DOI:
10.1039/D0QI00728E
(Research Article)
Inorg. Chem. Front., 2020,
7, 4062-4069
A bioorthogonal time-resolved luminogenic probe for metabolic labelling and imaging of glycans†
Received
21st June 2020
, Accepted 31st August 2020
First published on 4th September 2020
Abstract
The use of bioorthogonal fluorogenic probes is superior to labelling and imaging of biomolecules in live cells and organisms, although overcoming the limitation of autofluorescence is still a challenge for current probes to achieve high illumination resolution of the target of interest. We herein demonstrate a functionalized terbium complex Tb-1 that is stable and biocompatible to enable bioorthogonal ligation with engineered cell-surface glycans for providing responsive luminescence. A luminescence resonance energy transfer (LRET) quencher with bioorthogonal properties is strategically incorporated into a tripodal terbium complex with low toxicity, which can undergo a click-cycloaddition reaction with a cyclooctene to completely change the electronic structure of the quencher, resulting in a much less efficient LRET but a 5-fold enhancement in the long-lived terbium emission intensity. This work therefore establishes a time-resolved platform that enables labelling and imaging of the biomolecules of interest.
Introduction
Bioorthogonal chemistry, pioneered by Prescher and Bertozzi, refers to chemical reactions within cells that do not affect the physiological processes in place.1,2 The ideal visualization of various biological processes taking place in living systems should be performed without any interference from the imaging probes. By metabolic engineering,3 a chemical reporter, which is usually a small functional group that causes minimal structural changes to the substrate and does not interfere with its functions, can be incorporated into the target substrate, such as a glycan, in its biosynthetic pathways.4 Glycans existing mostly in glycoproteins or glycolipids via metabolic engineering play vital roles in various biological events.5–8 Subsequently, a fluorogenic labeling or an imaging probe carrying another functional group capable of reacting specifically with the chemical reporter would be introduced, and the cell surface glycans can be labeled without any interference or distress to the physiological environment.9 There are reports of various types of fluorogenic bioorthogonal reporters,10,11 such as tetrazine,12,13 azide,14,15 alkenes,16,17 cycloalkynes18,19 and cyclopropenes,20 that exhibit specific and click reactions to perform bioorthogonal ligation. However, the major drawback associated with conventional fluorescent dyes lies in the interference of autofluorescence.
Various imaging techniques have been utilized in the imaging of glycans, including stochastic optical reconstruction microscopy (STORM) imaging,21 magnetic resonance (MR) imaging22 and fluorescence imaging.23–26 The development of both bioorthogonal chemical reporters and labelling agents has gathered pace in the past decade as researchers hope to increase their specificity and reactivity by introducing new pairs of bioorthogonal conjugators and to gain better resolution in the visualization of the substrate.
Trivalent lanthanides, in particular europium(III) and terbium(III), have been commonly used as emission centers due to their characteristic emission profiles and long emission lifetimes (μs–ms time frame).27–30 The fingerprint emission bands allow differentiation from the broad and structureless emission bands of organic chromophores and convincingly distinguish lanthanide emissions from short-lived biological autofluorescence (ns-based) using time-resolved measurements.31–33 The technique of time-resolved spectroscopy makes use of a time-gated system to delay data acquisition of pulse-excited emission signals so that the detection window is only opened after the unwanted emissions have faded, and the desired longer-lived emission(s) are distinctly detected.34–36 The lanthanide probes have gained great momentum for application in biological detection and imaging, especially the “switch-off” strategy based on the principle of photoinduced electron transfer or antenna–analyte electron transfer.27,37 Recently, lanthanide complexes incorporated with several functional groups have also been covalently linked to metabolically engineered substrates via bioorthogonal ligation to perform time-resolved imaging and labelling of cell surface glycans in order to obtain more information on how glycans regulate progression.38–40 However, these complexes with “always-on” luminescence signals suffer from the interference of unremoved complexes. Therefore, to obtain high signal/background (S/B) ratios, the development of a luminogenic lanthanide probe that can be activated by a bioorthogonal reaction is highly desirable. Furthermore, time-resolved luminescence spectroscopy allows the probing of the excited state dynamics and deactivation pathways of our designed imaging complexes, offering complementary evidence for our bioorthogonal ligation mechanism.
To improve the imaging efficacy via combining bioorthogonal chemistry with lanthanide emission, herein, we report the design and synthesis of a luminogenic tetrazine-based terbium(III) probe (Tb-1, Scheme 1). Tb-1 was found to be activated via a bioorthogonal tetrazine ligation and exhibited dramatic enhancement of long-lived luminescence (5-fold) upon cycloaddition reaction with a strained trans-cyclooctene (TCO). Notably, Tb-1 could enable robust and sensitive detection of cyclooctene-modified glycome under no-wash conditions in living cells and zebrafish.
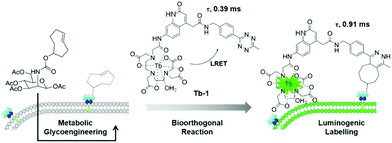 |
| Scheme 1 Schematic illustration of the bioorthogonal time-resolved luminogenic probe Tb-1 for metabolic labelling and imaging of glycans. | |
Results and discussion
As the absorption of tetrazine overlaps well with the emission of Tb3+ (Fig. S1†), we rationally hypothesized that the tetrazine-functional group could quench the luminescence of terbium via luminescence resonance energy transfer (LRET) between the terbium compound and the tetrazine moiety. To confirm the hypothesis, we also synthesized and characterized a reference complex Tb-M without the tetrazine moiety together with the probe Tb-1 based on a 7-substituted quinolinone antenna (Fig. 1 and Fig. S2–S13†).
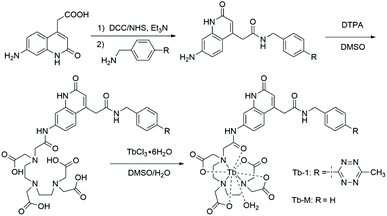 |
| Fig. 1 The synthesis route to Tb-M and Tb-1. | |
Typically, Tb-M and Tb-1 exhibited a similar quinolinone absorption band, located at 348 and 346 nm, respectively (Table 1). Unlike the colorless Tb-M, the pale red Tb-1 exhibited an absorption band centered at 522 nm,44,45 which is attributed to the tetrazine moiety (Table 1). On the other hand, there were no energy transfer pathways between the tetrazine moiety and antenna ligand (Fig. S14†). The emission quantum yield (λex = 340 nm) of Tb-1 showed a dramatic difference to that of Tb-M. Tb-M exhibited a strong green emission with a quantum yield of 10.7% in aqueous solution, while Tb-1 showed only very weak Tb emission under identical experimental conditions (Φ = 1.0%). The room temperature emission spectra of Tb-M and Tb-1 under the same excitation wavelength as the antenna ligand are given in Fig. S15,† which shows the characteristic 5D4 → 7FJ (J = 3, 4, 5, 6) transitions of Tb3+. The ratios of the 5D4 → 7FJ emissions from Tb-M and Tb-1 indicate that both complexes have similar coordination geometry. As for Tb-1, there were two steps in the energy transfer pathways including the energy transferred from antenna ligands to Tb3+, and further from Tb3+ to tetrazine. On the basis of the reported methods,29,46 the sensitization and LRET efficiency of these was obtained as 63.21% and 46.5%, respectively. By measuring the phosphorescence spectra of La-H and La-1 obtained at 77 K, we found that the two complexes exhibited a similar phosphorescence band of the antenna ligand at 445 nm (Fig. S16†), indicating that the energy level of the T1 excited states of the antenna chromophores are around 22
472 cm−1 and fall in the optimum energy transfer range of the 5D4 excited state of Tb3+ (20
400 cm−1). The quenched Tb3+ emission in Tb-1 is thereby attributed to LRET energy transfer from the excited Tb3+ state to the excited state of tetrazine (19
157 cm−1). Once the tetrazine binds with TCO, the excited state level of tetrazine–TCO would be higher than that of tetrazine, which could block the LRET process, resulting in enhancement of the Tb3+ luminescence emission. And the proposed energy transfer mechanism of Tb-1 is summarized in Fig. S17.†
Table 1 Photophysical parameters of the Tb(III) complexes
|
λ
abs a/nm |
ε
/M−1 cm−1 |
τ(H2O)c/ms |
τ(D2O)c/ms |
Φ
/% |
Measured in DI H2O.
Absorption coefficient, 298 K.
Tb emission decay (λem = 545 nm, 5D4 → 7F5, λex = 340 nm, 5 mM); hydration number of Tb(III) complexes, q = 1.2 × [k(H2O) − k(D2O) − 0.25], k = τ−1.41,42
Overall Tb emission quantum yield in H2O, determined by integrating sphere.43
With the addition of 20 equivalents TCO.
|
Tb-M
|
348 |
12 113 |
1.06 |
1.32 |
10.7 ± 0.6 |
Tb-1
|
346, 522 |
10 188, 1132 |
0.39 |
0.89 |
1.0 ± 0.8 |
Tb-1 + TCOe |
347 |
10 312 |
0.91 |
— |
9.0 ± 0.5 |
Considering that the cycloadduct of tetrazine has almost no absorption in the region of interest, the LRET degree should be controlled by the bioorthogonal reaction of tetrazine and TCO (Fig. 2a). The absorption of Tb-1 in the range of 500–600 nm was then found to disappear upon titration, and simultaneously, the solution turned from red to colorless, indicating the loss of the tetrazine adduct, further confirming that the quenching of luminescence was attributed to LRET (Fig. 2b and Fig. S18†). The time-gated spectra of Tb-1 upon titrating with TCO clearly show that the probe responded well and achieved a 5-fold increase in luminescence intensity (Fig. 2c). The luminescence lifetime was extended from 0.39 to 0.91 ms in PBS buffer solution, which is sufficiently long to eliminate background fluorescence (Fig. 2d, Table 1 and Fig. S19†) and the number of metal-bound water molecules in solution can be calculated to be one via reported methods, which was further confirmed by the Fourier transform infrared (FTIR) spectrum, demonstrating that there is no difference in solvent quenching (Fig. S20†).47 A linear relationship between the emission intensity at 545 nm and the concentration of TCO from 0–4 μM was observed, and the response limit of Tb-1 for TCO in solution was then determined to be 19.6 nM by means of the reported method (Fig. S21†).48
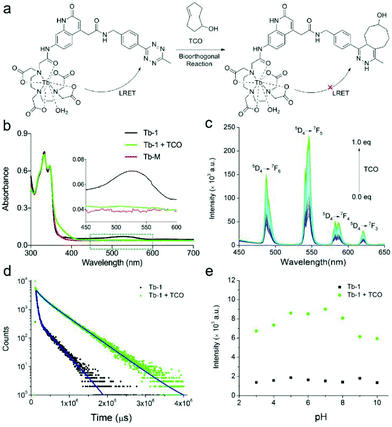 |
| Fig. 2 (a) Schematic of sensing bioorthogonal reaction of luminogenic Tb-1 with TCO based on the LRET mechanism. (b) The absorbance spectra of Tb-M, and Tb-1 in the absence or presence of TCO. (c) Time-gated luminescence spectra of Tb-1 titrating with TCO (λex = 340 nm). (d) Decay time curves of the 5D4 → 7F5 emission of Tb3+ (10 μM) in the absence and presence of TCO (20 μM). (e) The luminescence intensity (λem = 545 nm) of complex Tb-1 reacted with TCO in various pH buffers. | |
Next, the sensing of Tb-1 towards TCO was measured in a physiological environment with the pH ranging from 8.0 to 5.0 by monitoring the Tb3+ luminescence (Fig. 2e). The results showed that Tb-1 showed excellent responsiveness to TCO in such a physiological environment, despite the fact that pH-dependent emission was observed below 5.0 and over 8.0 due to the protonation and hydrolysis of the organic antenna.49 Meanwhile, the biostability of Tb-1 was also evaluated by measuring its luminescence in PBS solution under various conditions: PBS solution containing different serum concentrations (from 0 to 50%) were kept for different times (from 1 to 24 h). Persistent luminescence of the Tb-1 under these conditions was observed (Fig. S22†), indicating its high stability in the simulated physiological environments. Besides, the interference from unsaturated fatty acids including oleic acid or linoleic acid was studied by recording the luminescence intensity changes. And only the addition of TCO resulted in a prominent increase in the luminescence emission intensity within 60 min (Fig. S23†), which was because of the faster kinetics of reaction between tetrazine and TCO (3910 M−1 s−1, Fig. S24†) compared with the tetrazine and alkene.50 These performances strongly suggest that the bio-stable probe Tb-1 could be used as a preferable tool with higher sensitivity and a larger turn-on luminescence response to TCO.
Having shown that Tb-1 responds well to TCO in vitro, we then evaluated the capability of the probe in the labelling of specific biomolecules, glycans, in the living cells. The cytotoxicity of Tb-1 was firstly tested on different cell lines (such as A549 and EMT 6 or LO2 cells) by using the standard cell counting kit 8 (CCK-8) assays (Fig. S25†). The complex appeared to be quite nontoxic, with IC50 values of ca. 1 mmol: a level far above the normal working concentration of a probe. Then, A549 cells were treated with 50 μM peracetylated N-TCO-mannosamine (Ac4ManNTCO) that would be metabolically converted into the corresponding alkynylsialic acid bearing TCO groups on the cell surface for 72 h. Control cells were incubated with peracetylated mannosamine (Ac4ManNH2). After this, A549 cells pretreated with the Ac4ManNTCO or Ac4ManNH2 were incubated with Tb-1 for 3 h, and then imaging by fluorescence microscopy was performed without washing. The two-photon photophysical properties of some quinolinone-sensitized Tb complexes have been studied previously, further providing a means of reducing the interference from other biological matter by utilizing low energy near infrared (NIR) excitation.51–54 Two-photon excitation of Tb-1 with a NIR laser can also induce the typical Tb-1 emission in the presence of TCO at 298 K (Fig. S26†). Compared with Fig. 2c, a broad band arising from the intraligand transition is observed, and this band overlaps with the standard set of well-resolved narrow emission bands owing to the Tb3+ centered transitions due to the quinolinone fluorescence or phosphorescence at 298 K, which would only be partially quenched in the emission spectrum recorded at 77 K.47 As shown in Fig. 3a, A549 cells treated with Ac4ManNTCO and Tb-1 displayed a strong luminescence on the cell surface as observed by two-photon (λex = 730 nm) confocal microscopy, while the control cells labelled with Ac4ManNH2 exhibited only a very weak emission. The expression of TCO groups on sialoglycoproteins was then studied by Coomassie Brilliant Blue staining and western blot analysis (Fig. 3b and c). The results revealed that abundant TCO groups were generated via metabolic glycoengineering of proteins on the cell surface. The significant cell membrane labelling and imaging over the background is attributed to the good luminogenic behavior of Tb-1. Flow cytometry analysis of the A549 cells treated with Ac4ManNTCO and fluorescein isothiocyanate (FITC)-tetrazine further confirmed that the cells modified with TCO groups can be labelled and light-up dramatically (Fig. 3d).
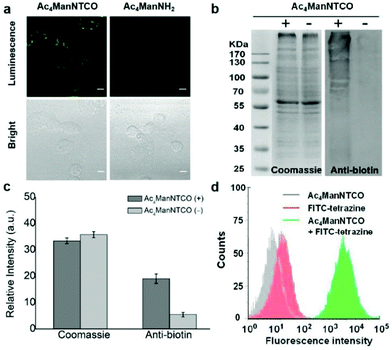 |
| Fig. 3 (a) Two-photon luminescence images of A549 cells after treatment with Ac4ManNTCO or Ac4ManNH2 (50 μM) for three days and further incubation with Tb-1 (50 μM) for 3 h. (b) Western blot analysis of lysates from A549 cells treated with Ac4ManNTCO or Ac4ManNH2 (50 μM). (c) Corresponding quantified intensity from (b). (d) Flow cytometry analysis of A549 cells pretreated with Ac4ManNTCO or not, followed by incubation with FITC-tetrazine. | |
Next, our tetrazine-functionalized luminogenic probe Tb-1 was further employed for glycome imaging in living systems, and zebrafish was selected as a typical proof-of-concept visualization model due to its advantages of well characterized morphology, optical transparency and high homology with mammals.55 In order to label sialylated glycans in zebrafish, its embryos were microinjected with 10 nL medium containing 5 mM Ac4ManNTCO for 72 h post-fertilization, resulting in metabolic labelling of O-linked sialylated glycans with TCO. We then incubated the embryos with Tb-1 for 3 h and imaged them by confocal microscopy (Fig. 4a and b). Notably, a very sharp luminescence signal was observed in the enveloping cell membranes of zebrafish pre-labeled with TCO–sialic acid, whereas there was no significant emission in control groups treated with Ac4ManNH2. And dose-dependent staining was observed in fishes treated with 0–5 mM Ac4ManNTCO (Fig. 4c and Fig. S27†). As a consequence, Tb-1 could be used as a valuable and efficient luminogenic probe for TCO-labelled glycomes in vivo via a two-step imaging procedure.
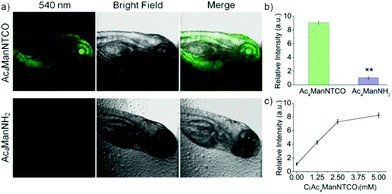 |
| Fig. 4 (a) Two-photon emission images of the Ac4ManNTCO (top row) or Ac4ManNH2 (bottom row) labelled zebrafish embryo at 72 h after fertilization. (b) Corresponding quantified intensity from (a). (c) Plots of the dose-dependent generation of TCO groups on the zebrafish after treatment with varied concentrations of Ac4ManNTCO. | |
Conclusions
In conclusion, we have presented a terbium(III) complex Tb-1 as a novel luminogenic bioorthogonal probe. The probe is weakly emissive due to luminescence resonance energy transfer (LRET) to the tetrazine group. However, it showed dramatic emission enhancement upon cycloaddition reaction with a strained cyclooctene by altering the tetrazine structure and regaining the antenna effect. Interestingly, this tetrazine complex was also identified as a luminogenic bioorthogonal label and imaging reagent for cyclooctene-modified glycomes. We hope that these results will pave the way for future site-specific protein labelling and imaging in live cells.
Experimental section
Materials and instruments
Materials.
TbCl3·6H2O (99%), diethylenetriaminepentaacetic acid (DTPA, ≥98%), NaOH (≥98%), N-hydroxysuccinimide (NHS, ≥98%), LaCl3·7H2O (99.99%), N,N-dimethylformamide (DMF, 99%), dimethyl sulfoxide (DMSO, 99%) and 1-ethyl-3-(3-dimethylaminopropyl)carbodiimide hydrochloride (EDC, ≥98%) were purchased from Aladdin Reagent, Ltd (Shanghai, China); and trans-3-hydroxyl–cyclooctene (TCO, ≥98%) and TCO-NHS (≥98%) were purchased from Ruixi Biological Technology Co., Ltd (Xi'an, China). CCK-8 was obtained from Dojindo (Japan). Dulbecco's modified Eagle's medium (DMEM) cell culture medium, fetal bovine serum, streptomycin, and penicillin were purchased from Thermo Fisher Scientific Co., Ltd (China). All chemicals used in this study were of analytical grade and reagents were used without further purification. The compound Ac4ManNTCO was prepared (Fig. S28a†) according to our previous literature.56 La-H and La-1 were introduced via coordinating between the central atom lanthanum ion (La3+) and the ligand of Tb-M and Tb-1, respectively (Fig. S28b†). All aqueous solutions were prepared using ultrapure deionized water (DI water), which was obtained through a Millipore Milli-Q water purification system (Billerica, USA) and had an electric resistance >18.2 MΩ.
Instruments and characterization.
The 1H NMR spectra were recorded on a Bruker Ultrashield 400 Plus NMR spectrometer. All chemical shifts are reported in the standard δ notation of parts per million. The UV–vis absorption spectra were recorded on a UV–vis spectrometer (Lambda 35 UV–vis spectrometer, PerkinElmer, USA) at room temperature. The emission lifetime measurements of Tb-1 and Tb-M were recorded with a FLS-1000 fluorescence spectrophotometer (Edinburgh Instruments, Britain). High-performance liquid chromatography (HPLC) with absorbance detection was carried out using an Agilent7890B column and acetonitrile as the eluent. FT-IR spectra were recorded with KBr pellets on a Bio-Rad FTS 6000 spectrometer (Bio-Rad Company, Hercules, California, USA) at room temperature. The confocal fluorescence images of cell and zebrafish were collected on an Olympus FluoView FV10 microscope (Olympus Imaging America Inc., Japan). Cell viability was measured using a microplate reader (Infinite 200, Tecan, Switzerland).
Synthesis of compound 1.
4-[(1,2,4,5-Tetrazin-3-yl)phenyl]-methanamine (tetrazine-benzylamine) and 7-amino-1,2-dihydro-2-oxo-4-quinoline acetic acid were produced according to the literature, respectively.57,58 Quinolinone acid (21.8 mg, 0.1 mmol) was reactivated with N-hydroxysuccinimide by adding 1.2 equiv. of NHS (13.8 mg, 0.12 mmol) and 1.2 equiv. of DCC (24.7 mg, 0.12 mmol) in dry DMF and reacted for 2 hours at room temperature. 1.1 equiv. of tetrazine-benzylamine (22.1 mg, 0.11 mmol) was added to the above solution and stirred at room temperature until the reaction reached completion (monitored by TLC). DMF was removed by rotary evaporation, and the crude mixture was purified by silica gel column chromatography with CH2Cl2/CH3CN (3
:
1–1
:
1, v/v) as the eluent yielding compound 1 (27.5 mg, 68.5%). 1H NMR (400 MHz, d6-DMSO): δ 11.23 (s, 1H), 8.74 (t, J = 4.0 Hz, 2H), 8.38 (d, J = 8.0 Hz, 2H), 7.49 (d, J = 8.0 Hz, 2H), 7.42 (d, J = 8.0 Hz, 1H), 6.44 (d, J = 8.0 Hz, 1H), 6.38 (s, 1H), 6.05 (s, 1H), 5.74 (d, J = 4.0 Hz, 2H), 4.41 (d, J = 4.0 Hz, 2H), 2.99 (s, 3H). ESI-MS (m/z): calcd for C21H19N7O2, 401.42 [M]; found, 402.2000.
Synthesis of compound 2.
1 equiv. of DTPA dianhydride (19.7 mg, 0.05 mmol) was dissolved in a mixture of DMSO, and 5 equiv. of Et3N (34 mL) under N2. 0.7 equiv. of compound 1 (14.1 mg, 0.035 mmol) dissolved in DMSO was added slowly to the above solution under vigorous stirring. The reaction mixture was stirred at room temperature for 2 h. After completion, the above solution was quenched by addition of 0.1 M HCl and pH was adjusted to 6.5. Then water was removed by rotary evaporation and the crude mixture was purified by ether precipitation yielding compound 2. 1H NMR (400 MHz, d6-DMSO): δ 11.23 (s, 1H), 7.95–8.54 (m, 7H), 7.51 (d, J = 4.0 Hz, 1H), 7.35 (d, J = 4.0 Hz, 1H), 6.42 (d, J = 8.0 Hz, 2H), 6.35 (s, 1H), 5.98 (d, J = 8.0 Hz, 1H), 4.38 (d, J = 4.0 Hz, 1H), 3.12 (d, J = 8.0 Hz, 8H), 2.43 (d, J = 4.0 Hz, 2H), 2.36 (s, 2H), 1.79 (s, 4H), 1.23 (s, 2H), 2.36 (t, J = 8.0 Hz, 1H). ESI-MS (m/z): calcd for C35H40N10O11, 776.75 [M]; found, 776.4000.
Synthesis of Tb-1.
Addition of metals: TbCl3·6H2O (5.0 mg, 0.01 mmol) was added to compound 2 (15.4 mg, 0.02 mmol) in a DMSO/H2O (2
:
1, v/v) solution, and pH was adjusted to 6.5 by addition of 0.1 M HCl, pH 6.5 buffer, and stirred for 3 h at room temperature. Then the water was removed by rotary evaporation and the crude mixture purified by ether precipitation affording Tb-1. ESI-MS (m/z): calcd for C35H36N10O11Tb3+, 931.64 [M]; found, 931.3000.
Calculation of the limit of detection (LOD).
LOD was calculated using the formula,48 LOD = 3δ/k. Here, δ represents the standard deviation of the fluorescence intensity of 3 blank solutions, and k represents the slope between fluorescence intensity and concentration.
Stability of Tb-1.
The stability of Tb-1 in the simulated physiological environment was detected as below: Tb-1 solution containing 0 to 50% serum (pH = 7.4) in PBS (pH 7.4) were kept for different times (from 1 to 24 h). The luminescence emission spectra were then recorded.
Cell culture.
Human lung adenocarcinoma cells (A549) and experimental mammary tumour-6 cells (EMT 6) were cultured in DMEM or normal human hepatic cell (LO2) were cultured in RIM1640 containing 10% fetal bovine serum and 1% penicillin/streptomycin at 37 °C in a humidified 5% CO2 atmosphere. The cell density was determined using a hemocytometer before experimentation.
Cytotoxicity evaluation in vitro.
Relative cell viabilities were determined by the standard CCK-8 assay. A549 cells and EMT6 or LO2 cells were seeded into 96-well plates (1 × 104 cells per well). And the cells were cultured for 12 h at 37 °C in a humidified incubator, 100 μL different concentrations of Tb-1 PBS (pH 7.4) solution were added into the wells. After this, the cells were incubated for another 12 h at 37 °C. Then the cells were washed using PBS buffer to remove the unbound compound, and fresh culture medium was added. After incubation at 37 °C for 12 h, solution containing 10% CCK-8 DMEM (100 μL) was added to these cells. After incubation for 2 h at 37 °C, OD450, the absorbance value at 450 nm, were measured with a microplate reader to determine cell viability.
Imaging glycans with cells.
A549 cells were first pretreated with Ac4ManNH2 (50 μM) or Ac4ManNTCO (50 μM) for 3 days and washed with PBS (pH 7.4) twice. This was followed by incubation with Tb-1 (50 μM) for 3 h at 37 °C in a humidified incubator and images were obtained using an Olympus FluoView FV10 microscope (Olympus Imaging America Inc., Japan) without washing. The two-photon excited luminescence of Tb3+ by a 730 nm laser was recorded at 500–560 nm.
Western blot analysis of cells.
A549 cells were pretreated with Ac4ManNH2 (50 μM) or Ac4ManNTCO (50 μM) for 3 days and washed with PBS (pH 7.4) twice, then harvested from the plates and centrifuged at 1800 rpm for 3 min. The cells were resuspended and incubated in 100 μL of lysis buffer (1% SDS, 100 mM Tris·HCl, pH 7.4) containing a protease inhibitor cocktail (Complete, EDTA-free) at 4 °C for 30 min and centrifuged for 20 min at 11
000 rpm to get supernatant solution. The solution lysates (5 mg mL−1, 50 μL) were incubated with tetrazine-biotin (5 μL, 5 mM in DPBS) for 6 h at 37 °C. Then the samples were resolved by 12% SDS/PAGE and transferred to pure nitrocellulose blotting membranes (BioTrace NT; Pall Life Science, Pensacola, FL, USA). The membranes were blocked using 10 mM Tris/HCl (pH 7.4), 150 mM NaCl, and 0.1% Tween-20 containing 5% nonfat milk for 1.5 h. Then, the membrane was incubated with streptavidin-HRP (diluted 1
:
2000 in TBST) overnight at 4 °C. The membrane was developed using a HPR-AEC western blotting substrate.
Flow cytometry analysis.
A549 cells were pretreated with Ac4ManNTCO (50 μM) or Ac4ManNH2 (50 μM) followed by tetrazine-FITC. Then, the cells were removed and washed with FACS buffer. 10
000 cells per sample were analysed on a FACS canto II flow cytometer (Becton Dickinson, Mountain View, CA, USA) with excitation at 488 nm.
Imaging glycans with zebrafish.
All animal experiments were performed in compliance with the National Institutes of Health (NIH) guidelines for the Care and Use of Laboratory Animals of South China Normal University, and the experiments were approved by the Animal Ethics Committee of South China Normal University. Zebrafish Husbandry: adult wild-type zebrafish were maintained at 28.5 °C on a 14 hour light and 10 hour dark cycle. Embryos were obtained from natural spawning and were maintained in embryo medium (EM; 150 mM NaCl, 0.5 mM KCl, 1.0 mM CaCl2, 0.37 mM KH2PO4, 0.05 mM Na2HPO4, 2.0 mM MgSO4, and 0.71 mM NaHCO3 in deionized water, pH 7.4).59
Zebrafish embryos at the one-cell stage were dechorionated by 1 mg mL−1 protease (1 mg mL−1 in embryo medium) at 4 hours post-fertilization (hpf). And then these embryos were microinjected into the yolk with 5 nL of 5 mM Ac4ManNTCO. Meanwhile, the embryos microinjected with 5 nL of 5 mM Ac4ManNH2 were used as control groups. The embryos were then cultured at 28 °C using the standard embryo culture protocol. PTU (131 μM) was included in the medium beginning at 12 hpf to inhibit melanin production. After 72 hpf, they were labeled with the TCO group on zebrafish using a similar strategy to that of cellular glycome labeling described above. The embryos were then removed from the Ac4ManNTCO or Ac4ManNH2 containing solution, rinsed, and incubated in embryo medium containing Tb-1 (100 μM) for 3 h at 28 °C. The embryos were anesthetized with tricaine (2.6 μM in embryo medium) and mounted between two cover slips in embryo medium containing 0.6% low melting point agarose, 2.6 μM tricaine, and 131 μM PTU. After this, the embryos were detected using microscopy with excitation at 730 nm.
Conflicts of interest
There are no conflicts to declare.
Acknowledgements
This research was supported by the National Natural Science Foundation of China (21771065), the Guangdong Special Support Program (2017TQ04R138), the Science and Technology Program of Guangzhou (2019050001), the Natural Science Foundation of Guangdong (2019A1515012021), the Pearl River Nova Program of Guangzhou, Guangdong Province, China (201806010189), the Hong Kong Research Grants Council (HKBU1230019) and the Hong Kong Baptist University (RC-ICRS/18-19/01), and CAS-Croucher Funding Scheme for Joint Laboratories (CAS 18204).
References
- J. A. Prescher and C. R. Bertozzi, Chemical Technologies for Probing Glycans, Cell, 2006, 126, 851–854 Search PubMed.
- J. A. Prescher and C. R. Bertozzi, Chemistry in Living Systems, Nat. Chem. Biol., 2005, 1, 13–21 Search PubMed.
- J. D. Keasling, Manufacturing molecules through metabolic engineering, Science, 2010, 330, 1355–1358 Search PubMed.
- P. V. Chang, J. A. Prescher, M. J. Hangauer and C. R. Bertozzi, Imaging cell surface glycans with bioorthogonal chemical reporters, J. Am. Chem. Soc., 2007, 129, 8400–8401 Search PubMed.
- R. Xie, L. Dong, Y. Du, Y. Zhu, R. Hua, C. Zhang and X. Chen, In vivo metabolic labeling of sialoglycans in the mouse brain by using a liposome-assisted bioorthogonal reporter strategy, Proc. Natl. Acad. Sci. U. S. A., 2016, 113, 5173–5178 Search PubMed.
- A. Shajahan, S. Parashar, S. Goswami, S. M. Ahmed, P. Nagarajan and S.-G. Sampathkumar, Carbohydrate-neuroactive hybrid strategy for the metabolic glycan engineering of the central nervous system in vivo, J. Am. Chem. Soc., 2017, 139, 693–700 Search PubMed.
- H. Wang, R. Wang, K. Cai, H. He, Y. Liu, J. Yen, Z. Wang, M. Xu, Y. Sun, X. Zhou, Q. Yin, L. Tang, I. T. Dobrucki, L. W. Dobrucki, E. J. Chaney, S. A. Boppart, T. M. Fan, S. Lezmi, X. Chen, L. Yin and J. Cheng, Selective in vivo metabolic cell-labeling-mediated cancer targeting, Nat. Chem. Biol., 2017, 13, 415–424 Search PubMed.
- D. Zhang, Y. S. Zheng, Z. Lin, X. L. Liu, J. Li, H. H. Yang and W. H. Tan, Equipping natural killer cells with specific targeting and checkpoint blocking aptamers for enhanced adoptive immunotherapy in solid tumors, Angew. Chem., Int. Ed., 2020, 59, 12022–12028 Search PubMed.
- J. Hassenruck and V. Wittmann, Cyclopropene derivatives of aminosugars for metabolic glycoengineering, Beilstein J. Org. Chem., 2019, 15, 584–601 Search PubMed.
- C. P. Ramil and Q. Lin, Bioorthogonal chemistry: Strategies and recent developments, Chem. Commun., 2013, 49, 11007–11022 Search PubMed.
- P. Shieh and C. R. Bertozzi, Design strategies for bioorthogonal smart probes, Org. Biomol. Chem., 2014, 12, 9307–9320 Search PubMed.
- S. Stairs, A. A. Neves, H. Stöckmann, Y. A. Wainman, H. Ireland-Zecchini, K. M. Brindle and F. J. Leeper, Metabolic glycan imaging by isonitrile-tetrazine click chemistry, ChemBioChem, 2013, 14, 1063–1067 Search PubMed.
- J. Yang, J. Šečkutė, C. M. Cole and N. K. Devaraj, Live-cell imaging of cyclopropene tags with fluorogenic tetrazine cycloadditions, Angew. Chem., Int. Ed., 2012, 51, 7476–7479 Search PubMed.
- Y. Y. Yang, J. M. Ascano and H. C. Hang, Bioorthogonal chemical reporters for monitoring protein acetylation, J. Am. Chem. Soc., 2010, 132, 3640–3641 Search PubMed.
- X. L. Hu, H. Y. Jin, X. P. He, T. D. James, G. R. Chen and Y. T. Long, Colorimetric and plasmonic detection of lectins using core-shell gold glyconanoparticles prepared by copper-free click chemistry, ACS Appl. Mater. Interfaces, 2015, 7, 1874–1878 Search PubMed.
- A. Niederwieser, A.-K. Späte, L. D. Nguyen, C. Jüngst, W. Reutter and V. Wittmann, Two-color glycan labeling of live cells by a combination of diels-alder and click chemistry, Angew. Chem., Int. Ed., 2013, 52, 4265–4268 Search PubMed.
- M. F. Debets, S. S. van Berkel, J. Dommerholt, A. J. Dirks, F. P. J. T. Rutjes and F. L. van Delft, Bioconjugation with strained alkenes and alkynes, Acc. Chem. Res., 2011, 44, 805–815 Search PubMed.
- S. Bernard, D. Audisio, M. Riomet, S. Bregant, A. Sallustrau, L. Plougastel, E. Decuypere, S. Gabillet, R. A. Kumar, J. Elyian, M. N. Trinh, O. Koniev, A. Wagner, S. Kolodych and F. Taran, Bioorthogonal click and release reaction of iminosydnones with cycloalkynes, Angew. Chem., Int. Ed., 2017, 56, 15612–15616 Search PubMed.
- J. Dommerholt, S. Schmidt, R. Temming, L. J. A. Hendriks, F. P. J. T. Rutjes, J. C. M. van Hest, D. J. Lefeber, P. Friedl and F. L. van Delft, Readily accessible bicyclononynes for bioorthogonal labeling and three-dimensional imaging of living cells, Angew. Chem., Int. Ed., 2010, 49, 9422–9425 Search PubMed.
- D. M. Patterson, L. A. Nazarova, B. Xie, D. N. Kamber and J. A. Prescher, Functionalized cyclopropenes as bioorthogonal chemical reporters, J. Am. Chem. Soc., 2012, 134, 18638–18643 Search PubMed.
- S. Letschert, A. Göhler, C. Franke, N. Bertleff-Zieschang, E. Memmel, S. Doose, J. Seibel and M. Sauer, Super-resolution imaging of plasma membrane glycans, Angew. Chem., Int. Ed., 2014, 53, 10921–10924 Search PubMed.
- C. Witte, V. Martos, H. M. Rose, S. Reinke, S. Klippel, L. Schröder and C. P. R. Hackenberger, Live-cell MRI with xenon hyper-cest biosensors targeted to metabolically labeled cell-surface glycans, Angew. Chem., Int. Ed., 2015, 54, 2806–2810 Search PubMed.
- M. Sawa, T. L. Hsu, T. Itoh, M. Sugiyama, S. R. Hanson, P. K. Vogt and C. H. Wong, Glycoproteomic probes for fluorescent imaging of fucosylated glycans in vivo, Proc. Natl. Acad. Sci. U. S. A., 2006, 103, 12371–12376 Search PubMed.
- S. T. Laughlin, J. M. Baskin, S. L. Amacher and C. R. Bertozzi, In vivo imaging of membrane-associated glycans in developing zebrafish, Science, 2008, 320, 664 Search PubMed.
- S. Park, J. W. Sung and I. Shin, Fluorescent Glycan derivatives: Their use for natural glycan microarrays, ACS Chem. Biol., 2009, 4, 699–701 Search PubMed.
- H. Stöckmann, A. A. Neves, S. Stairs, H. Ireland-Zecchini, K. M. Brindle and F. J. Leeper, Development and evaluation of new cyclooctynes for cell surface glycan imaging in cancer cells, Chem. Sci., 2011, 2, 932–936 Search PubMed.
- M. C. Heffern, L. M. Matosziuk and T. J. Meade, Lanthanide probes for bioresponsive imaging, Chem. Rev., 2014, 114, 4496–4539 Search PubMed.
- T. Zhang, X. Zhu, C. C. W. Cheng, W.-M. Kwok, H. L. Tam, J. Hao, D. W. J. Kwong, W.-K. Wong and K.-L. Wong, Water-soluble mitochondria-specific ytterbium complex with impressive NIR emission, J. Am. Chem. Soc., 2011, 133, 20120–20122 Search PubMed.
- D. Kovacs, X. Lu, L. S. Mészáros, M. Ott, J. Andres and K. E. Borbas, Photophysics of coumarin and carbostyril-sensitized luminescent lanthanide complexes: implications for complex design in multiplex detection, J. Am. Chem. Soc., 2017, 139, 5756–5767 Search PubMed.
- E. M. Surender, S. J. Bradberry, S. A. Bright, C. P. McCoy, D. C. Williams and T. Gunnlaugsson, Luminescent lanthanide cyclen-based enzymatic assay capable of diagnosing the onset of catheter-associated urinary tract infections both in solution and within polymeric hydrogels, J. Am. Chem. Soc., 2017, 139, 381–388 Search PubMed.
- H. M. Burke, T. Gunnlaugsson and E. M. Scanlan, Glycosylated lanthanide cyclen complexes as luminescent probes for monitoring glycosidase enzyme activity, Org. Biomol.
Chem., 2016, 14, 9133–9145 Search PubMed.
- K. Y. Zhang, Q. Yu, H. Wei, S. Liu, Q. Zhao and W. Huang, Long-lived emissive probes for time-resolved photoluminescence bioimaging and biosensing, Chem. Rev., 2018, 118, 1770–1839 Search PubMed.
- J. Zheng, Y. Wu, D. Xing and T. Zhang, Synchronous detection of glutathione/hydrogen peroxide for monitoring redox status in vivo with a ratiometric upconverting nanoprobe, Nano Res., 2019, 12, 931–938 Search PubMed.
- S. F. Xue, Z. H. Chen, X. Y. Han, Z. Y. Lin, Q. X. Wang, M. Zhang and G. Shi, DNA encountering terbium(III): A smart “Chemical nose/tongue” For large-scale time-gated luminescent and lifetime-based sensing, Anal. Chem., 2018, 90, 3443–3451 Search PubMed.
- J. C. G. Bünzli, Lanthanide luminescence for biomedical analyses and imaging, Chem. Rev., 2010, 110, 2729–2755 Search PubMed.
- D. Jin and J. A. Piper, Time-gated luminescence microscopy allowing direct visual inspection of lanthanide-stained microorganisms in background-free condition, Anal. Chem., 2011, 83, 2294–2300 Search PubMed.
- A. B. Aletti, D. M. Gillen and T. Gunnlaugsson, Luminescent/colorimetric probes and (chemo-) sensors for detecting anions based on transition and lanthanide ion receptor/binding complexes, Coord. Chem. Rev., 2018, 354, 98–120 Search PubMed.
- H. Stockmann, V. Todorovic, P. L. Richardson, V. Marin, V. Scott, C. Gerstein, M. Lake, L. Wang, R. Sadhukhan and A. Vasudevan, Cell-surface receptor-ligand interaction analysis with homogeneous time-resolved fret and metabolic glycan engineering: Application to transmembrane and gpi-anchored receptors, J. Am. Chem. Soc., 2017, 139, 16822–16829 Search PubMed.
- X. Ai, L. Lyu, Y. Zhang, Y. Tang, J. Mu, F. Liu, Y. Zhou, Z. Zuo, G. Liu and B. Xing, Remote regulation of membrane channel activity by site-specific localization of lanthanide-doped upconversion nanocrystals, Angew. Chem., Int. Ed., 2017, 56, 3031–3035 Search PubMed.
- L. Ruhe, S. Ickert, S. Beck and M. W. Linscheid, A new strategy for metal labeling of glycan structures in antibodies, Anal. Bioanal. Chem., 2018, 410, 21–25 Search PubMed.
- A. Beeby, I. M. Clarkson, R. S. Dickins, S. Faulkner, D. Parker, L. Royle, A. S. de Sousa, J. A. Gareth Williams and M. Woods, Non-radiative deactivation of the excited states of europium, terbium and ytterbium complexes by proximate energy-matched OH, NH and CH oscillators: an improved luminescence method for establishing solution hydration states, J. Chem. Soc., Perkin Trans. 2, 1999, 493–504 Search PubMed.
- W. D. Horrocks Jr. and D. R. Sudnick, Lanthanide ion probes of structure in biology. Laser-induced luminescence decay constants provide a direct measure of the number of metal-coordinated water molecules, J. Am. Chem. Soc., 1979, 101, 334–340 Search PubMed.
- J. C. de Mello, H. F. Wittmann and R. H. Friend, An improved experimental determination of external photoluminescence quantum efficiency, Adv. Mater., 1997, 9, 230–232 Search PubMed.
- A. Wieczorek, T. Buckup and R. Wombacher, Rigid tetrazine fluorophore conjugates with fluorogenic properties in the inverse electron demand diels-alder reaction, Org. Biomol. Chem., 2014, 12, 4177–4185 Search PubMed.
- H. Wu and N. K. Devaraj, Advances in tetrazine bioorthogonal chemistry driven by the synthesis of novel tetrazines and dienophiles, Acc. Chem. Res., 2018, 51, 1249–1259 Search PubMed.
- P. Miluskia, M. Kochanowicz, J. Zmojdaa, A. Baranowska and D. Dorosz, Energy transfer of Tb(tmhd)3-Rhodamine B in poly(methyl methacrylate) fiber for new photonic applications, Opt. Mater., 2019, 87, 132–138 Search PubMed.
- J. B. M. R. Filho, P. R. Santos, J. A. Vale, W. M. Faustino, D. S. Farias, H. F. Brito, M. C. F. C. Felinto and E. E. S. Teotonio, Rare Earth-Indomethacinate Complexes with Heterocyclic
Ligands: Synthesis and Photoluminescence Properties, J. Braz. Chem. Soc., 2017, 12, 2281–2290 Search PubMed.
- X. H. Pan, Y. H. Zhao, T. T. Cheng, A. S. Zheng, A. B. Ge, L. X. Zang, K. H. Xu and B. Tang, Monitoring NAD(P)H by an ultrasensitive fluorescent probe to reveal reductive stress induced by natural antioxidants in HepG2 cells under hypoxia, Chem. Sci., 2019, 10, 8179–8186 Search PubMed.
- A. C. Knall and C. Slugovc, Inverse electron demand diels-alder (iedda)-initiated conjugation: a (high) potential click chemistry scheme, Chem. Soc. Rev., 2013, 42, 5131–5142 Search PubMed.
- U. Rieder and N. W. Luedtke, Alkene-Tetrazine Ligation for Imaging Cellular DNA, Angew. Chem., Int. Ed., 2014, 53, 9168–9172 Search PubMed.
- E. M. Surender, S. Comby, B. L. Cavanagh, O. Brennan, T. C. Lee and T. Gunnlaugsson, Two-photon luminescent bone imaging using europium nanoagents, Chem, 2016, 1, 438–455 Search PubMed.
- M. J. Davis, C. H. Kragor, K. G. Reddie, H. C. Wilson, Y. Zhu and T. M. Dore, Substituent effects on the sensitivity of a quinoline photoremovable protecting group to one- and two-photon excitation, J. Org. Chem., 2009, 74, 1721–1729 Search PubMed.
- X. Y. Chen, J. Shi, Y. M. Li, F. L. Wang, X. Wu, Q. X. Guo and L. Liu, Two-photon fluorescent probes of biological zn(II) derived from 7-hydroxyquinoline, Org. Lett., 2009, 11, 4426–4429 Search PubMed.
- Y. Mikata, A. Yamanaka, A. Yamashita and S. Yano, Isoquinoline-based TQEN family as TPEN-derived fluorescent zinc sensors, Inorg. Chem., 2008, 47, 7295–7301 Search PubMed.
- S. K. Ko, X. Chen, J. Yoon and I. Shin, Zebrafish as a good vertebrate model for molecular imaging using fluorescent probes, Chem. Soc. Rev., 2011, 40, 2120–2130 Search PubMed.
- R. J. Zhang, J. D. Zheng and T. Zhang, In vivo selective imaging of metabolic glycosylation with a tetrazine-modified upconversion nanoprobe, RSC Adv., 2020, 10, 15990–15996 Search PubMed.
- H.-S. Han, N. K. Devaraj, J. Lee, S. A. Hilderbrand, R. Weissleder and M. G. Bawendi, Development of a bioorthogonal and highly efficient conjugation method for quantum dots using tetrazine-norbornene cycloaddition, J. Am. Chem. Soc., 2010, 132, 7838–7839 Search PubMed.
- P. Ge and P. R. Selvin, Carbostyril derivatives as antenna molecules for luminescent lanthanide chelates, Bioconjugate Chem., 2004, 15, 1088–1094 Search PubMed.
- Y. Wu, G. Guo, J. Zheng, D. Xing and T. Zhang, Fluorogenic “photoclick” Labeling and imaging of dna with coumarin-fused tetrazole in vivo, ACS Sens., 2019, 4, 44–51 Search PubMed.
Footnote |
† Electronic supplementary information (ESI) available. See DOI: 10.1039/d0qi00728e |
|
This journal is © the Partner Organisations 2020 |
Click here to see how this site uses Cookies. View our privacy policy here.