H. Wang, Q. Y. Jia, G. L. Niu, X. Huang, H. Y. Zhou, X. M. Meng, P. F. Wang, C. S. Lee, W. J. Zhang and X. D. Han, Nat. Commun., 2014, 5, 4596 CrossRef CAS PubMed.
- R. L. Liu, D. Q. Wu, X. L. Feng and K. Müllen, J. Am. Chem. Soc., 2011, 133, 15221–15223 CrossRef CAS PubMed.
- W. X. Meng, X. Bai, B. Y. Wang, Z. Y. Liu, S. Y. Lu and B. Yang, Energy Environ. Mater., 2019, 1–21 CAS.
- V. Sharma, P. Tiwari and S. M. Mobin, J. Mater. Chem. B, 2017, 5, 8904–8924 RSC.
- E. L. Bentzen, I. D. Tomlinson, J. Mason, P. Gresch, M. R. Warnement, D. Wright, E. Sanders-Bush, R. Blakely and S. J. Rosenthal, Bioconjugate Chem., 2005, 16, 1488–1494 CrossRef CAS PubMed.
- J. H. Shen, Y. H. Zhu, X. L. Yang, J. Zong, J. M. Zhang and C. Z. Li, New J. Chem., 2012, 36, 97–101 RSC.
- L. Wang, W. Li, B. Wu, Z. Li, S. L. Wang, Y. Liu, D. Y. Pan and M. H. Wu, Chem. Eng. J., 2016, 300, 75–82 CrossRef CAS.
- C. H. Lee, R. Rajendran, M.-S. Jeong, H. Y. Ko, J. Y. Joo, S. Cho, Y. W. Chang and S. Kim, Chem. Commun., 2013, 49, 6543–6545 RSC.
- X. W. Zhao, J. L. Zhang, L. H. Shi, M. Xian, C. Dong and S. M. Shuang, RSC Adv., 2017, 7, 42159–42167 RSC.
- Z. Wang, F. Yuan, X. Li, Y. Li, H. Zhong, L. Fan and S. Yang, Adv. Mater., 2017, 29, 1702910 CrossRef.
- J. C. Ge, M. H. Lan, B. J. Zhou, W. M. Liu, L. Guo, H. Wang, Q. Y. Jia, G. L. Niu, X. Huang, H. Y. Zhou, X. M. Meng, P. F. Wang, C. S. Lee, W. J. Zhang and X. D. Han, Nat. Commun., 2014, 5, 4596 CrossRef CAS PubMed.
- L. Tang, R. B. Ji, X. M. Li, G. X. Bai, C. P. Liu, J. H. Hao, J. Y. Lin, H. X. Jiang, K. S. Teng, Z. B. Yang and S. P. Lau, ACS Nano, 2014, 8, 6312–6320 CrossRef CAS.
- F. L. Yuan, T. Yuan, L. Z. Sui, Z. B. Wang, Z. F. Xi, Y. C. Li, X. H. Li, L. Z. Fan, Z. A. Tan, A. Chen, M. X. Jin and S. H. Yang, Nat. Commun., 2018, 9, 2249 CrossRef PubMed.
- D. Li, P. T. Jing, L. H. Sun, Y. An, X. Y. Shan, X. H. Lu, D. Zhou, D. Han, D. Z. Shen, Y. C. Zhai, S. N. Qu, R. Zbořil and A. L. Rogach, Adv. Mater., 2018, 30, 1705913 CrossRef PubMed.
- H. R. Jia, Z. B. Wang, T. Yuan, F. L. Yuan, X. H. Li, Y. C. Li, Z. A. Tan, L. Z. Fan and S. H. Yang, Adv. Sci., 2019, 6, 1900397 CrossRef PubMed.
- X. X. Shi, H. M. Meng, Y. Q. Sun, L. B. Qu, Y. H. Lin, Z. H. Li and D. Du, Small, 2019, 0, 1901507 CrossRef CAS PubMed.
- H. M. Kim and B. R. Cho, Chem. Rev., 2015, 115, 5014–5055 CrossRef CAS PubMed.
- H. Q. Tao, K. Yang, Z. Ma, J. M. Wan, Y. J. Zhang, Z. H. Kang and Z. Liu, Small, 2012, 8, 281–290 CrossRef CAS PubMed.
- L. P. Li, R. P. Zhang, C. X. Lu, J. H. Sun, L. J. Wang, B. T. Qu, T. T. Li, Y. D. Liu and S. J. Li, J. Mater. Chem. B, 2017, 5, 7328–7334 RSC.
- Q. Y. Jia, X. L. Zheng, J. C. Ge, W. M. Liu, H. H. Ren, S. Q. Chen, Y. M. Wen, H. Y. Zhang, J. S. Wu and P. F. Wang, J. Colloid Interface Sci., 2018, 526, 302–311 CrossRef CAS PubMed.
- V. Singh, S. Kashyap, U. Yadav, A. Srivastava, A. V. Singh, R. K. Singh, S. K. Singh and P. S. Saxena, Toxicol. Res., 2019, 8, 395–406 CrossRef CAS PubMed.
- N. Abdullah, Al, J. E. Lee, I. In, H. Lee, K. D. Lee, J. H. Jeong and S. Y. Park, Mol. Pharmaceutics, 2013, 10, 3736–3744 CrossRef PubMed.
- M. Nurunnabi, Z. Khatun, K. M. Huh, S. Y. Park, D. Y. Lee, K. J. Cho and Y. K. Lee, ACS Nano, 2013, 7, 6858–6867 CrossRef CAS PubMed.
- S. Fasbender, S. Allani, C. Wimmenauer, R. P. Cadeddu, K. Raba, J. C. Fischer, B. Bulat, M. Luysberg, C. A. M. Seidel, T. Heinzel and R. Haas, RSC Adv., 2017, 7, 12208–12216 RSC.
- X. T. Zheng, A. Ananthanarayanan, K. Q. Luo and P. Chen, Small, 2015, 11, 1620–1636 CrossRef CAS PubMed.
- Y. T. Liu, S. X. Zhou, L. Z. Fan and H. Fan, Microchim. Acta, 2016, 183, 2605–2613 CrossRef CAS.
- Z. T. Fan, S. H. Li, F. L. Yuan and L. Z. Fan, RSC Adv., 2015, 5, 19773–19789 RSC.
- Y. B. Song, S. J. Zhu and B. Yang, RSC Adv., 2014, 4, 27184–27200 RSC.
- S. J. Zhu, J. H. Zhang, C. Y. Qiao, S. J. Tang, Y. F. Li, W. J. Yuan, B. Li, L. Tian, F. Liu, R. Hu, H. N. Gao, H. T. Wei, H. Zhang, H. C. Sun and B. Yang, Chem. Commun., 2011, 47, 6858–6860 RSC.
- S. J. Zhu, J. H. Zhang, X. Liu, B. Li, X. F. Wang, S. J. Tang, Q. N. Meng, Y. F. Li, C. Shi, R. Hu and B. Yang, RSC Adv., 2012, 2, 2717–2720 RSC.
- R. V. Nair, R. T. Thomas, V. Sankar, H. Muhammad, M. Dong and S. Pillai, ACS Omega, 2017, 2, 8051–8061 CrossRef CAS PubMed.
- K. X. Jin, H. Gao, L. H. Lai, Y. Q. Pang, S. Y. Zheng, Y. N. Niu and X. L. Li, J. Lumin., 2018, 197, 147–152 CrossRef CAS.
- Y. Fu, G. Y. Gao and J. F. Zhi, J. Mater. Chem. B, 2019, 7, 1494–1502 RSC.
- R. S. Li, B. F. Yuan, J. H. Liu, M. L. Liu, P. F. Gao, Y. F. Li, M. Li and C. Z. Huang, J. Mater. Chem. B, 2017, 5, 8719–8724 RSC.
- Y. Chong, Y. F. Ma, H. Shen, X. L. Tu, X. Zhou, J. Y. Xu, J. W. Dai, S. J. Fan and Z. J. Zhang, Biomaterials, 2014, 35, 5041–5048 CrossRef CAS PubMed.
- J. Dong, Y. L. Zhao, K. Q. Wang, H. Y. Chen, L. Liu, B. L. Sun, M. F. Yang, L. P. Sun, Y. Wang, X. G. Yu and L. F. Dong, ChemistrySelect, 2018, 3, 12696–12703 CrossRef CAS.
- X. Y. Tan, Y. C. Li, X. H. Li, S. X. Zhou, L. Z. Fan and S. H. Yang, Chem. Commun., 2015, 51, 2544–2546 RSC.
- M. Zheng, S. B. Ruan, S. Liu, T. T. Sun, D. Qu, H. F. Zhao, Z. G. Xie, H. L. Gao, X. B. Jing and Z. C. Sun, ACS Nano, 2015, 9, 11455–11461 CrossRef CAS PubMed.
- Y. P. Zhang, J. M. Ma, Y. S. Yang, J. X. Ru, X. Y. Liu, Y. Ma and H. C. Guo, Spectrochim. Acta, Part A, 2019, 217, 60–67 CrossRef CAS PubMed.
- C. F. Hu, Y. L. Liu, Y. H. Yang, J. H. Cui, Z. R. Huang, Y. L. Wang, L. F. Yang, H. B. Wang, Y. Xiao and J. H. Rong, J. Mater. Chem. B, 2013, 1, 39–42 RSC.
- M. Zhang, W. T. Wang, Y. J. Cui, N. L. Zhou and J. Shen, ACS Biomater. Sci. Eng., 2018, 4, 151–162 CrossRef CAS.
- H. C. Yao, W. W. Zhao, S. G. Zhang, X. F. Guo, Y. Li and B. Du, J. Mater. Chem. B, 2018, 6, 3107–3115 RSC.
- Y. Song, X. Yan, Z. H. Li, L. B. Qu, C. Z. Zhu, R. F. Ye, S. Q. Li, D. Du and Y. H. Lin, J. Mater. Chem. B, 2018, 6, 3181–3187 RSC.
- Y. Q. Dong, C. Q. Chen, X. T. Zheng, L. L. Gao, Z. M. Cui, H. B. Yang, C. X. Guo, Y. W. Chi and C. M. Li, J. Mater. Chem., 2012, 22, 8764–8766 RSC.
- J. Peng, W. Gao, B. K. Gupta, Z. Liu, R. Romero-Aburto, L. H. Ge, L. Song, L. B. Alemany, X. B. Zhan, G. H. Gao, S. A. Vithayathil, B. A. Kaipparettu, A. A. Marti, T. Hayashi, J. J. Zhu and P. M. Ajayan, Nano Lett., 2012, 12, 844–849 CrossRef CAS PubMed.
- Z. T. Fan, Y. C. Li, X. H. Li, L. Z. Fan, S. X. Zhou, D. C. Fang and S. H. Yang, Carbon, 2014, 70, 149–156 CrossRef CAS.
- S. Sun, L. Zhang, K. Jiang, A. G. Wu and H. W. Lin, Chem. Mater., 2016, 28, 8659–8668 CrossRef CAS.
- Q. Liu, B. D. Guo, Z. Y. Rao, B. H. Zhang and J. R. Gong, Nano Lett., 2013, 13, 2436–2441 CrossRef CAS PubMed.
- P. J. Fairchild, K. F. Nolan, S. Cartland and H. Waldmann, Int. Immunopharmacol., 2005, 5, 13–21 CrossRef CAS PubMed.
- G. C. Parker, M. Anastassova-Kristeva, H. E. Broxmeyer, W. H. Dodge, L. M. Eisenberg, U. M. Gehling, L. M. Guenin, R. Huss, N. I. Moldovan, M. Rao, E. F. Srour and M. C. Yoder, Stem Cells Dev., 2004, 13, 579–584 CrossRef PubMed.
- W. H. Shang, X. Y. Zhang, M. Zhang, Z. T. Fan, Y. Sun, M. Han and L. Z. Fan, Nanoscale, 2014, 6, 5799–5806 RSC.
- F. L. Yuan, L. Ding, Y. C. Li, X. H. Li, L. Z. Fan, S. X. Zhou, D. C. Fang and S. H. Yang, Nanoscale, 2015, 7, 11727–11733 RSC.
- K. Jiang, S. Sun, L. Zhang, Y. Lu, A. Wu, C. Z. Cai and H. W. Lin, Angew. Chem., Int. Ed., 2015, 54, 5360–5363 CrossRef CAS PubMed.
- M. Havrdova, K. Hola, J. Skopalik, K. Tomankova, M. Petr, K. Cepe, K. Polakova, J. Tucek, A. B. Bourlinos and R. Zboril, Carbon, 2016, 99, 238–248 CrossRef CAS.
- K. Jiang, S. Sun, L. Zhang, Y. H. Wang, C. Z. Cai and H. W. Lin, ACS Appl. Mater. Interfaces, 2015, 7, 23231–23238 CrossRef CAS PubMed.
- L. H. Shi, Y. Y. Li, X. F. Li, X. P. Wen, G. M. Zhang, J. Yang, C. Dong and S. M. Shuang, Nanoscale, 2015, 7, 7394–7401 RSC.
- Y. E, L. Bai, L. Fan, M. Han, X. Zhang and S. Yang, J. Mater. Chem., 2011, 21, 819–823 RSC.
- B. Bao, A. Ahmad, A. S. Azmi, S. Ali and F. H. Sarkar, Curr. Protoc. Pharmacol., 2013, 61, 14.25.11–14.25.14 Search PubMed.
- T. Reya, S. J. Morrison, M. F. Clarke and I. L. Weissman, Nature, 2001, 414, 105–111 CrossRef CAS PubMed.
- E. M. De Francesco, F. Sotgia and M. P. Lisanti, Biochem. J., 2018, 475, 1611–1634 CrossRef CAS PubMed.
- R. H. Guo, S. X. Zhou, Y. C. Li, X. H. Li, L. Z. Fan and N. H. Voelcker, ACS Appl. Mater. Interfaces, 2015, 7, 23958–23966 CrossRef CAS.
- S. T. Yang, L. Cao, P. G. Luo, F. S. Lu, X. Wang, H. F. Wang, M. J. Meziani, Y. F. Liu, G. Qi and Y. P. Sun, J. Am. Chem. Soc., 2009, 131, 11308–11309 CrossRef CAS PubMed.
- P. G. Luo, S. Sahu, S. T. Yang, S. K. Sonkar, J. P. Wang, H. F. Wang, G. E. LeCroy, L. Cao and Y. P. Sun, J. Mater. Chem. B, 2013, 1, 2116–2127 RSC.
- L. Cao, S. T. Yang, X. Wang, P. G. Luo, J. H. Liu, S. Sahu, Y. M. Liu and Y. P. Sun, Theranostics, 2012, 2, 295–301 CrossRef CAS PubMed.
- Y. P. Li, T. Y. Lin, Y. Luo, Q. Q. Liu, W. W. Xiao, W. C. Guo, D. Lac, H. Y. Zhang, C. H. Feng, S. Wachsmann-Hogiu, J. H. Walton, S. R. Cherry, D. J. Rowland, D. Kukis, C. Pan and K. S. Lam, Nat. Commun., 2014, 5, 4712 CrossRef CAS.
- L. V. Wang and S. Hu, Science, 2012, 335, 1458–1462 CrossRef CAS.
- F. Liu, T. L. Liu, X. X. Xu, X. Y. Guo, N. Li, C. Y. Xiong, C. Li, H. Zhu and Z. Yang, Mol. Pharmaceutics, 2018, 15, 619–628 CrossRef CAS PubMed.
- H. Zhu, C. K. Zhao, F. Liu, L. X. Wang, J. N. Feng, C. C. Shou and Z. Yang, ACS Med. Chem. Lett., 2017, 8, 266–269 CrossRef CAS PubMed.
- Y. Wang, H. Song, G. Wang, X. Yang, J. Wang and H. Wei, J. Radioanal. Nucl. Chem., 2019, 319, 1119–1125 CrossRef CAS.
- S. P. Song, Y. Qin, Y. He, Q. Huang, C. H. Fan and H. Y. Chen, Chem. Soc. Rev., 2010, 39, 4234–4243 RSC.
- U. Resch-Genger, M. Grabolle, S. Cavaliere-Jaricot, R. Nitschke and T. Nann, Nat. Methods, 2008, 5, 763 CrossRef CAS.
- S. A. Gomes, C. S. Vieira, D. B. Almeida, J. R. SantosMallet, R. F. Menna-Barreto, C. L. Cesar and D. Feder, Sensors, 2011, 11, 11664–11678 CrossRef CAS PubMed.
- C. E. Bradburne, J. B. Delehanty, K. Boeneman Gemmill, B. C. Mei, H. Mattoussi, K. Susumu, J. B. Blanco-Canosa, P. E. Dawson and I. L. Medintz, Bioconjugate Chem., 2013, 24, 1570–1583 CrossRef CAS.
- X. X. Wu, S. S. Sun, Y. H. Wang, J. L. Zhu, K. Jiang, Y. M. Leng, Q. H. Shu and H. W. Lin, Biosens. Bioelectron., 2017, 90, 501–507 CrossRef CAS PubMed.
- C. X. Wang, K. L. Jiang, Q. Wu, J. P. Wu and C. Zhang, Chem. – Eur. J., 2016, 22, 14475–14479 CrossRef CAS PubMed.
- W. L. Gao, Y. M. Zhou, C. G. Xu, M. X. Guo, Z. C. Qi, X. J. Peng and B. Gao, Sens. Actuators, B, 2019, 281, 905–911 CrossRef CAS.
- W. L. Gao, H. H. Song, X. Wang, X. Q. Liu, X. B. Pang, Y. M. Zhou, B. Gao and X. J. Peng, ACS Appl. Mater. Interfaces, 2018, 10, 1147–1154 CrossRef CAS PubMed.
- X. Bao, Y. Yuan, J. Q. Chen, B. H. Zhang, D. Li, D. Zhou, P. T. Jing, G. Y. Xu, Y. L. Wang, K. Holá, D. Shen, C. F. Wu, L. Song, C. B. Liu, R. Zbořil and S. N. Qu, Light: Sci. Appl., 2018, 7, 91 CrossRef PubMed.
- W. M. Girma, K. Dehvari, Y. C. Ling and J. Y. Chang, Mater. Sci. Eng., C, 2019, 101, 179–189 CrossRef CAS PubMed.
- X. N. Jing, Z. Zhi, L. M. Jin, F. Wang, Y. S. Wu, D. Q. Wang, K. Yan, Y. P. Shao and L. J. Meng, Nanoscale, 2019, 11, 9457–9467 RSC.
- I. L. Christensen, Y. P. Sun and P. Juzenas, J. Biomed. Nanotechnol., 2011, 7, 667–676 CrossRef CAS PubMed.
- Z. M. Markovic, B. Z. Ristic, K. M. Arsikin, D. G. Klisic, L. M. Harhaji-Trajkovic, B. M. Todorovic-Markovic, D. P. Kepic, T. K. Kravic-Stevovic, S. P. Jovanovic, M. M. Milenkovic, D. D. Milivojevic, V. Z. Bumbasirevic, M. D. Dramicanin and V. S. Trajkovic, Biomaterials, 2012, 33, 7084–7092 CrossRef CAS PubMed.
- P. Agostinis, K. Berg, K. A. Cengel, T. H. Foster, A. W. Girotti, S. O. Gollnick, S. M. Hahn, M. R. Hamblin, A. Juzeniene, D. Kessel, M. Korbelik, J. Moan, P. Mroz, D. Nowis, J. Piette, B. C. Wilson and J. Golab, Ca-Cancer J. Clin., 2011, 61, 250–281 CrossRef.
- J. C. Ge, Q. Y. Jia, W. M. Liu, L. Guo, Q. Y. Liu, M. H. Lan, H. Y. Zhang, X. M. Meng and P. F. Wang, Adv. Mater., 2015, 27, 4169–4177 CrossRef CAS.
- J. P. Celli, B. Q. Spring, I. Rizvi, C. L. Evans, K. S. Samkoe, S. Verma, B. W. Pogue and T. Hasan, Chem. Rev., 2010, 110, 2795–2838 CrossRef CAS PubMed.
- M. Höckel and P. Vaupel, J. Natl. Cancer I., 2001, 93, 266–276 CrossRef.
- Q. Y. Jia, J. C. Ge, W. M. Liu, X. L. Zheng, S. Q. Chen, Y. M. Wen, H. Y. Zhang and P. F. Wang, Adv. Mater., 2018, 30, 1706090 CrossRef PubMed.
- K. Hola, Y. Zhang, Y. Wang, E. P. Giannelis, R. Zboril and A. L. Rogach, Nano Today, 2014, 9, 590–603 CrossRef CAS.
- J. C. Qiu, R. B. Zhang, J. H. Li, Y. H. Sang, W. Tang, P. Rivera Gil and H. Liu, Int. J. Nanomed., 2015, 10, 6709–6724 CAS.
- C. E. Dunbar, K. A. High, J. K. Joung, D. B. Kohn, K. Ozawa and M. Sadelain, Science, 2018, 359, eaan4672 CrossRef PubMed.
- C. L. Hardee, L. M. Arevalo-Soliz, B. D. Hornstein and L. Zechiedrich, Genes, 2017, 8, 65 CrossRef PubMed.
- S. M. Ghafary, M. Nikkhah, S. Hatamie and S. Hosseinkhani, Sci. Rep., 2017, 7, 9552 CrossRef PubMed.
Footnote |
† These authors contributed equally to this work. |
|
This journal is © the Partner Organisations 2020 |