DOI:
10.1039/D0QI00265H
(Review Article)
Inorg. Chem. Front., 2020,
7, 2107-2128
Correlation between crystal structures and polar (ferroelectric) properties of hybrids of haloantimonates(III) and halobismuthates(III)
Received
2nd March 2020
, Accepted 2nd April 2020
First published on 7th April 2020
Abstract
Halogenoantimonates(III) and halogenobismuthates(III) are a highly versatile class of organic–inorganic hybrid materials, applicable in optoelectronics and switchable dielectric devices. In this review, we discuss the rich chemistry of molecular–ionic halide complexes of Bi(III) and Sb(III) focusing on the correlations between their crystal structures and ferroelectric properties as well as on an explanation of the molecular mechanism of the paraelectric–ferroelectric phase transition. This review summarizes the current state of the art in the field of ferroelectricity among organic–inorganic hybrids based on Bi(III) and Sb(III) halides, which has become one of the key exploration areas of modern materials chemistry.
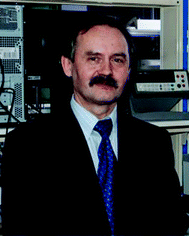 Ryszard Jakubas | Ryszard Jakubas was born in 1951 in Ząbkowice Śl, Poland. He received his Ph.D. in 1980 from the University of Wrocław (Physics and Chemistry Faculty). He has been a professor at the Chemistry Department at Wrocław University since 1998. In 1991 he took scientific internship at Université de Lille Sciences et Technologies, France. In 2009, he was appointed as the head of the Physical Chemistry Department (University of Wrocław). He is the co-author of about 350 original scientific articles in the field of physicochemistry of organic–inorganic hybrid materials. |
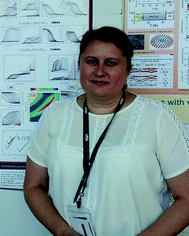 Magdalena Rok | Magdalena Rok was born in 1977 in Brzeg Dolny, Poland. She is an adjunct at the Faculty of Chemistry of the University of Wrocław. She obtained her Ph.D. in 2006 from the University of Wroclaw. She took scientific internships at the Jülich Center for Neutron Science JCNS (MLZ, Garching, Germany), where she conducted research on neutron scattering in molecular complexes. Her current research interest is in functional metal complexes and coordination polymers based on their structural phase transitions. She is a member of the Ferroelectric and Liquid Crystals Team. |
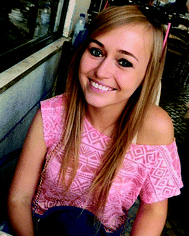 Klaudia Mencel | Klaudia Mencel was born in 1992 in Ostrów Wielkopolski, Poland. She received her M.Sc. degree at the Faculty of Chemistry of the University of Wrocław in 2016. She is a Ph.D. student in the Ferroelectrics and Liquid Crystals Team, Faculty of Chemistry (University of Wrocław). |
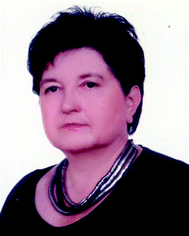 Grażyna Bator | Grażyna Bator was born in 1958 in Pleszew, Poland. She completed her Ph.D. in 1986 at the Faculty of Chemistry of the University of Wrocław, Poland. After presenting her habilitation dissertation in 2000, she obtained the title of Professor in 2007. She took scientific internships at KU Leuven in Belgium and ZIBJ in Dubna, FR, and carried out experiments on neutron scattering in cooperation with FRM II, Garching, Germany. She is the co-author of about 160 articles in the field of materials chemistry. She is the head of the specialization ‘Materials Chemistry for Modern Technologies’ at the Faculty of Chemistry of the University of Wrocław. |
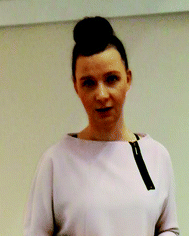 Anna Piecha-Bisiorek | Anna Piecha-Bisiorek was born in 1979 in Rybnik, Poland. She received her Ph.D. degree (supervisor: Prof. R. Jakubas) at the Faculty of Chemistry of the University of Wrocław in 2008 and became an assistant professor in 2018. She took scientific internships at the Jülich Center for Neutron Science JCNS (MLZ, Garching, Germany). Her research interests cover mainly the physicochemistry of organic–inorganic hybrid materials. |
1. Introduction
Materials whose physical properties can be controlled by external stimuli have attracted considerable interest due to their potential applications in substrate-film interfaces,1–3 high-strain states, electrocaloric circuits,4,5 nanotubes and nanowires,6 ferroelectric random access memories (FeRAMs),7 dynamic random access memory (DRAM) capacitors,8–10 electron emitters,2,8 solar cell components,11 and weak-magnetic field sensors.12–16 Ferroelectrics are of importance in the design of thermo-sensitive multifunctional switching materials because they are often accompanied by multiple switchable physical properties, e.g. piezoelectricity, pyroelectricity, dielectric constant and second harmonic generation (SHG). Ferroelectrics are polar substances in the solid state (crystalline or polymeric) or liquid-crystalline state (liquid crystals), in which a spontaneous polarization (Ps) is generated and it is reversible in an external electric field (E). The relationship between an electric shift (D) or polarization (P) and the electric field intensity leads to a hysteresis loop (D–E loop) between the opposite polarities. Such an electrical bistability can be used in non-volatile memory parts. It refers in particular to the ferroelectric computer memory type, FeRAM, which is crucial for the application of novel materials. In principle all ferroelectrics have a Curie temperature, Tc, connected with the phase changes from the ferroelectric to paraelectric phase but some of them decompose before achieving Tc. When the temperature approaches Tc, the dielectric constant fulfilling the Curie–Weiss law undergoes an abnormal increase; this is a quantity, which can be used in capacitors. Pyroelectricity is another important quantity. The change of the Ps with temperature, when the crystal is being heated or cooled down, generates electric current. The pyroelectric effect is particularly enhanced just below the Tc temperature and is used in temperature sensors and in detecting infrared radiation. With regard to ferroelectrics, they comprise, for instance, coupled electrical and mechanical phenomena. The stress generates electric polarization charge known as the piezoelectric effect, and the electric field generates stress (electrostatics). Large piezoelectric and electrostriction effects are used in various types of devices, e.g. piezoelectric elements and microsensors. In addition, polar structures are the source of second order optical nonlinearity, which affects SHG and the linear electro-optical effect (Pockels Effect).
A considerable effort has been made so far to understand the switchable molecular dynamics of polar organic cations confined in various polymeric coordination hosts. However, due to the lack of in-depth knowledge on the relationship between the phase transition (PT) and crystal structure, a rational design of molecular ferroelectrics still represents a great challenge, especially of hybrid molecular–ionic ferroelectrics based on polar organic cations embedded in inorganic networks. The general symmetry consideration of almost all physical phenomena is a very effective way for obtaining their description. Ferroelectricity is no exception. From this point of view, there are 230 space groups and 32 point groups, which describe both the macro- and microscopic symmetries of crystal structures. Among the 32 point groups, 11 belong to the centrosymmetric classes, while the remaining 21 are noncentrosymmetric.17 One should note, that the lack of spatial-inversion symmetry observed in the noncentrosymmetric group of crystals is most sought for in applications. It is important for the ferroelectric phase to adopt one of the 10 polar point groups, from which 68 polar space groups are formed (see Table 1).
Table 1 68 ferroelectric space groups belonging to the 10 polar point groups17
Crystal system |
Polar point group |
Space group |
Triclinic |
1 |
P1 |
Monoclinic |
2 |
m
|
P2, P21, C2 |
Pm, Pc, Cm, Cc
|
Orthorhombic |
mm2 |
Pmm2, Pmc21, Pcc2, Pma2, Pca21, Pnc2, Pmn21, Pba2, Pna21, Pnn2, Cmm2, Cmc21, Ccc2, Amm2, Abm2, Ama2, Aba2, Fmm2, Fdd2, Imm2, Iba2, Ima2 |
Tetragonal |
4 |
4mm
|
P4, P41, P42, P43, I4, I41 |
P4mm, P4bm, P4
2
cm, P4
2
nm, P4cc, P4nc, P4
2
mc, P4
2
bc, I4mm, I4cm, I4
1
md, I4
1
cd
|
Trigonal |
3 |
3m
|
P3, P31, P32, R3, |
P3m1, P31m, P31c, P3c1, R3m, R3c
|
Hexagonal |
6 |
6mm
|
P6, P61, P65, P62, P64, P63 |
P6mm, P6cc, P6
3
cm, P6
3
mc
|
The most important functionalities that make ferroelectrics so useful are the high values of the real part of complex dielectric permittivity, ε*, spontaneous polarization, Ps, good mechanical and thermal resistance and small dielectric losses.18,19 This set of traits determines the transition from the paraelectric phase (which is usually high-temperature, high symmetry state) to the ferroelectric one (low-temperature, low-symmetry state) in the close vicinity of the Curie temperature (Tc). The paraelectric–ferroelectric PT is related to the symmetry change (e.g. some symmetry elements of the paraelectric phase are lost, when the ferroelectric phase is reached), and leads to the structurally modified (ordered) ferroelectric phase. This structural transformation (described often as symmetry-breaking), is reflected in the macroscopic properties of the material, e.g. the appearance of Ps in the ferroelectric phases.
One of the classes of molecular–ionic materials are organic–inorganic hybrid ferroelectrics which are currently of particular interest, due to the designable and modifiable characteristics of their organic and inorganic components, for exploring ferroelectric-based multifunctional materials such as multiferroics, semiconductors and photovoltaic materials.20–23 Of note, the symmetry, size, and value of the dipole moment and finally, the reorientations of the organic cations play an important role in triggering the structural PT. Therefore, control of the ‘order–disorder’ motion of the organic counterions in a molecular crystal is a key issue for the design of hybrid molecular–ionic ferroelectrics.
Haloantimonates(III) and halobismuthates(III), which are the subject of the present review, defined by the general formula RaMbX3b+a, (where R – organic cations, M = Sb(III) or Bi(III) and X = Cl, Br, I) create one of the well-recognizable groups of ferroic compounds, essential mainly from an application point of view as they combine many desirable features, e.g. facile synthesis and processing, and cost-effectiveness with promising electrical and optical properties.24–29 A characteristic feature of the compounds under consideration is that they possess the lone electron pair (5s2 (Sb) and 6s2 (Bi)). However, its presence, which has a moderate impact on the structural properties of these salts, seems to have a tremendous effect on the physical properties of crystals. The lone electron pair deforms very easily a spherical symmetry of the orbital, on which they are located. This leads to an increase in the length of the M–X bonds, and thus to a considerable deformation of the MX6 octahedra.
The polyanionic structure of the lattice is determined by the size of the organic cation, its symmetry and ability to create hydrogen bonds of the N–H⋯X type; the size of the halogen atom, which jointly creates the lattice is also of crucial importance. The alkylammonium cations belong to a wide variety of organic cations, which crystallize with various anionic sublayers. They are characterized by the different chain lengths, from methyl- to butyl-ammonium, and they may form five- or six-membered rings in the aromatic cations (e.g. imidazolium, pyridinium and its substituted derivatives) as well as saturated heterocyclic amines (e.g. pyrrolidine) (Fig. 1).
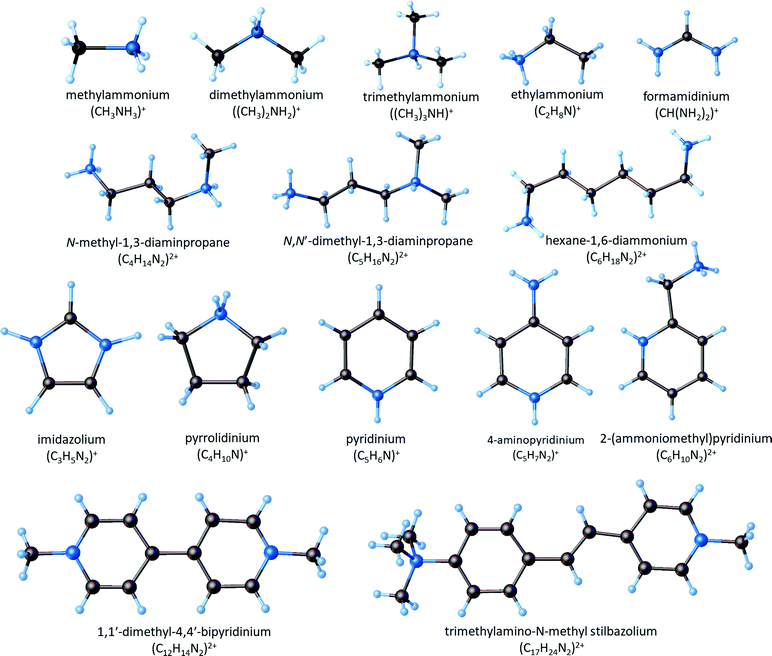 |
| Fig. 1 Molecular structure of the organic cations creating ferroelectric compounds among halobismuthates(III) and haloantimonates(III). | |
An analysis of these compounds has revealed a richness of the cationic unit movements. The motions depend on three basic factors such as: (i) the symmetry of the cation; (ii) the value of the dipole moments of the cation; (iii) the structure of the anionic network surrounding the cation. The reorientation ability of the dipolar units occupying vacancies inside the anionic sublayers is determined mainly by the symmetry and size of these spatial vacancies (a reorientational disorder), while the dynamic properties of the anionic networks are limited primarily by the formation of polymeric structures. In the organic part, the dynamic properties change significantly when the temperature is being lowered since the cation mobility decreases. This process is accompanied by a significant distortion of the anionic lattice. It must be mentioned that one of the most significant features of this lattice is its flexibility and, at the same time, its facility of adaptability of symmetric cations, which is related to the effect of the electron lone pair on the Sb(III) and Bi(III) atoms. The presence of hydrogen bonds of the N–H⋯X-type in the crystal structure is significant for the lattice formation. The strength of these bonds increases proportionally to the size of atom radius of the corresponding halogen. A rearrangement of these bonds takes place during the PT, which significantly affects the dynamic properties as well as the ordering of the cations.
In the present paper, we would like to focus on the ferroelectric structures among halogenoantimonates(III) and halogenobismuthates(III), which can be found in the literature and on the correlation between their crystal structures and polar properties. For this reason, the structural analysis of the compounds with the organic cations, a description of the cationic dynamics and the deformation of the inorganic layers have been undertaken. Moreover, macroscopic dielectric properties are discussed in detail.
2. Structural diversity of the anionic networks of haloantimonates(III) and halobismuthates(III)
Haloantimonates(III) and halobismuthates(III) are characterized by a significant diversity of the anionic networks (from zero-, through one-, two-, and three-dimensional architectures).30–44 Taking into account the molar ratio of the amine (R) and metal (M) (Sb(III)/Bi(III)) the following four basic stoichiometries are observed.
2.1. RMX4 (R
:
M = 1
:
1)
The anionic sublattices observed in this group may be divided into two types: (i) polyanionic chains; (ii) isolated units.
As for type (i) three different types of polyanionic chain arrangements are observed:
• chains of the edge-sharing octahedra (with two terminal and four bridging halogen atoms) (Fig. 2(a))45–54
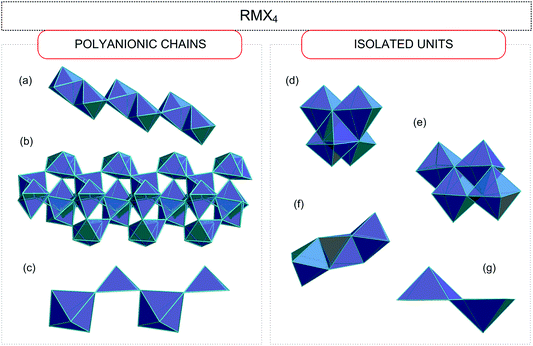 |
| Fig. 2 Structures of anionic lattices with [MX4]− stoichiometry: (a) infinite chain with edge-sharing octahedra; (b) infinite tape of differently joined octahedra; (c) infinite chain of square pyramids; (d) four octahedra possessing three bridging and three terminal halogens; (e) two octahedra with three terminal and three bridging halogens and two others with two terminal and four bridging halogens; (f) three face-sharing octahedra; (g) two edge-sharing pyramids. | |
• the [MX6]3− octahedra linked as follows: three non-equivalent M atoms possess three bridging and three terminal contacts, whereas the remaining one possesses only one terminal and five bridging contacts (Fig. 2(b));55
• chains of the corner-sharing square pyramids (Fig. 2(c));56
With regard to the separated units (ii) four different assemblies may be recognized.
Four octahedra linked with each other in two different ways:
• one type of central atom with three bridging and three terminal halogens (Fig. 2(d))57
• two central atoms surrounded by three terminal and three bridging halogens and two others coordinated by two terminal and four bridging halogens (Fig. 2(e));58–60
• face-sharing octahedra (Fig. 2(f));61,62
• edge-sharing two square pyramids (Fig. 2(g));63–70
2.2. R3M2X9 (R
:
M = 1.5
:
1)
•. infinite, one-dimensional (1D) zig-zag double chains (pleated ribbon structures) (Fig. 3(a));71–76
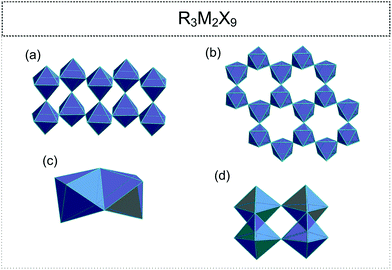 |
| Fig. 3 Anionic structure of the [M2X9]3− composition; (a) one-dimensional double chain; (b) two-dimensional corrugated sheet; (c) two face-sharing octahedra; (d) four edge and corner-sharing octahedra. | |
•. two-dimensional (2D) layers (honey-comb like corrugated sheets) (Fig. 3(b));72,77–89
•. discrete bioctahedra (0D) (Fig. 3(c));45,90–94
•. four octahedral units (0D) ([M4X18]6−) (Fig. 3(d)).95–99
2.3. R2MX5 (R
:
M = 2
:
1)
• one-dimensional (1D) chains (cis- or trans-type) (Fig. 4(a) and (b) respectively)100–109
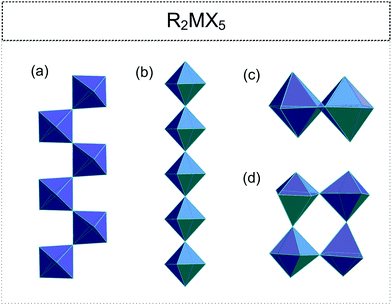 |
| Fig. 4 Anionic structure of the [MX5]2− composition; (a and b) the polyanionic chains with corner-sharing octahedra ((a) cis- (b) trans-configuration); (c) two edge-sharing octahedra; (d) a squaric structure containing four corner-sharing octahedra. | |
• isolated [M2X10]4− units (two edge-sharing octahedra) (Fig. 4(c));49,110,111
• tetrameric polyanion with corner-sharing octahedra (Fig. 4(d)).55,112
2.4. R5M2X11 (R
:
M = 2.5
:
1)
• Corner-sharing octahedra (0D) ([M2X11]5−) (Fig. 5(a)).113–123
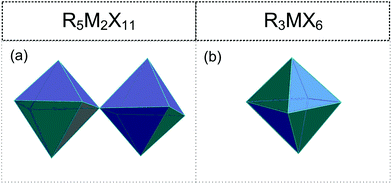 |
| Fig. 5 (a) Structure of the isolated [M2X11]5− anions; (b) isolated [MX6]3− octahedra. | |
2.5. R3MX6 (R
:
M = 3
:
1)
• Isolated [MX6]3− octahedra (Fig. 5(b)).124–133,134,135
2.6. Different modifications of the anionic substructures
The richness of the anionic structures among halobismuthates(III) and haloantimonates(III) is related also to the more exotic type of stoichiometry34,136 (see Fig. 6), e.g. RM2X7,137,138 RM3X10,139–141 R3M5X18,99,142,143 R4M6X22,142–144 R4M8X28138,139 or R6M8X30.145
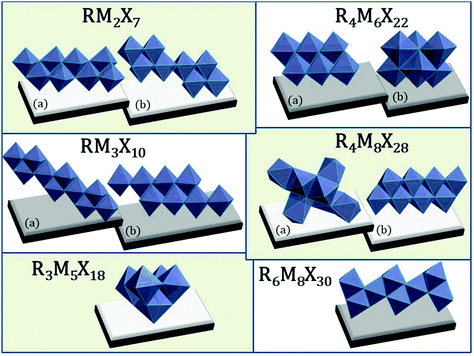 |
| Fig. 6 Atypical anionic structures among haloantimonates(III) and halobismuthates(III). ((a and b) labels correspond to the different organization of the inorganic structures) RM2X7 polyanionic 1D chains; R4M6X22 0D units; RM3X10 infinite (1D) polyoctahedral chains; R4M8X28, R3M5X18, R6M8X30 isolated (0D) units.137–145 | |
3. Ferroelectricity among haloantimonates(III) and halobismuthates(III)
The ferroelectricity among halobismuthates(III) and haloantimonates(III) is limited only to four different types of chemical compositions: RMX4, R3M2X9, R2MX5 and R5M2X11. Below, the analysis of the physical properties is presented and a correlation between the crystal structure and the origin of their polarity (ferroelectricity) is discussed. In our review we focus on pure organic–inorganic hybrids based on Bi(III) and Sb(III) (mixed anionic networks were not analyzed); however, due to the discovery of the first ferroelectric among halobismuthates(III) characterized by the mixed organic networks (R′R2′′MX6) a single exception has been made.
3.1. RMX4 type compounds
Ferroelectricity in the RMX4 type compounds is a unique feature. Based on the literature, only two examples of ferroelectrics, characterized by the 1D polymeric anionic structure, are known: (4-aminopyridinium)[SbCl4]51–53 ((4-NH2C5H4NH)[SbCl4], 4-APCA) and (trimethylamino-N-methyl stilbazolium)[Bi2Cl8]54 ((C17H24N2)[Bi2Cl8], (TAMS)[Bi2Cl8]). The detailed X-ray structure analysis indicates that in both compounds, the ferroelectricity is related mainly to a strong deformation of the [MX4]−∞ type of substructure (see Fig. 2(a) and Table 2).
Table 2 Phase diagram of RMX4 stoichiometry
|
Ref. |
1st-first order PT, 2nd-second order PT ferroelectric phase paraelectric phase |
|
51–53
|
|
54
|
The crystal structure of 4-APCA is built up of the polyanionic chains of [SbCl4−]∞ forming a tunnel-like structure and disordered 4-aminopyridinium cations connected via weak hydrogen bonds. Phase situation is complex and characterized by rich polymorphism in the solid state (see Scheme 1). The ac calorimetry studies have shown that the high temperature PT at 304 K is of the ‘order – disorder’ type and leads to a incommensurate modulated phase (II). At 270.5 K (II → III) a ‘lock-in’ transition to a commensurate modulated phase is observed, which, in turn, becomes a ferroelectric one. The anomaly at 248 K leads to the next polar, but modulated incommensurate phase, while below 240 K the crystal becomes nonpolar again. The ferroelectric transformation at 270.5 K is characterized by a ‘displacive’ type of mechanism related to the displacement of the organic cations in relation to the anionic chains. Dielectric dispersion measurements carried out in the radio-frequency region around Tc (270.5 K) revealed a critical slowing-down assigned mainly to the domain-like wall motion of the incommensurate phase (Fig. 7(a)). The relaxation process was found to be nearly monodispersive, which is rare among incommensurate ferroelectrics.146 The polar properties of 4-APCA were confirmed by the switchable Ps of about 0.35 μC cm−2 at 265 K. One should note that among halogenobismuthates(III) and halogenoantimonates(III), 4-APCA was the first example of a ferroelectric compound characterized by the molecular mechanism of a ‘displacive’-type assigned to the ferroelectric PT.
 |
| Scheme 1 Phase diagram of 4-APCA.51–53 | |
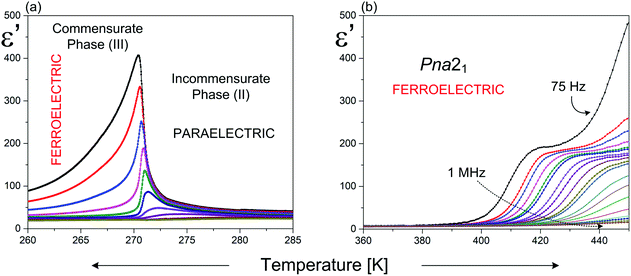 |
| Fig. 7 Temperature dependence of the real part of the complex dielectric permittivity for: (a) 4-APCA;51 (b) (TAMS)[Bi2Cl8].54 | |
With regard to (TAMS)[Bi2Cl8], it belongs to room temperature (RT) ferroelectrics, but the Curie temperature was not achieved on heating due to the earlier decomposition of the compound. (TAMS)[Bi2Cl8] crystallizes in an acentric orthorhombic space group (Pna21) and consists of a framework of infinitive [Bi2Cl8]2−∞ inorganic chains, separated by [TAMS]2+ cations. The non-centrosymmetric crystal structure and polar alignment of the organic species contribute to the Ps value, which is of the order of 0.08 μC cm−2 at 453 K.
The dielectric measurements confirm the lack of PT in a wide temperature range and indicate the existence of the dielectric relation process (Fig. 7(b)). The expected effect of the slowing down of the macroscopic relaxation time, related to the dynamics of the ferroelectric domains, is not observed when approaching the para-ferroelectric transition, which might be connected to the huge contribution of the electric conductivity to the dielectric response. It should be noted that the estimated relaxation time for the relaxation process in (TAMS)[Bi2Cl8] becomes shorter on heating, which is a quite opposite effect in comparison with that observed in the other ferroelectrics. This is characteristic of the systems with dipolar group reorientations without any long-range dipole–dipole interactions. The activation energy of the relaxation process calculated from the Arrhenius relationship, Ea, equals to 280 kJ mol−1 and may be a result of some dipole cooperative motions or steric hindrance originating from the huge organic molecule as for the single dipole motion.54
3.2. R3M2X9 type compounds
Within the chemical stoichiometry R3M2X9 the ferroelectricity is limited to two types of anionic sublayers among four possible ones (Fig. 3); 2D (two-dimensional layers) (Fig. 3(b)) and 0D-discrete bioctahedral units (Fig. 3(c)). The 2D structure is usually characteristic of the chloride and bromide ferroelectrics containing only small alkylammonium moieties (methyl-, dimethyl and trimethyl-ammonium) or non-substituted rings (e.g. pyrrolidinium cation) (Table 3). The bulky organic cations due to the steric effects cannot occupy small vacancies within the layers. On the other hand all known iodide ferroelectrics possess the 0D anionic network (Table 4) thus the restriction with respect to the size and symmetry of the organic moieties is not so obvious.
Table 3 Phase diagram of R3M2X9 with a 2D anionic architecture
Table 4 Phase diagram of R3M2X9 with a 0D anionic architecture
The characteristic feature of the paraelectric phase in 2D R3M2X9 ferroelectrics is the presence of two crystallographically independent alkylammonium cations in the crystal structure (N(1) and N(2)). The cation N(1) is located in voids inside the polyanionic layers, whereas the N(2) one between the layers (Fig. 8). All cations are connected to the anionic sublattice by N–H⋯X hydrogen bonds. Both types of cations are highly disordered in the paraelectric phase.
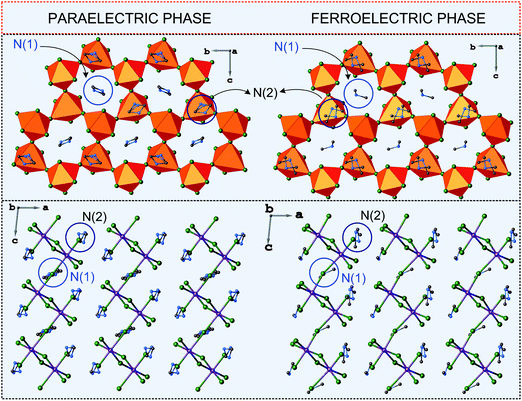 |
| Fig. 8 Comparison of the crystal structures of DMACA in the paraelectric and ferroelectric phases (ref.88). | |
The disorder of N(1) is usually described by a two-site model with an occupancy factor of the N(1) atom, equal to 0.5. The other cation N(2) is also split between two positions, N(21) and N(22), however, with different occupancy factors, which change with temperature. Since the N(2) cation is in the general position of the crystal structure, this change does not lead to any changes in the lattice symmetry in contrast to the N(1) cation dynamics. The paraelectric–ferroelectric transitions lead to the distortion of the anionic network, which results in the loss of the symmetry center. Thus the N(1) cations now occupy a single position in a ferroelectric domain and their long-range order is responsible for an appearance of Ps. In the case of the 2D ferroelectrics the polar direction is maintained within the corrugated layer structure. This means that the layers enhanced the polarizability of the system, which is coupled with the dynamics of the N(1) type cations.
An enhanced value of the electric permittivity close to Tc is observed for the 2D ferroelectrics, because easily polarizable anionic layers favor the long-range order of the dipole–dipole interactions (Fig. 9).
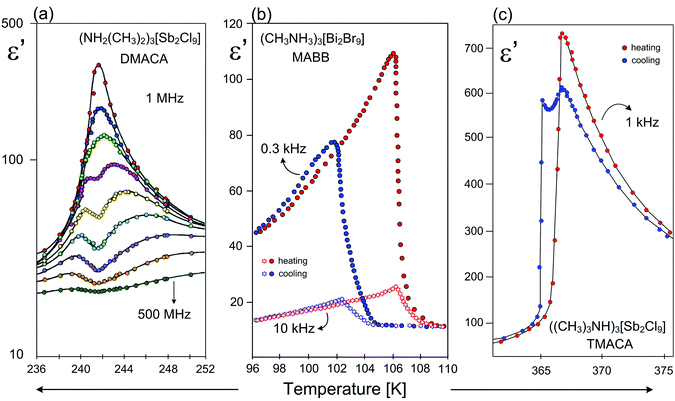 |
| Fig. 9 Temperature dependence of the real part of the dielectric permittivity (ε′) for (a) ((CH3)2NH2)3[Sb2Cl9] (DMACA)131 (b) (CH3NH3)3[Bi2Br9] (MABB);84,85 (c) ((CH3)3NH)3[Sb2Cl9] (TMACA);131 around Tc. | |
In spite of the structural similarities of the 2D type ferroelectrics their dielectric responses, character of PTs and phase situations are quite diverse. The dielectric response of the majority of the 2D analogs shows a critical slowing down of the macroscopic relaxation time, which indicates an ‘order–disorder’ mechanism of ferroelectric transition (Fig. 9(a)). In turn in the case of MABB the dielectric response (ε(T)) is characteristic of ‘improper’ ferroelectrics (1st – order PT) (Fig. 9(b)), while for the TMACA the dielectric results indicate the existence of a narrow-range intermediate metastable phase (incommensurate) between the ferroelectric and paraelectric phases (Fig. 9(c)).
The only parameter that correlates well with the temperature scope of the ferroelectric phases is the cation size (Tables 3 and 4). It is clearly seen that an increase of the cations size leads to the shift of the Curie point towards high temperatures. The large cations usually have less freedom of movement in the anion layer (N(1)-type) gaps, which is why they are easier to freeze at higher temperatures in cooling cycles in comparison with the ferroelectrics with the small cations.
Haloantimonates(III) and halobismuthates(III), characterized by the 2D anionic structure, usually possess a smaller value of Ps in contrast to that for the 0D analogs, in spite of the fact that the organic cations of the presented crystals have a larger value of dipole moments, μ. Nevertheless it should be noted that in the formamidinium analogs the cations possess a quite small value of μ = 0.23 D and the dielectric anomalies are rather intermediate. It seems that the relationship between structural parameters of the crystals and the macroscopic properties (Ps and εmax) is rather complex and its recognition requires further studies on R3M2X9-type compounds. It is interesting that most 0D ferroelectrics possess polar properties above room temperature, except for (NH2CHNH2)3[Bi2I9]151 and (NH2CHNH2)3[Sb2I9].152 The paraelectric phase exhibits usually high symmetry (trigonal or hexagonal), which proves a high dynamic disorder of the cationic networks. Generally, the mechanism of ferroelectric phase transitions for the 0D compounds is quite complex because the ‘order–disorder’ and ‘displacive’ contributions are possible.
3.3. R2MX5 type compounds
A Cambridge Structural Database survey (version 5.40 March 2019) permits us to predict the probability of the appearance of acentric/ferroelectric compounds within R2MX5-type stoichiometry. 192 entries, adopting either polymeric (1D) or isolated (0D) anionic substructures, have been found.
The results presented in Tables 5 and 6 confirm that the ferroelectricity in the R2MX5-type compounds is adopted in the 1D anionic network. The ferroelectricity does not depend on the symmetry and size of the organic cations (heteroaromatic, heterocyclic aliphatic) but is strongly affected by the dipole moment values.
Table 5 Phase diagram of R2MX5 or RMX5 stoichiometry
Table 6 The results of CSD analysis
Anionic substructure |
All compounds |
Piezoelectric |
Ferroelectric |
Isolated pyramids [MX5]2− |
35 |
8 |
0 |
Isolated bioctahedra [M4X10]4− |
81 |
2 |
0 |
Isolated tetrameric forms [M4X20]8− |
4 |
1 |
0 |
Infinite chains [MX5]∞ |
72 |
30 |
9 |
Summary
|
192
|
41
|
9
|
A characteristic feature of this subclass is the construction of the anionic lattice, which is created by 1D infinite chains of different configurations (cis- or trans-mode), however, the cis-conformation (and its modifications) is very common in the analyzed subgroup. Generally, one can state that the distortion of the anionic chains through the PT is a driving force, which contributes mainly to the Ps value. This effect is coupled with the dynamics of the polar organic cations, which are usually disordered in the paraelectric phase to varying degrees. Distortion of the anionic substructure is treated as a ‘displacive’ contribution to the molecular mechanism of the ferroelectric transition, whereas a change in the dynamics of the organic moieties is an ‘order–disorder’ contribution (Fig. 10).
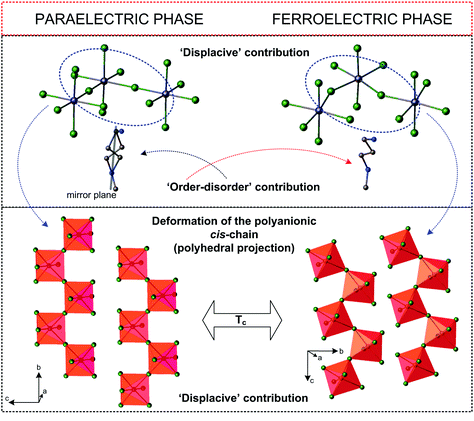 |
| Fig. 10 Comparison of the crystal structures of (NH2(CH2)3NHCH3)[BiCl5]155 in the paraelectric and ferroelectric phases. | |
The molecular mechanism of the paraelectric-ferroelectric transformation varies from ‘displacive’ through mixed up to purely ‘order–disorder’, however, which of these mechanisms is dominant depends strongly on the subtle differences in the development of the polyanionic chains. In the case of the ‘displacive’ R2MX5 subclass we should take into account the ns2 electron lone pair effect (5s2-Sb and 6s2-Bi), which seems to play a key role in the distortion of the MX6 octahedron. The derivatives, characterized by trans-connected octahedra containing bulky organic methylviologen MV+ amine: (MV)[BiBr5],157 (MV)[BiI3Cl2], are an example of such a distorted structure.107 The MV2+ cations have stabilized unprecedented regular [BiBr52−]∞ chains of trans-connected octahedra.
Through PT, the trans Bi–Br bonds differentiate markedly and the result is the chain polarity. Moreover, the electronic lone pair is stereochemically activated below 243 K leading to an acentric polar phase. The mixed-halide analog, (MV)[BiI3Cl2], reaches one of the highest room-temperature Ps values (>15 μC cm−2 at 298 K) in the field of organic–inorganic hybrid ferroelectrics. It should be added that trans-connected isomers are much rarer than cis-connected ones. It is difficult to indicate a different correlation between the crystal structure and dynamics and the scope of polar phases in this group of compounds.
Compared to R3M2X9 connections, the organic cations in R2MX5 show less freedom of movement in the paraelectric phases (usually a two-site model) thus the compounds adopt lower symmetry (mainly orthorhombic). Thus most of the paraelectric-ferroelectric transitions in the R2MX5-subclass is described by the mmmFmm2 Aizu relationship (non ferroelastic transitions), in turn methylviologen analogs characterized by trans-connected-modes of the anionic chains exhibit also non-ferroelastic transition between monoclinic phases described by 2/mFm or 2/mF2 species (Fig. 11).
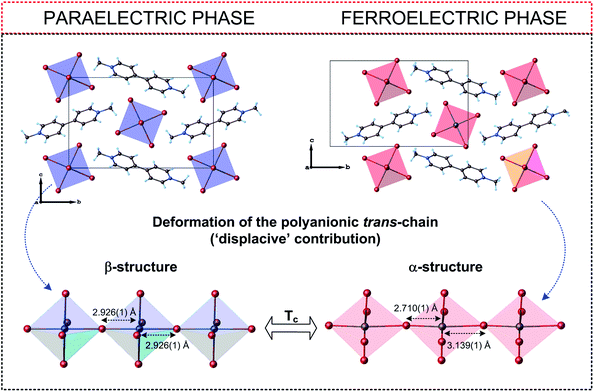 |
| Fig. 11 Comparison of the crystal structures of (C12H14N2)[BiBr5]157 in the paraelectric and ferroelectric phases. | |
As it is presented (Table 5) for the hybrids based on very similar organic cations (N-methyl-1,3-diaminopropane) and (N,N-dimethyl-1,3-diaminopropane) the differences in the Tc values (existence of polar phases) reach about 230 K (376 and 143 K, respectively), however, the values of Ps are comparable (2.38 and 1.36 μC cm−2, respectively). In turn, the organic–inorganic compounds based on the ethylammonium cation indicate similar values of Tc (190 and 160 K), while Ps differs almost by three orders 1.4 and 5 × 10−3 μC cm−2. In conclusion, within R2MX5-type ferroelectrics the amount of the deformation of the anionic chains is crucial, when it comes to the value of Ps and a character of the dielectric response close to Tc (Fig. 12).
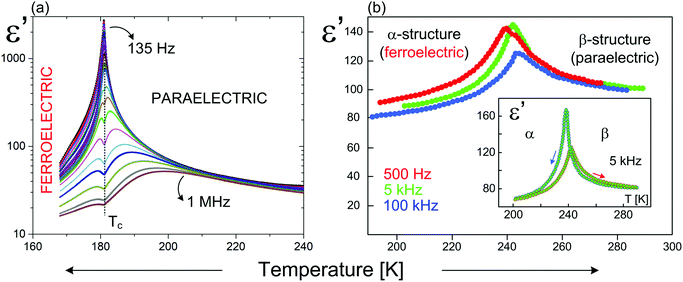 |
| Fig. 12 Temperature dependence of the real part of the complex dielectric permittivity for: (a) (C3H5N2)2[SbCl5];158 (b) (C12H14N2)[BiBr5].157 | |
3.4. R5M2X11 type compounds
Complexes of R5M2X11 stoichiometry are unique in crystalochemistry of haloantimonates(III) and halobismuthates(III). Currently, scientific literature gives only seven examples of the compounds marked by this type of chemical composition and all of them exhibit ferroelectric properties (see Table 7). A typical trait of the compounds in question is the presence of discrete bioctahedral units, [M2X11]5− in their crystal structure, in which two octahedra are connected with each other by one bridging halogen atom (see Fig. 13).
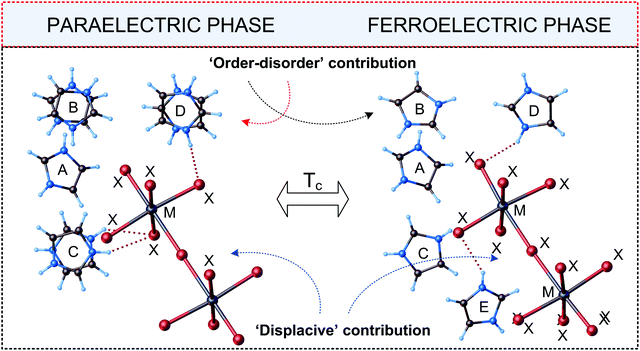 |
| Fig. 13 Independent parts of the unit cell of [C3N2H5]5[Bi2Br11] in the paraelectric and ferroelectric phases (M = Bi(III)/Sb(III), X = Cl, Br, I).113,114 | |
Table 7 Phase diagram of R5M2X11
A cationic subnetwork consists in turn of four kinds of nonequivalent types of organic species marked by varied dynamic disorder. Phase situation for all R5M2X11-ferroelectrics is presented in Table 7.
A detailed analysis of the phase diagrams and structural data shows that the size of the organic cation and its shape and symmetry, have a significant influence on PTs’ sequence, on the symmetry of each phase, on the polar properties of compounds as well as on the temperature range of the existence of the polar (ferroelectric) phases. Thus, this group may be divided into two subgroups: the first one created by the hybrids based on the aliphatic cations, e.g.: (CH3NH3)5[Bi2Br11],116–118 (CH3NH3)5[Bi2Cl11]119–122 and (NH2CHNH2)5[Sb2Br11]163 (abbreviated as aliphatic analogs) and the second one formed by aromatic counterions: (C3H5N2)5[Bi2Cl11],113 (C3H5N2)5[Bi2Br11],114 (C3H5N2)5[Sb2Br11]123 (R = imidazolium) and (C5H5NH2)5[Bi2Br11]115 (R = pyridinium) denoted as an aromatic subgroup.
Consequently, an analysis of phase symmetry indicates that the symmetry of the paraelectric phases of the aliphatic analogs is higher (orthorhombic symmetry) than that of the three remaining salts containing aromatic organic cations (monoclinic). The temperature range of the existence of ferroelectric phases in the aliphatic compounds stays within room temperature (except of the formamidinium analog), while in the aromatic compounds it is shifted by almost 150–180 K towards the low temperatures. Despite obvious structural differences between these two subgroups (aliphatic/aromatic), the following elements are common for the compounds of the R5M2X11 type:
• PTs are continuous in nature;
• PTs are classified as an ‘order–disorder’ type;
• Close to Tc, a critical slowing down of the macroscopic relaxation time is observed;
• Molecular mechanism of the ferroelectric PT is dominated by the dynamics of the organic cations.
In the paraelectric phase, two out of five cations, being in a general position, are ordered, while the remaining three, (located at special positions) reveal an orientational disorder (two-site model, 180° reorientation). In the ferroelectric phase, all cations are ordered, however, the key input to Ps is given only by three out of five organic cations.
Another element that distinguishes the analyzed materials is the long-range dipole–dipole interaction. It determines both the values of Ps and the electric permittivity close to Tc. Methylammonium ferroelectrics mark themselves out by the higher values of Ps in the range of 2 – 3 μC cm−2 and εmax 1 – 2 ×104, than those for the aromatic analogs. The value of Ps for the latter compounds is by almost one order, and for ε′ almost by 1.5 order, lower than that for the former ones. This means that the long-range dipole–dipole interactions in the aliphatic ferroelectrics are much stronger than those in the aromatic ones. This in turn has an impact on the values of the PT temperatures. The stronger the dipole–dipole interaction the higher the values of Tc (Fig. 14).
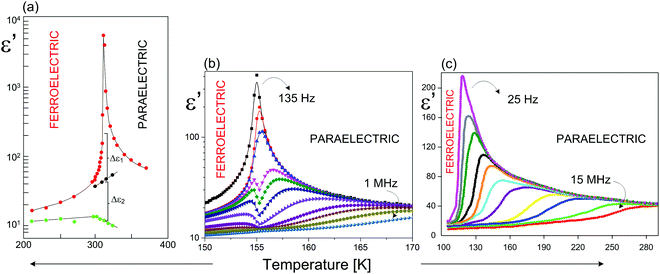 |
| Fig. 14 Temperature dependence of the real part of the dielectric permittivity (ε′) for (a) (CH3NH3)5[Bi2Br11]116–118 (MAPBB); (b) (C3H5N2)5[Bi2Br11];114 (c) (C5H5NH)5[Bi2Br11]115 around Tc. | |
The other property, which deserves to be compared, is the dynamic property of ferroelectrics, analyzed using the dielectric relaxation data. All R5M2X11 ferroelectrics indicate the critical slowing down of the macroscopic relaxation time around Tc, a phenomenon typical only of the ferroelectrics with an ‘order–disorder’ mechanism of PT. What matters for these crystals is a difference in the values of the macroscopic dielectric relaxation time. The dielectric dispersion in a case of the aromatic analogs is limited to the kilohertz frequencies (102–104 Hz), while in the aliphatic ones it is shifted to the microwave range (10–8–10–10 Hz). One of the reasons for such a difference may be the size of the inertia moments of the organic dipoles as well as the way, in which the cations are bound through the hydrogen bound networks. The imidazolium cations have much higher inertia moments than methylammonium and formamidinium.
The most mysterious phenomenon in the R5M2X11 ferroelectric group is the molecular mechanism of PT. In this regard, two possible contributions must be taken into account:
(i) ‘order–disorder’
(ii) ‘displacive’
The first contribution (i) is related to the dynamics of the organic cations, i.e. orientational disorder. The typical feature of the ferroelectrics with a continuous PT is the critical slowing down of the macroscopic relaxation time and a significant entropy effect (ΔS > R
ln 2) indicating the mechanism of type (i).
The other contribution, of the ‘displacive’ type (ii), is not proved by experimental methods; however, based on the structural data, there are reasons to believe that the distortion of the discrete bioctahedral units is likely to appear and, therefore, should be taken into account. This effect is well visible among the aliphatic ferroelectrics but it is marginal in aromatic ones. The distortion of the bioctahedra units causes a change in the negative charge distribution in the crystal structures, related to the halogen atoms; this subsequently leads to a change of the Ps values. There is one more factor necessary to analyze, namely, the electron lone pair effect. This effect should be attributed to the presence of the 6s2 electrons of Bi(III) atom, which is less polarizable than 5s2 electrons of Sb(III) atoms. All that leads to the conclusion that the anionic sublattice plays an important role in the generation of ferroelectricity in the R5M2X11-type hybrids. Flexibility of the bioctahedral units (corner-sharing octahedra) may be a decisive parameter in the origin of spontaneous polarization.
3.5. R3MX6 type compounds and their modification (e.g. R′R′′2 MX6)
Recently a first halobismuthate(III) compound characterized by mixed organic networks has been reported by Wang et al.161 The cationic substructure consists of two different molecules: one methylammonium (R′) and two benzylammonium (R′′) ones as well as by an isolated octahedral unit [BiBr6]3−. [(CH3)2NH2][C6H5CH2NH2]2BiBr6 appears to be a RT multiaxial ferroelectric with Ps = 1.0 μC cm−2. The symmetry-breaking paraelectric–ferroelectric transformation is regarded in the Aizu notation as
mFm at 386 K. The molecular mechanism of the ferroelectric PT is assigned to the change in dynamics of the organic cations thus the transition is classified as an ‘order–disorder’ type. The discovery of a new type of connection of halobismuthates(III) with a mixed anionic substructure creates a new path to design molecular multiaxial ferroelectrics.
Although R3MX6-type compounds seem to be the ‘poorest’ with regard to their ferroelectricity, an interesting phenomenon, related to the imidazolium based hybrid, has been detected. The most spectacular result was the preparation of a new crystalline ferroelectric material ((C3H5N2)5[Sb2Br11]) using an in situ solid-state chemical reaction, where the starting substrate was (C3H5N2)3[SbBr6]·H2O.162
4. Conclusions
In this review we present the attempts to find a correlation between crystal structures and polar/ferroelectric properties of haloantimonates(III) and halobismuthates(III). The materials under consideration are characterized by a rich diversity of the anionic sublattice (more than 40 types), nevertheless, the ferroelectric properties are limited only to four specific compositions: RMX4, R3M2X9, R2MX5, R5M2X11. Of importance is that within the mentioned stoichiometry, the anionic network dimensions are also changed. One should note that so far already 31 ferroelectrics have been synthesized and fully characterized from the above mentioned family, which put them on one line with a well-known family of perovskite-type ferroelectric crystals (ABX3, A2BX4).164–167
The RMX4-type ferroelectrics (2 examples) indicate a 1D anionic structure, while the PTs are classified as a ‘displacive’ one. In the case of R3M2X9 hybrids the ferroelectric ordering is observed only in the 0D (face-sharing bioctahedral species) and 2D inorganic lattice (corrugated layer structure and small organic cations). The molecular mechanism of the paraelectric–ferroelectric PTs for the 2D ferroelectrics is of the ‘order–disorder’ type. In turn for the 0D compounds the mechanism is quite complex because the ‘order–disorder’ and ‘displacive’ contributions are possible. Moreover, in the case of the R3M2X9 complexes of the 2D-type the ferroelastic structure in both paraelectric and ferroelectric phases is observed (possible biferroics), however, the coupling between the corresponding order parameters, polarization and deformation, is very weak.
With regard to R2MX5 complexes, the ferroelectricity is conditioned by the presence of the 1D anionic structures with different modifications and the majority of PTs is of the ‘displacive’-type with a minor ‘order–disorder’ contribution.
The R5M2X11 hybrids are the most attractive from the viewpoint of polar properties. In this subclass, the inorganic part is created by the discrete anionic structures of the corner-sharing octahedra (0D-type). All of the R5M2X11 crystals reveal ferroelectric properties. The molecular mechanism of the paraelectric–ferroelectric PT is not well recognized yet, however, the ‘order–disorder’ contribution, related to the dynamics of the organic cations, seems to play a key role. On the other hand the strong distortion of the anionic units as a result of the electron lone-pair effect (5s2 (Sb) and 6s2 (Bi)) influences additionally the total polarization of the material. Of great importance is that aliphatic analogs, e.g. (CH3NH3)5[Bi2Cl11], (CH3NH3)5[Bi2Br11] and (NH2CHNH2)5[Sb2Br11] indicate dielectric properties similar to the well-known TGS (triglicyne sulphate) family and thus make them the most promising materials for applications.
It appears that not all stoichiometries have been analysed in terms of possible ferroelectric properties and R′R′′BiBr6 is the best example. It should be also added that the complexes of iodobismuthates(III), both with the organic cations (e.g. methylammonium) and with the monovalent metals (mixed cationic sublattice), are the most advantageous in the case of absorbing materials in solar cells. We anticipate that the broadening group of molecular–ionic ferroelectrics will help to develop rational structure–property relationships, leading to materials with precisely tunable characteristics.
Conflicts of interest
There are no conflicts to declare.
Acknowledgements
The paper is a result of the realization of the project no. UMO-2016/21/B/ST3/004640201/2078/17 financed by the National Science Centre, Poland (Prof. G. Bator).
References
- G. Rijnders, S. Currás, M. Huijben, D. H. A. Blank and H. Rogalla, Influence of Substrate-Film Interface Engineering on the Superconducting Properties of YBa2Cu3O7−δ, Appl. Phys. Lett., 2004, 84, 1150 CrossRef CAS
.
- J. F. Scott, Applications of Modern Ferroelectrics, Science, 2007, 315, 954 CrossRef CAS PubMed
.
- P. Hou, K. Yang, K. Ni, J. Wang, X. Zhong, M. Liao and S. Zheng, An Ultrathin Flexible Electronic Device Based on the Tunneling Effect: A Flexible Ferroelectric Tunnel Junction, J. Mater. Chem. C, 2018, 6, 5193 RSC
.
- H. Gu, X. Qian, X. Li, B. Craven, W. Zhu, A. Cheng, S. C. Yao and Q. M. Zhang, Chip Scale Electrocaloric Effect Based Cooling Device, Appl. Phys. Lett., 2013, 102, 122904–122901 CrossRef
.
- Y. D. Wang, S. J. Smullin, M. J. Sheridan, Q. Wang, C. Eldershaw and D. E. Schwartz, A Heat-Switch-Based Electrocaloric Cooler, Appl. Phys. Lett., 2015, 107, 134103–134101 CrossRef
.
- J. F. Scott, F. D. Morrison, M. Miyake and P. Zubko, Nano-Ferroelectric Materials and Devices, Ferroelectrics, 2006, 336, 237 CrossRef CAS
.
- K. Gao, M. Gu, X. Qiu, X. N. Ying, H. Y. Ye, Y. Zhang, J. Sun, X. Meng, F. M. Zhang, D. Wu, H. L. Cai and X. S. Wu, Above-Room-Temperature Molecular Ferroelectric and Fast Switchable Dielectric of Diisopropylammonium Perchlorate, J. Mater. Chem. C, 2014, 2, 9957 RSC
.
- M. Dawber, K. M. Rabe and J. F. Scott, Physics of Thin-Film Ferroelectric Oxides, Rev. Mod. Phys., 2005, 77, 1083 CrossRef CAS
.
- G. Lupina, J. Dąbrowski, P. Dudek, G. Kozłowski, M. Lukosius, C. Wenger and H.-J. Müssig, Perovskite BaHfO3 Dielectric Layers for Dynamic Random Access Memory Storage Capacitor Applications, Adv. Eng. Mater., 2009, 11, 259 CrossRef CAS
.
- S. K. Kim, S. W. Lee, J. H. Han, B. Lee, S. Han and C. S. Hwang, Capacitors with an Equivalent Oxide Thickness of <0.5 nm for Nanoscale Electronic Semiconductor Memory, Adv. Funct. Mater., 2010, 20, 2989 CrossRef CAS
.
- S. A. Adonin, M. N. Sokolov and V. P. Fedin, Bismuth(III) Halide Complexes: New Structural Types and New Application Areas, Russ. J. Inorg. Chem., 2017, 62, 1789 CrossRef CAS
.
- B. Sun, L. Wei, H. Li and P. Chen, White-Light-Controlled Ferromagnetic and Ferroelectric Properties of Multiferroic Single-Crystalline BiFeO3 Nanoflowers at Room Temperature, J. Mater. Chem. C, 2014, 2, 7547 RSC
.
- D. Damjanovic, P. Muralt and N. Setter, Ferroelectric Sensors, IEEE Sens. J., 2001, 1, 191 CAS
.
- Y. Lee, J. Park, S. Cho, Y.-E. Shin, H. Lee, J. Kim, J. Myoung, S. Cho, S. Kang, C. Baig and H. Ko, Flexible Ferroelectric Sensors with Ultrahigh Pressure Sensitivity and Linear Response over Exceptionally Broad Pressure Range, ACS Nano, 2018, 12, 4045 CrossRef CAS PubMed
.
- X. Ren, Large electric-field-induced strain in ferroelectric crystals by point-defect-mediated reversible domain switching, Nat. Mater., 2004, 3, 91 CrossRef CAS PubMed
.
- R. I. Epstein and K. J. Malloy, Electrocaloric Devices Based on Thin-Film Heat Switches, J. Appl. Phys., 2009, 106, 064509–064501 CrossRef
.
- K. Aizu, Possible Species of Ferromagnetic, Ferroelectric, and Ferroelastic Crystals, Phys. Rev. B: Solid State, 1970, 2, 754 CrossRef
.
- J. Ma, U. Azhar, C. Zong, Y. Zhang, A. Xu, C. Zhai, L. Zhang and S. Zhang, Structured PVDF@BT Nanoparticles for Dielectric Materials: A Novel Composite to Prove the Dependence of Dielectric Properties on Ferroelectric Shell, Mater. Des., 2019, 164, 107556 CrossRef CAS
.
- J. M. Gregg, Ferroelectrics at the Nanoscale, Phys. Status Solidi A, 2009, 206, 577 CrossRef CAS
.
- G. C. Xu, W. Zhang, X. M. Ma, Y. H. Chen, L. Zhang, H. L. Cai, Z. M. Wang, R. G. Xiong and S. Gao, Coexistence of Magnetic and Electric Orderings in the Metal-Formate Frameworks of [NH4][M(HCOO)3], J. Am. Chem. Soc., 2011, 133, 14948 CrossRef CAS PubMed
.
- R. Samantaray, R. J. Clark, E. S. Choi, H. Zhou and N. S. Dalal, M3− x(NH4)XCrO8 (M = Na, K, Rb, Cs): A New Family of Cr5+-Based Magnetic Ferroelectrics, J. Am. Chem. Soc., 2011, 133, 3792 CrossRef CAS PubMed
.
- A. Stroppa, P. Barone, P. Jain, J. M. Perez-Mato and S. Picozzi, Hybrid Improper Ferroelectricity in a Multiferroic and Magnetoelectric Metal-Organic Framework, Adv. Mater., 2013, 25, 2284 CrossRef CAS PubMed
.
- Y. Y. Tang, W. Y. Zhang, P. F. Li, H. Y. Ye, Y. M. You and R. G. Xiong, Ultrafast Polarization Switching in a Biaxial Molecular Ferroelectric Thin Film: [Hdabco]ClO4, J. Am. Chem. Soc., 2016, 138, 15784 CrossRef CAS PubMed
.
- T. H. Moon, S. J. Oh and K. M. Ok, [((R)-C8H12N)4][Bi2Br10] and [((S)-C8H12N)4][Bi2Br10]: Chiral Hybrid Bismuth Bromides Templated by Chiral Organic Cations, ACS Omega, 2018, 3, 17895 CrossRef CAS PubMed
.
- W. Zhang and R. G. Xiong, Ferroelectric Metal-Organic Frameworks, Chem. Rev., 2012, 112, 1163 CrossRef CAS PubMed
.
- M. Ghasemi, M. Lyu, M. Roknuzzaman, J. H. Yun, M. Hao, D. He, Y. Bai, P. Chen, P. V. Bernhardt, K. Ostrikov and L. Wang, Phenethylammonium Bismuth Halides: From Single Crystals to Bulky-Organic Cation Promoted Thin-Film Deposition for Potential Optoelectronic Applications, J. Mater. Chem. A, 2019, 7, 20733 RSC
.
- I. W. H. Oswald, E. M. Mozur, I. P. Moseley, H. Ahn and J. R. Neilson, Hybrid Charge-Transfer Semiconductors: (C7H7)SbI4, (C7H7)BiI4, and Their Halide Congeners, Inorg. Chem., 2019, 58, 5818 CrossRef CAS PubMed
.
- B. Wagner, N. Dehnhardt, M. Schmid, B. P. Klein, L. Ruppenthal, P. Müller, M. Zugermeier, J. M. Gottfried, S. Lippert, M. U. Halbich, A. Rahimi-Iman and J. Heine, Color Change Effect in an Organic-Inorganic Hybrid Material Based on a Porphyrin Diacid, J. Phys. Chem. C, 2016, 120, 28363 CrossRef CAS
.
- R. Jakubas, G. Bator and Z. Ciunik, Halogenoantimonates(III) and halogenobismuthates(III)-a rich family of ferroelectric crystals, Ferroelectrics, 2003, 295, 3 CAS
.
- S. A. Adonin, I. D. Gorokh, D. G. Samsonenko, I. V. Korol‘kov, M. N. Sokolov and V. P. Fedin, Crystal Structures of Binuclear Bi(III) Chloride and Bromide Complexes with Some Cations—Alkylated Pyridine Derivatives, J. Struct. Chem., 2017, 58, 718 CrossRef CAS
.
- S. A. Adonin, E. V. Peresypkina, M. N. Sokolov and V. P. Fedin, Iodobismuthate Complex (Bu4N)3[Bi3I12]: Crystal Structure of a New Polymorph, J. Struct. Chem., 2015, 56, 795 CrossRef CAS
.
- S. A. Adonin, I. D. Gorokh, D. G. Samsonenko, A. S. Novikov, I. V. Korolkov, P. E. Plyusnin, M. N. Sokolov and V. P. Fedin, Binuclear and polymeric bromobismuthates complexes: Crystal structures and thermal stability, Polyhedron, 2019, 159, 318 CrossRef CAS
.
- S. A. Adonin, M. N. Sokolov and V. P. Fedin, Polyhalide-bonded metal complexes. Structural diversity in an electric
class of compounds, Coord. Chem. Rev., 2018, 367, 1 CrossRef CAS
.
- S. A. Adonin, M. N. Sokolov and V. P. Fedin, Polynuclear halide complexes of Bi(III): From structural diversity to the new properties, Coord. Chem. Rev., 2016, 312, 1 CrossRef CAS
.
- S. A. Adonin, A. N. Usoltsev, A. S. Novikov, B. A. Kolesov, V. P. Fedin and M. N. Sokolov, One- and Two-Dimensional Iodine-Rich Iodobismuthates(III) Complexes: Structure, Optical Properties, and Features of Halogen Bonding in the Solid State, Inorg. Chem., 2019, 58, 15479 CrossRef PubMed
.
- A. M. Goforth, M. A. Tershansy, M. D. Smith, L. R. Peterson, J. G. Kelley, W. J. I. DeBenedetti and H.-C. Zur Loye, Structural Diversity and Thermochromic Properties of Iodobismuthate Materials Containing D-Metal Coordination Cations: Observation of a High Symmetry [Bi3I11]2− Anion and of Isolated I− Anions, J. Am. Chem. Soc., 2011, 133, 603 CrossRef CAS PubMed
.
- I. D. Gorokh, S. A. Adonin, P. A. Abramov, A. S. Novikov, M. N. Sokolov and V. P. Fedin, New strucutral type in polybromide-bromometalate hybrids: (Me3NH)3{[Bi2Br9](Br2)} - Crystal structure and theoretical studies of noncovalent Br⋯Br interactions, Inorg. Chem. Commun., 2018, 98, 169 CrossRef CAS
.
- I. D. Gorokh, S. A. Adonin, D. G. Samsonenko, M. N. Sokolov and V. P. Fedin, Mono- and Binuclear Chloride and Bromide Complexes of Bi(III) with Double-Charged Cations Based on Pyridine: Synthesis and Crystal Structures, Russ. J. Coord. Chem., 2018, 44, 502 CrossRef CAS
.
- M. L. Liu and L. H. Kong, Two novel trimethylaulfonium salts with polymeric {[SbCl4]−}n or {[CdCl3]−}n anions, Acta Crystallogr., 2014, C70, 169 Search PubMed
.
- N. Mercier, N. Louvain and W. Bi, Structural Diversity and Retro-Crystal Engineering Analysis of Iodometalate Hybrids, CrystEngComm, 2009, 11, 720 RSC
.
- A. N. Usoltsev, M. Elshobaki, S. A. Adonin, L. A. Frolova, T. Derzhavskaya, P. A. Abramov, D. V. Anokhin, I. V. Korolkov, S. Y. Luchkin, N. N. Dremova, K. J. Stevenson, M. N. Sokolov, V. P. Fedin and P. A. Troshin, Polymeric Iodobismuthates {[Bi3I10]} and {[BiI4]} with N-Heterocyclic Cations: Promising Perovskite-like Photoactive Materials for Electronic Devices, J. Mater. Chem. A, 2019, 7, 5957 RSC
.
- S. A. Adonin, I. D. Gorokh, A. S. Novikov, P. A. Abramov, M. N. Sokolov and V. P. Fedin, Halogen Contacts-Induced Unusual Coloring in BiIII Bromide Complex: Anion-to-Cation Charge Transfer via Br⋯Br Interactions, Chem. – Eur. J., 2017, 23, 15612 CrossRef CAS PubMed
.
- S. A. Adonin, I. D. Gorokh, A. S. Novikov, D. G. Samsonenko, I. V. Korolkov, M. N. Sokolov and V. P. Fedin, Bromobismuthates: Cation-Induced Structural Diversity and Hirshfeld Surface Analysis of Cation–Anion Contacts, Polyhedron, 2018, 139, 282 CrossRef CAS
.
- S. A. Adonin, I. D. Gorokh, A. S. Novikov, D. G. Samsonenko, P. E. Plyusnin, M. N. Sokolov and V. P. Fedin, Bromine-Rich Complexes of Bismuth: Experimental and Theoretical Studies, Dalton Trans., 2018, 47, 2683 RSC
.
- S. K. Porter and R. A. Jacobson, Crystal Structure of Pyridinium Nonabromodiantimonate(III) Dibromide, J. Chem. Soc., 1970, 1359 RSC
.
- B. Blažič and F. Lazarini, Structure of Diethylammonium Tetrachlorobismuthate(III), Acta Crystallogr., 1985, C41, 1619 Search PubMed
.
- A. Gągor, G. Banach, M. Węcławik, A. Piecha-Bisiorek and R. Jakubas, The Lone-Pair-Electron-Driven Phase Transition and Order-Disorder Processes in Thermochromic (2-MIm)SbI4 Organic-Inorganic Hybrid, Dalton Trans., 2017, 46, 16605 RSC
.
- M. Owczarek, R. Jakubas, A. Pietraszko, W. Medycki and J. Baran, Investigation of Structure-Properties Relationship in a Novel Family of Halogenoantimonates(III) and Halogenobismuthates(III) with Morpholinium Cation: [NH2(C2H4)2O]MX4]. Crystal Structure, Phase Transitions and Dynamics of Molecule, Dalton Trans., 2013, 42, 15069 RSC
.
- I. Płowaś, P. Szklarz, R. Jakubas and G. Bator, Structural, Thermal and Dielectric Studies on the Novel Solution Grown (4-Dimethylaminopyridinium) Chloroantimonate(III) and Chlorobismuthate(III) Crystals, Mater. Res. Bull., 2011, 46, 1177 CrossRef
.
- B. Kulicka, V. Kinzhybalo, R. Jakubas, Z. Ciunik, J. Baran and W. Medycki, Crystal Structure and Phase Transition of 4-Aminopyridinium Tetrachlorobismuthate(III), [4-NH2C5H4NH][BiCl4], as Studied by x-Ray Diffraction, Dielectric, Proton NMR and Infrared Spectroscopy, J. Phys.: Condens. Matter, 2006, 18, 5087 CrossRef CAS
.
- R. Jakubas, Z. Ciunik and G. Bator, Ferroelectric Properties of [4-NH2C5H4NH][SbCl4], Phys. Rev. B: Condens. Matter Mater. Phys., 2003, 67, 241031 CrossRef
.
- A. Gągor, Phase Transitions in Ferroelectric 4-Aminopyridinium Tetrachloroantimonate(III) – Revisited, Acta Crystallogr., 2018, B74, 217 Search PubMed
.
- M. Wojtaś, R. Jakubas, J. Zaleski, G. Bator and J. Baran, The Phase Situation and Ferroelectric Properties in the Mixed Crystals, J. Mol. Struct., 2008, 887, 262–268 CrossRef
.
- G. Xu, Y. Li, W. W. Zhou, G. J. Wang, X. F. Long, L. Z. Cai, M. S. Wang, G. C. Guo, J. S. Huang, G. Bator and R. Jakubas, A Ferroelectric Inorganic-Organic Hybrid Based on NLO-Phore Stilbazolium, J. Mater. Chem., 2009, 19, 2179 RSC
.
- A. Lipka, Preparation and Crystal structures of 2,2′ Bipyridinium Pentachloroantimonate (C10H8N2H2)SbCl5 and of the Metastable Modification of 4,4′ bypiridinium pentachloroantimonate (C10H8N2H2)SbCl5, Z. Naturforsch., 1983, 38b, 1615 CAS
.
- U. Ensinger, W. Schwarz and A. Schmidt, Alkylammonium chloroantimonates(III). Structure and Vibrational Spectra, Z. Naturforsch., 1983, 38b, 149 CAS
.
- U. Ensinger, W. Schwarz and A. Schmidt, Tetraalkylammonium Tetrachloroantimonates(III). Structure and Vibrational spectra, Z. Naturforsch., 1982, 37b, 1584 CAS
.
- K. Kozawa and T. Uchida, Structures of Two Polymorphic Forms of Phenothiazine Tetrachloroantimonate, Acta Crystallogr., 1990, C46, 1006 CAS
.
- A. L. Rheingold, A. D. Uhler and A. G. Landers, Synthesis, Crystal Structure, and Molecular Geometry of [(η5-C5H5)2Fe]4[Bi4Br16], the Ferrocenium Salt of a “Cluster of Octahedra” Hexadecabromotetrabismuthate Counterion, Inorg. Chem., 1983, 22, 3255 CrossRef CAS
.
- L. Antolini, A. Benedetti, A. C. Fabretti and A. Giusti, Crystal and Molecular Structure, and Spectroscopic Characterization of Tetrakis(2-Amino-l,3,4-Thiadiazolium) Hexadecabromotetra-Antimonate(III), J. Chem. Soc., Dalton Trans., 1988, 2501 RSC
.
- U. Geiser, E. Wade, H. H. Wang and J. M. Williams, Structure of a new iodobismuthate: tetra(n-butyl)ammonium 1,2;1,2;1,2;2,3;2,3;2,3-hexa-μ-iodo-1,1,1,3,3,3 hexaiodotribismuthate(III) (3:1), Acta Crystallogr., 1990, C46, 1547 CAS
.
- B. Borgsen, F. Weller and K. Dehnicke, Uber Die Kronenetherkomplexe [K(15-Krone-5)2]3[Sb3I12], [TeCl3(15-Krone-5)][TeCl5] Und [TeCl3(15-Krone-5)]2[TeCl6], Z. Anorg. Allg. Chem., 1991, 596, 55 CrossRef CAS
.
- S. G. Bott, L. Brammer, N. G. Connelly, M. Green, A. G. Orpen, J. F. Paxton, C. J. Schaverien, S. Bristow and N. C. Norman, Reactions of Co-ordinated Ligands, Part 48. Reactivity Studies of the Octatrienediylidenedimolybdenum Complexes [Mo2(μ-C8Me8)(η-C5H5)2] and [Mo2(μ-C8H4But4)(η-C5H5)2]. Crystal structures of [Mo2(μ-C8Me7CH2)-(η-C5H5)2][CF3SO3], [Mo2(μ-C8Me8)(μ-Cl)2(η-C5H5)2][SbCl4]·CH2Cl2 and [Mo2(μ-C8H4But4)(μMοC,-H)2(η-C5H5)2][BPh4]·CH2Cl2, J. Chem. Soc., Dalton Trans., 1990, 2, 1957 RSC
.
- A. T. Mohammed and U. Muller, Tetraphenylphosphonium-Octabromodiantimonate, (PPh4)2[Sb2Br8]-Synthesis and Crystal Structure, Z. Naturforsch., 1985, 40b, 562 CAS
.
- S. Pohl, W. Saak, R. Lotz and D. Haase, On the Existence of Weak Interactions between Sb(III) and Phenyl Groups: Sb2Br6(SPPh3)2, Sb2I6(SePPh3)2·2CH2Cl2, Sb4Br12(SPMe2Ph)4 and (Ph4P)2Sb2Br8·CH3CN, Z. Naturforsch., 1990, 45b, 1355 Search PubMed
.
- N. W. Alcock, M. Ravindran and G. R. Willey, Crown Ether Complexes of BiIII. Synthesis and Crystal and Molecular Structures of BiCl3·12-Crown-4 and 2BiCl3·18-Crown-6, J. Chem. Soc., Chem. Commun., 1989, 52, 1063 RSC
.
- M. Wojciechowska, P. Szklarz, A. Białońska, J. Baran, R. Janicki, W. Medycki, P. Durlak, A. Piecha-Bisiorek and R. Jakubas, Enormous Lattice Distortion through an Isomorphous Phase Transition in an Organic-Inorganic Hybrid Based on Haloantimonate(III), CrystEngComm, 2016, 18, 6184 RSC
.
- A. Piecha-Bisiorek, K. Mencel, V. Kinzhybalo, A. Szota, R. Jakubas, W. Medycki and W. Zawrocki, Ferroelasticity and Piezoelectricity of Organic-Inorganic Hybrid Materials with a One-Dimensional Anionic Structure: So Similar, yet so Different, CrystEngComm, 2018, 20, 2112 RSC
.
- J. Belz, R. Wber, A. Roloff and B. Ross, Crystal structure of bis(Tert.-Butylammonium)- Di-μ -Chloro-Trichloroantimonate, (C4H12N)2Sb2Cl8, Z. Kristallogr. – Cryst. Mater., 1992, 202, 281 CAS
.
- H.-J. Lubberger, E. Muller, J. Hofmann, H. Fischer and J. C. Jochims, Reactions of 1-Thia-3-azoniabutatriene Salts with Alcohols, Carbonyl Compounds, Diazoalkanes, Nitrile Oxides, Nitrones, Enamines, and Butadienes, Chem. Ber., 1991, 124, 2537 CrossRef CAS
.
- K. Kihara and T. Sudo, The Crystal Structures of β-Cs3Sb2Cl9 and Cs3Bi2Cl9, Acta Crystallogr., 1974, B30, 1088 CrossRef
.
- R. Jakubas, Z. Czapla, Z. Galewski, L. Sobczyk, O. J. Żogał and T. Lis, Structure and Phase Transition in (CH3NH2)3Sb2Cl9, Phys. Status Solidi, 1986, 93, 449 CrossRef CAS
.
- R. Jakubas, G. Bator, J. Zaleski, A. Pietraszko and R. Decressain, The Structure and Phase Transition of (CH3NH3)3Sb2(1−X)Bi2xCl9 Mixed Crystals, J. Phys.: Condens. Matter, 1996, 8, 367 CrossRef CAS
.
- H. Ishihara, K. Yamada, T. Okuda and A. Weisz, The Structures of M2X93− (M = Bi: X = Cl, Br) Ions Determined by Rietveld Analysis of X-Ray Powder Diffraction Data, Bull. Chem. Soc. Jpn., 1993, 66, 380 CrossRef CAS
.
- P. Ciąpała, J. Zaleski, G. Bator, R. Jakubas and A. Pietraszko, The Structure and Phase Transition of Tris (n−Propylammonium) Enneachlorodiantimonate(III), (n-C3H7NH3)3Sb2Cl9, J. Phys.: Condens. Matter, 1996, 8, 1957 CrossRef
.
- V. G. Meyer and A. Schonemund, Zur Kenntnis der Hochtemperatur-Phasenumwandlung bei Cs3Bi2Cl9, Z. Anorg. Allg. Chem., 1980, 468, 185 CrossRef
.
- A. Piecha, V. Kinzhybalo, K. Ślepokura and R. Jakubas, Characterization, Thermal and Electric Properties of Imidazolium Bromoantimonate(III): [C3H5N2]3[Sb2Br9], J. Solid State Chem., 2007, 180, 265 CrossRef CAS
.
- M. Bujak and J. Zaleski, High temperature ferro-paraelectric phase transition in tris(trimethylammonium) nonachlorodiantimonate(III) (TMACA) studied by X-ray diffraction method, Cryst. Eng., 2001, 4, 241 CrossRef CAS
.
- R. Jakubas, E. Narewski and L. Sobczyk, High Frequency Dielectric Dispersion in [(CH3)3NH]3Sb2Cl9, Phys. Status Solidi, 1986, 98, K115 CrossRef CAS
.
- M. Wojciechowska, A. Gągor, A. Piecha-Bisiorek, R. Jakubas, A. Cizman, J. K. Zaręba, M. Nyk, P. Zieliński, W. Medycki and A. Bil, Ferroelectricity and Ferroelasticity in Organic Inorganic Hybrid (Pyrrolidinium)3[Sb2Cl9], Chem. Mater., 2018, 30, 4597 CrossRef CAS
.
- J. Mróz and R. Jakubas, Ferroelectric and Ferroelastic Phase Transitions in (CH3NH3)3Sb2Br9 Crystals, Ferroelectr. Lett. Sect., 1994, 17, 73 CrossRef
.
- R. Jakubas, G. Bator, L. Sobczyk and J. Mróz, Ferroelectrics Dielectric and Pyroelectric Properties Crystals in the Ferroelectric Phase Transition Regions, Ferroelectrics, 1994, 158, 43 CrossRef CAS
.
- G. Bator, R. Jakubas, L. Sobczyk and J. Mróz, Dielectric and Pyroelectric Properties of [N(CH3)3H]3Sb2Cl9 in the Low Temperature Region, Ferroelectrics, 1993, 141, 177 CrossRef CAS
.
- R. Jakubas and L. Sobczyk, Phase Transitions in Alkylammonium Halogenoantimonates and Bismuthates, Phase Transitions, 1990, 20, 163 CrossRef CAS
.
- R. Jakubas, U. Krzewska, G. Bator and L. Sobczyk, Structure and Phase Transition in (CH3NH3)3Bi2Br9. A Novel Improper Ferroelectrics, Ferroelectrics, 1988, 77, 129 CrossRef CAS
.
- J. Zaleski, C. Pawlaczyk, R. Jakubas and H.-G. Unruh, Related Content Structure and Dynamic Dielectric Behaviour of Review, J. Phys.: Condens. Matter, 2000, 12, 7509 CrossRef CAS
.
- A. Miniewicz and R. Jakubas, Pyroelectric Properties and Phase Transition in Tris(Dimethylammonium)nonabromodiantimonate(III), Solid State Commun., 1988, 67, 1079 CrossRef CAS
.
- J. Zaleski and A. Pietraszko, Structure at 200 and 298 K and X-Ray Investigations of the Phase Transition at 242 K of [NH2(CH3)2]3Sb2Cl9 (DMACA), Acta Crystallogr., 1996, B52, 287 CAS
.
- A. Kallel and J. W. Bats, Tris(Trimethylammonium) Nonachlorodiantimonate(III), [NH(CH3)3]3[Sb2Cl9], Acta Crystallogr., 1985, C41, 1022 CAS
.
- F. Lazarini, Tetraphenylphosphonium Enneabromodibismuthate(III), Acta Crystallogr., 1977, 33, 2686 CrossRef
.
- C. R. Hubbard and R. A. Jacobson, Molecular Bromine Bridging of SbIII2Br93− Anions and the Crystal Structure of Tetramethylammonium Nonabromodiantimonate(III)-Dibromine, Inorg. Chem., 1972, 11, 2247 CrossRef CAS
.
- M. Hall, M. Nunn, M. J. Begley and D. B. Sowerby, Nonahalogenodiantimon(III)ates; their preparation and the crystal structures of [Hpy]3[Sb2Cl9], [nMe4]3[Sb2Br9], and [nMe4]3[Sb2Br3Cl6], J. Chem. Soc., Dalton Trans., 1986, 1231 RSC
.
- M. Węcławik, A. Gągor, R. Jakubas, A. Piecha-Bisiorek, W. Medycki, J. Baran, P. Zieliński and M. Gałązka, Structure-Property Relationships in Hybrid (C3H5N2)3[Sb2I9] and (C3H5N2)3[Bi2I9] Isomorphs, Inorg. Chem. Front., 2016, 3, 1306 RSC
.
- P. Szklarz, R. Jakubas, A. Piecha-Bisiorek, G. Bator, M. Chański, W. Medycki and J. Wuttke, Organic-Inorganic Hybrid Crystals, (2,4,6-CH3PyH)3Sb2Cl9 and (2,4,6-CH3PyH)3Bi2Cl9. Crystal Structure Characterization and Tunneling of CH3 Groups Studied by 1H NMR and Neutron Spectroscopy, Polyhedron, 2018, 139, 249 CrossRef CAS
.
- B. Aurivillius and C. Stalhandske, The Crystal Structure of a Tetranuclear Bismuth(III) Complexes, (C5H5NH)6Bi4Cl18, Acta Chem. Scand., Ser. A, 1978, 32, 715 CrossRef
.
- A. Piecha, R. Jakubas, V. Kinzhybalo and W. Medycki, Crystal Structure, Dielectric Properties and Molecular Motions of Molecules in Thiazolium Halometalates(III): (C3H4NS)6M4Br18·2H2O (M = Sb, Bi), J. Mol. Struct., 2012, 1013, 55 CrossRef CAS
.
- A. Piecha, R. Jakubas, V. Kinzhybalo and T. Lis, Structural and Dielectric Properties of Thiazolium Chlorobismuthate(III) and Chloroantimonate(III), J. Mol. Struct., 2008, 887, 194 CrossRef CAS
.
- A. Piecha, R. Jakubas, A. Pietraszko, J. Baran, W. Medycki and D. Kruk, Structural Characterization, Thermal, Dielectric, Vibrational Properties and Molecular Motions in [C3N2H5]6[Bi4Br18], J. Solid State Chem., 2009, 182, 2949 CrossRef CAS
.
- A. Piecha, R. Jakubas and A. Pietraszko, Phase Transitions and Electric Properties of Imidazolium Chlorobismuthate(III): [C3H5N2]6[Bi4Cl18], J. Mol. Struct., 2007, 829, 149 CrossRef CAS
.
- M. Ksiądzyna, A. Gągor, A. Piecha-Bisiorek, A. Ciżman, W. Medycki and R. Jakubas, Exploring a Hybrid Ferroelectric with a 1-D Perovskite-like Structure: Bis(Pyrrolidinium) Pentachloroantimonate(III), J. Mater. Chem. C, 2019, 7, 10360 RSC
.
- Z. Yin, J. X. Gao, H. P. Chen and W. Q. Liao, An Above-Room-Temperature Phase Transition with Dielectric-Switching Properties in a Halogenobismuthate(III) – Tris(Cyclohexylmethylammonium) Pentabromobismuthate(III) Bromide, Eur. J. Inorg. Chem., 2017, 2017, 3555 CrossRef CAS
.
- A. Piecha-Bisiorek, A. Gągor, R. Jakubas, A. Ciżman, R. Janicki and W. Medycki, Ferroelectricity in Bis(Ethylammonium) Pentachlorobismuthate(III): Synthesis, Structure, Polar and Spectroscopic Properties, Inorg. Chem. Front., 2017, 4, 1281 RSC
.
- C. Y. Mao, W. Q. Liao, Z. X. Wang, Z. Zafar, P. F. Li, X. H. Lv and D. W. Fu, Temperature-Triggered Dielectric-Optical Duple Switch Based on an Organic-Inorganic Hybrid Phase Transition Crystal: [C5N2H16]2SbBr5, Inorg. Chem., 2016, 55, 7661 CrossRef CAS PubMed
.
- C. Y. Mao, W. Q. Liao, Z. X. Wang, P. F. Li, X. H. Lv, H. Y. Ye and Y. Zhang, Structural Characterization, Phase Transition and Switchable Dielectric Behaviors in a New Zigzag Chain Organic-Inorganic Hybrid Compound: [C3H7NH3]2SbI5, Dalton Trans., 2016, 45, 5229 RSC
.
- T. B. Rhaiem, H. Boughzala and A. Van Der Lee, Crystal Structure of a New Hybrid Antimony-Halide-Based Compound for Possible Non-Linear Optical Applications, Acta Crystallogr., 2015, E71, 498 CrossRef PubMed
.
- N. Mercier, The Templating Effect and Photochemistry of Viologens in Halometalate Hybrid Crystals, Eur. J. Inorg. Chem., 2013, 19 CrossRef
.
- N. Leblanc, N. Mercier, L. Zorina, S. Simonov, P. Auban-Senzier and C. Pasquier, Spontaneous Polarization and Clear Hysteresis Loop of a Room-Temperature Hybrid Ferroelectric Based on Mixed-Halide [BiI3Cl2] Polar Chains and Methylviologen Dication, J. Am. Chem. Soc., 2011, 133, 14924 CrossRef CAS PubMed
.
- M. Owczarek, P. Szklarz, R. Jakubas and A. Miniewicz, MX5: A New Family of Morpholinium Nonlinear Optical Materials among Halogenoantimonate(III) and Halogenobismuthate(III) Compounds. Structural Characterization, Dielectric and Piezoelectric Properties, Dalton Trans., 2012, 41, 7285 RSC
.
- M. Moskwa, G. Bator, M. Rok, W. Medycki, A. Miniewicz and R. Jakubas, Investigations of Organic-Inorganic Hybrids Based on Homopiperidinium Cation with Haloantimonates(III) and Halobismuthates(III). Crystal Structures, Reversible Phase Transitions, Semiconducting and Molecular Dynamic Properties, Dalton Trans., 2018, 47, 13507 RSC
.
- F. Bigoli, M. Lanfranchi and M. A. Pellinghelli, The structures of bis(1H+,5H+-S-methylisothiocarbonohydrazidium) di-μ-chloro-octachlorodibismuthate(III) tetrahydrate and tris(1H+-S-methylisothiocarbonohydrazidium) esachlorobismuthate(III), Inorg. Chim. Acta, 1984, 90, 215 CrossRef CAS
.
- A. Piecha, V. Kinzhybalo, R. Jakubas, J. Baran and W. Medycki, Structural Characterization, Molecular Dynamics, Dielectric and Spectroscopic Properties of Tetrakis(Pyrazolium) Bis(M2-Bromo-Tetrabromobismuthate(III)) Dihydrate, [C3N2H5]4[Bi2Br10]·2H2O, Solid State Sci., 2007, 9, 1036 CrossRef CAS
.
- M. Moskwa, G. Bator, A. Piecha-Bisiorek, R. Jakubas, W. Medycki, A. Ciżman and J. Baran, X-Ray Structure and Investigation of Molecular Motions by Dielectric, Vibrational and 1H NMR Methods for Two Organic-Inorganic Hybrid Piperazinium Compounds: (C4H12N2)2[Sb2Cl10]·2H2O and (C4H12N2)2[Sb2Br10]·2H2O, Mater. Res. Bull., 2018, 104, 202 CrossRef CAS
.
- R. Jakubas, A. Piecha, A. Pietraszko and G. Bator, Structure and Ferroelectric Properties of (C3N2H5)5Bi2Cl11, Phys. Rev. B: Condens. Matter Mater. Phys., 2005, 72, 1 CrossRef
.
- A. Piecha, A. Białońska and R. Jakubas, Structure and Ferroelectric Properties of [C3N2H 5]5[Bi2Br11], J. Phys.: Condens. Matter, 2008, 20, 325224 CrossRef
.
- J. Jóźków, R. Jakubas, G. Bator and A. Pietraszko, Ferroelectric Properties of (C5H5NH)5Bi2Br11, J. Chem. Phys., 2001, 114, 7239 CrossRef
.
- C. Pawlaczyk, H. Motsch, R. Jakubas and H.-G. Unruh, Dielectric behaviour of the new ferroelectric (CH3NH3)5Bi2Br11 in the microwave region, Ferroelectrics, 1990, 108, 127 CrossRef CAS
.
- C. Pawlaczyk, R. Jakubas, K. Planta, C. Bruch, J. Stephan and H.-G. Unruh, Dynamic Dielectric Behaviour of (CH3NH3)5Bi2X11 (X: Br, CI) Single Crystals, Ferroelectrics, 1992, 126, 145 CrossRef CAS
.
- M. Połomska and R. Jakubas, Dynamics of Ferroelectric Domains in (CH3NH3)5Bi2Br11 Studied by Liquid Crystal Decoration Technique, Ferroelectrics, 1990, 106, 57 CrossRef
.
- J. Lefebvre, P. Carpentier and R. Jakubas, Structures and Phase Transition in the Ferroelectric Crystal of Pentakis(Methylammonium) Undecachlorodibismuthate(III): [NH3(CH3)]5Bi2Cl11, Acta Crystallogr., 1991, B47, 228 CAS
.
- P. Carpentier, J. Lefebvre and R. Jakubas, Structure of Pentakis(Methylammonium) Undecachlorodibismuthate(III), [NH3(CH3)]5Bi2Cl11, at 130 K and Mechanism of the Phase Transitions, Acta Crystallogr., 1995, B51, 167 Search PubMed
.
- R. Jakubas, A New Ferroelectric Compound: (CH3NH3)5Bi2Br11, Solid State Commun., 1989, 69, 267 CrossRef CAS
.
- R. Cach and R. Jakubas, Determination of Landau-energy parameters by dielectric measurements in (CH3NH3)5Bi2Cl11 crystals, Ferroelectrics, 1990, 108, 121 CrossRef CAS
.
- A. Piecha, A. Pietraszko, G. Bator and R. Jakubas, Structural Characterization and Ferroelectric Ordering in (C3N2H5)5Sb2Br11, J. Solid State Chem., 2008, 181, 1155 CrossRef CAS
.
- F. Lazarini, Structure of Diethylammonium Hexabromobismuthate (III), Acta Crystallogr., 1985, C41, 1617 CAS
.
- W. G. McPherson and E. A. Meyers, The Crystal Structures of Bismuth Halide Complex Salts. III. Tris(Dimethylammonium) Hexabromobismuthate(III), [(CH3)2NH2]3BiBr6, J. Phys. Chem., 1968, 72, 3117 CrossRef CAS
.
- L. R. Morrs and W. R. Robinson, Crystal Structure of Cs2NaBiCl6, Acta Crystallogr., 1972, B28, 653 CrossRef
.
- F. Lazarini, Structure of Diethylammonium Hexachlorobismuthate(III), Acta Crystallogr., 1987, C43, 637 CAS
.
- S. Jarraya, A. B. Salah, A. Daoud, W. Rothammel and H. Burzlaff, Structure of [(C2H5)2NH2]3BiCl6, Acta Crystallogr., 1993, C49, 1594 CAS
.
- M. Bujak, Formation and Distortion of Iodidoantimonates(III): The First Isolated [SbI6]3− Octahedron, Acta Crystallogr., 2017, B73, 432 Search PubMed
.
- A. Piecha, A. Gągor, M. Węcławik, R. Jakubas and W. Medycki, Anomalous Dielectric Behaviour in Centrosymmetric Organic-Inorganic Hybrid Chlorobismuthate(III) Containing Functional N,N-Dimethylethylammonium Ligand. Crystal Structure and Properties, Mater. Res. Bull., 2013, 48, 151 CrossRef CAS
.
- M. Chański, A. Białońska, R. Jakubas and A. Piecha-Bisiorek, Structural Characterization and Properties of Bis (1,4-H2-1,2,4-Triazolium) Pentachlorobismuthate (III) and Cocrystal of Ammonium Chloride with Tris (1,4-H2-1,2,4-Triazolium) Hexachlorobismuthate (III), Polyhedron, 2014, 71, 69 CrossRef
.
- I. Płowaś, G. Bator, R. Jakubas and J. Baran, Thermal, Dielectric and Vibrational Properties of Allylammonium Chloroantimonates(III) and Chlorobismuthates(III): [C3H5NH3]3[BiCl6] and [C3H5NH3]3[SbCl5]Cl, Vib. Spectrosc., 2012, 62, 121 CrossRef
.
- I. Płowaś, A. Białońska, G. Bator, R. Jakubas, W. Medycki and J. Baran, Tris(Allylammonium) Hexabromobismuthate(III) - Crystal Structure, Phase Transitions and Thermal, Dielectric, Vibrational and 1H NMR Properties over a Range of Temperatures, Eur. J. Inorg. Chem., 2012, 636 CrossRef
.
- J. Tarasiewicz, R. Jakubas, G. Bator, J. Zaleski, J. Baran and W. Medycki, Structural Characterization, Thermal, Dielectric, Vibrational Properties and Molecular Dynamics of (C5H5NH)3BiCl6, J. Mol. Struct., 2009, 932, 6 CrossRef CAS
.
- B. Kulicka, R. Jakubas, B. Bednarska-Bolek, G. Bator and Z. Ciunik, Crystal Structure and Phase Transitions in Dipropylammonium Hexachloroantimonate(V): [N(C3H7)2H2][SbCl6], J. Mol. Struct., 2006, 792–793, 151 CrossRef CAS
.
- L. Sobczyk, R. Jakubas and J. Zaleski, Self-Assembly of Sb(III) and Bi(III) Halo-Coordinated Octahedra in Salts of Organic Cations. Structure, Properties and Phase Transitions, Pol. J. Chem., 1997, 71, 265 CAS
.
- R. D. Rogers, A. H. Bond and S. Aguinaga, Alcoholysis of Bi(NO3)3·5H2O by Polyethylene Glycols. Comparison with Bismuth(III) Nitrate Crown Ether Complexation, J. Am. Chem. Soc., 1992, 114, 2960 CrossRef CAS
.
- S. Pohl, R. Lötz, D. Haase and W. Saak, Iodoantimonates of compositions A+Sb2I7−: Discrete Anions in (Me4N)4Sb8I28 and (Me3S)4Sb8I28, polymeric chains in (Me3NCH2Ph)Sb2I7 (Me = CH3, Ph = C6H5), Z. Naturforsch., 1988, 43b, 1144 Search PubMed
.
- S. Pohl, W. Saak and D. Haase, Synthesis and crystal structure of (Ph4P)4Sb8I28 and (Ph4P)Sb3I10 (Ph = C6H5), Z. Naturforsch., 1987, 42b, 1493 Search PubMed
.
- B. S. Pohl, W. Saak, P. Mayer and A. Schmidpeter, [Sb3110−]∞ A Polymeric Anion with Transitions from Trigonal Pyramidal to Octahedral Coordination of Antimony(III), Angew. Chem., Int. Ed. Engl., 1986, 25, 825 CrossRef
.
- C. J. Carmalt, N. C. Norman and L. J. Farrugia, The Structure of an Unusual Polymeric Iodoantimonate(III) Anion with Disordered Antimony Sites, Polyhedron, 1994, 13, 1655 CrossRef
.
- S. Pohl, M. Peters, D. Haase and W. Saak, Formation of Iodoantimonates and Bismuthates. Crystal Structures of (PhCH2NEt3)4[Sb6I22], (PhCH2NEt3)4[Bi6I22] and (Ph4P)3[Bi5I18], Z. Naturforsch., 1994, 49b, 741 Search PubMed
.
- S. Pohl, R. Lotz, W. Saak and D. Haase, Structural Diversity in Iodoantimonates; the Anions Sb3I112−, Sb5I183− and Sb6I224−, Angew. Chem., Int. Ed. Engl., 1989, 28, 344 CrossRef
.
- S. Pohl, D. Haase, R. Lötz and W. Saak, [Fe(phen)3]2Sb6I22·2CH3CN: An Iodoantimonate with Central [Sb6I6]-Double Cube (phen = 1,10-Phenanthrolin), Z. Naturforsch., 1988, 43b, 1033 Search PubMed
.
- T. Zaleski, T. Głowiak, R. Jakubas and L. Sobczyk, Crystal structure and phase transitions of [(C2H5)4N]6Bi8Cl30, J. Phys. Chem. Solids, 1989, 50, 1265 CrossRef
.
- G. Bator and R. Jakubas, Dynamical Dielectric Properties of [4-NH2C5H4NH][SbCl4] in the Incommensurate Phase, J. Phys. Soc. Jpn., 2003, 72, 2369 CrossRef CAS
.
- R. Jakubas, J. Zaleski and L. Sobczyk, Phase Transitions in (CH3NH3)3Bi2I9 (MAIB), Ferroelectrics, 1990, 108, 109 CrossRef CAS
.
- M. E. Kamminga, A. Stroppa, S. Picozzi, M. Chislov, I. A. Zvereva, J. Baas, A. Meetsma, G. R. Blake and T. T. M. Palstra, Polar Nature of (CH3NH3)3Bi2I9 Perovskite-Like Hybrids, Inorg. Chem., 2017, 56, 33 CrossRef CAS PubMed
.
- C. Ji, Z. Sun, A. Zeb, S. Liu, J. Zhang, M. Hong and J. Luo, Bandgap Narrowing of Lead-Free Perovskite-Type Hybrids for Visible-Light-Absorbing Ferroelectric Semiconductors, J. Phys. Chem. Lett., 2017, 8, 2012 CrossRef CAS PubMed
.
- Z. Sun, A. Zeb, S. Liu, C. Ji, T. Khan, L. Li, M. Hong and J. Luo, Exploring a Lead-Free Semiconducting Hybrid Ferroelectric with a Zero-Dimensional Perovskite-like Structure, Angew. Chem., Int. Ed., 2016, 55, 11854 CrossRef CAS PubMed
.
- P. Szklarz, A. Gągor, R. Jakubas, P. Zieliński, A. Piecha-Bisiorek, J. Cichos, M. Karbowiak, G. Bator and A. Cizman, Lead-Free Hybrid Ferroelectric Material Based on Formamidine: [NH2CHNH2]3Bi2I9, J. Mater. Chem. C, 2019, 7, 3003 RSC
.
- P. Szklarz, R. Jakubas, A. Gągor, G. Bator, J. Cichos and M. Karbowiak, [NH2CHNH2]3Sb2I9: A Lead-Free and Low-Toxicity Organic–Inorganic Hybrid Ferroelectric Based on Antimony(III) as a Potential Semiconducting Absorber, Inorg. Chem. Front., 2020, 7, 1780 RSC
.
- J. Zhang, S. Han, C. Ji, W. Zhang, Y. Wang and K. Tao, [(CH3)3NH]3Bi2I9: A Polar Lead-Free Hybrid Perovskite-Like Material as a Potential Semiconducting Absorber, Chem. – Eur. J., 2017, 23, 17304 CrossRef CAS PubMed
.
- H. Y. Zhang, Z. Wei, P. F. Li, Y. Y. Tang, W. Q. Liao, H. Y. Ye, H. Cai and R. G. Xiong, The Narrowest Band Gap Ever Observed in Molecular Ferroelectrics: Hexane-1,6-Diammonium Pentaiodobismuth(III), Angew. Chem., Int. Ed., 2018, 57, 526–530 CrossRef CAS PubMed
.
- Y. Wang, C. Shi and X.-B. Han, Organic–Inorganic Hybrid [H2mdap][BiCl5] Showing an above-Room-Temperature Ferroelectric Transition with Combined Order–Disorder and Displacive Origins, Polyhedron, 2017, 133, 132 CrossRef CAS
.
- P. Li, Y. Tang, W. Liao, H. Ye, Y. Zhang, D. Fu and Y. You, Semiconducting Molecular Ferroelectric with a Bandgap Much Lower than That of BiFeO3, NPG Asia Mater., 2017, 9, 1 CrossRef
.
- W. Bi, N. Leblanc, N. Mercier, P. Auban-Senzier and C. Pasquier, Thermally Induced Bi(III) Lone Pair Stereoactivity: Ferroelectric Phase Transition and Semiconducting Properties of (MV)BiBr5 (MV = Methylviologen), Chem. Mater., 2009, 21, 4099 CrossRef CAS
.
- A. Piecha, A. Białońska and R. Jakubas, Novel Organic-Inorganic Hybrid Ferroelectric: Bis(Imidazolium) Pentachloroantimonate(III), (C3N2H5)2SbCl5, J. Mater. Chem., 2012, 22, 333 RSC
.
- R. Jakubas, A. Gągor, M. J. Winiarski, M. Ptak, A. Piecha-Bisiorek and A. Ciżman, Ferroelectricity in Ethylammonium Bismuth-Based Organic-Inorganic Hybrid: (C2H5NH3)2[BiBr5], Inorg. Chem., 2020, 59, 3417 CrossRef CAS PubMed
.
- W. P. Zhao, C. Shi, A. Stroppa, D. Di Sante, F. Cimpoesu and W. Zhang, Lone-Pair-Electron-Driven Ionic Displacements in a Ferroelectric Metal-Organic Hybrid, Inorg. Chem., 2016, 55, 10337 CrossRef CAS PubMed
.
- B. Wang, D. Ma, H. Zhao, L. Long and L. Zheng, Room Temperature Lead-Free Multiaxial Inorganic-Organic Hybrid Ferroelectric, Inorg. Chem., 2019, 58, 13953–13959 CrossRef CAS PubMed
.
- A. Piecha, A. Gągor, A. Pietraszko and R. Jakubas, Unprecedented Solid-State Chemical Reaction - From (C3N2H5)3SbBr6·H2O to (C3N2H5)5Sb2Br11. From Centrosymmetric to Non-Centrosymmetric Crystal Structure, J. Solid State Chem., 2010, 183, 3058 CrossRef CAS
.
- K. Mencel, A. Gągor, R. Jakubas, A. Ciżman, W. Medycki, J. K. Zaręba and A. Piecha-Bisiorek, Ferroelectricity in Lead Free Organic-Inorganic 0D Hybrid: Formamidinium Bromoantimonate(III), J. Mater. Chem. C 10.1039/d0tc00168f
.
- H. Y. Ye, Y. Y. Tang, P. F. Li, W. Q. Liao, J. X. Gao, X. N. Hua, H. Cai, P. P. Shi, Y. M. You and R. G. Xiong, Metal-Free Three-Dimensional Perovskite Ferroelectrics, Science, 2018, 361, 151–155 CrossRef CAS PubMed
.
- Y. M. You, W. Q. Liao, D. Zhao, H. Y. Ye, Y. Zhang, Q. Zhou, X. Niu, J. Wang, P. F. Li, D. W. Fu, Z. Wang, S. Gao, K. Yang, J. M. Liu, J. Li, Y. Yan and R. G. Xiong, An Organic-Inorganic Perovskite Ferroelectric with Large Piezoelectric Response, Science, 2017, 357, 306–309 CrossRef CAS PubMed
.
- W. Q. Liao, D. Zhao, Y. Y. Tang, Y. Zhang, P. F. Li, P. P. Shi, X. G. Chen, Y. M. You and R. G. Xiong, A Molecular Perovskite Solid Solution with Piezoelectricity Stronger than Lead Zirconate Titanate, Science, 2019, 363, 1206–1210 CrossRef CAS PubMed
.
- W. Li, Z. Wang, F. Deschler, S. Gao, R. H. Friend and A. K. Cheetham, Chemically Diverse and Multifunctional Hybrid Organic-Inorganic Perovskites, Nat. Rev. Mater., 2017, 2, 16099 CrossRef
.
|
This journal is © the Partner Organisations 2020 |