DOI:
10.1039/C9QI01198F
(Research Article)
Inorg. Chem. Front., 2020,
7, 150-156
RbNa(HC3N3O3)·2H2O exhibiting a strong second harmonic generation response and large birefringence as a new potential UV nonlinear optical material†
Received
17th September 2019
, Accepted 14th October 2019
First published on 14th October 2019
Abstract
A new mixed alkali hydro-isocyanurate, RbNa(HC3N3O3)·2H2O, with the noncentrosymmetric space group Pna21 (No. 33), has been successfully grown by a hydrothermal method. The structure can be described as 2D wavy [Na(HC3N3O3)O4]∞ layers separated by Rb atoms. The UV-vis diffuse reflection measurement revealed that RbNa(HC3N3O3)·2H2O has a large band gap of 5.10 eV, corresponding to the UV cutoff edge of 241 nm. The polarizing microscope measurement of a micron-sized single crystal shows that RbNa(HC3N3O3)·2H2O has a large birefringence of 0.194. The powder SHG measurement indicated that RbNa(HC3N3O3)·2H2O exhibits a strong SHG effect that is 5.3 times that of KH2PO4 (KDP) and can realize phase-matching in the visible and UV region. Moreover, the differences in the SHG responses between RbNa(HC3N3O3)·2H2O and two isostructural compounds have been investigated, which depend heavily on the structural modulation of the (HC3N3O3)2− group by cations. Meanwhile, the electronic structures and optical properties were well analyzed using DFT methods.
Introduction
Nonlinear optical (NLO) crystals that can generate ultraviolet (UV) coherent light are of considerable interest in the fields of laser processing, optical communication and photoetching.1–7 To date, the most famous UV NLO crystal is β-BaB2O4 (BBO)8 due to its strong SHG effect (∼5 × KDP), short UV cutoff edge (190 nm) and large birefringence (Δn = 0.12@1064 nm), which has been widely used in second-, third-, fourth-, and fifth-harmonic generation. According to the anionic group theory, the superior linear and nonlinear properties of BBO should originate from its large π-conjugated delocalized (B3O6)3− anionic group, which suggests that introducing large π-conjugated groups into NLO crystals would be a very efficient way for achieving excellent NLO performance. However, it is noteworthy that non-centrosymmetric (NCS) borates containing a large π-conjugated (B3O6)3− group are rarely reported. In addition to BBO, there are only γ-KBe2B3O7,9 CsZnB3O7,10,11 LiB6O9F,12 CsB4O6F
13 and Cs2Al2(B2O6)2O.14 Therefore, this urges materials scientists to expand the research scope of large π-conjugated groups to search for new high performance UV NLO crystals.
In 2013, the Meyer group first found that the (C3N3O3)3− group has a highly similar structure to the (B3O6)3− group. Then, they successfully synthesized the first NLO-active cynaurates, M3(C3N3O3) (M = Ca, Sr), containing the (C3N3O3)3− group, via cyclo-trimerization of linear (CNO)− groups under vacuum conditions, which are isostructural to BBO but exhibit more superior linear and nonlinear optical properties than BBO.15,16 In 2017, the Lin group confirmed that the large π-conjugated (C3N3O3)3− group is an ideal fundamental building block for UV NLO crystals in theory.17 These studies show that cynaurates have potential applications as UV NLO crystals.
More recently, our group further proposed that hydroisocyanurates may be more suitable candidates for UV NLO crystals because they not only have the large π-conjugated (HC3N3O3)2− and (H2C3N3O3)− groups with high nonlinearity and strong optical anisotropy, the same as the (C3N3O3)3− group, but more importantly, their bulk single crystals are easy to grow via a simple water solution method. Accordingly, we successfully found the first hydro-isocyanurate NLO crystal, KLi(HC3N3O3)·2H2O, via a solvent-drop grinding method and grew bulk single crystals through the water solution technique.18 Afterward, several hydroisocyanurate crystals have been reported including K2(HC3N3O3),19 M2N(H2C3N3O3)4·6H2O (M/N = Li/Ca and Na/Ba),20 RE(H2C3N3O3)2·(OH)·nH2O (RE = La, Y and Gd),21 A2B(H2C3N3O3)4·nH2O (A = K, Rb, Cs; B = Mg, Ca; n = 4, 10),22 RbLi(HC3N3O3)·2H2O
23 and Cs3Na(H2C3N3O3)4·3H2O.24 Among them, however, only RbLi(HC3N3O3)·2H2O and Cs3Na(H2C3N3O3)4·3H2O are NLO-active. Thus, it is very essential to find more hydro-isocyanurates or dihydro-isocyanurates as UV-NLO materials.
In this work, we have selected the RbOH–NaOH–H3C3N3O3 system to further search for promising hydroisocyanurate NLO crystals. As a result, a new mixed alkali NCS isocyanurate, RbNa(HC3N3O3)·2H2O, with a strong SHG effect and large birefringence, has been successfully grown through the hydrothermal method. Detailed in this study are the syntheses, crystal structures, and the relationship between the structure and NLO properties.
Experimental
Reagents and synthesis
In the synthesis of RbNa(HC3N3O3)·2H2O, the reaction mixture of RbOH·H2O (2.41 g, 0.02 mol, 99%, Alfa), NaOH (0.80 g, 0.02 mol, 96%, Sinopharm), H3C3N3O3 (2.58 g, 0.02 mol, 98%, Adamas) and H2O (5.0 mL) was sealed in an autoclave with a Teflon liner (200 mL). After heating at 120 °C for 2 hours, the mixture was slowly cooled down to ambient temperature at a rate of 20 °C h−1. The product was washed with acetone, and then dried in air. Colorless rod-like millimeter-sized crystals were obtained (as shown in Fig. 1).
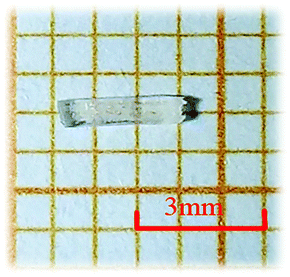 |
| Fig. 1 Photos of RbNa(HC3N3O3)·2H2O crystals. | |
Single crystal X-ray diffraction
Single crystal X-ray diffraction data of the title compounds were collected at room temperature on a Rigaku Mercury CCD diffractometer with graphite-monochromatic Mo Kα radiation (λ = 0.71073 Å). A transparent block of the crystal was mounted on a glass fiber with epoxy for structure determination. The intensity data sets were corrected with the ω-scan technique. The data were integrated with the CrystalClear program. The intensities were corrected for Lorentz polarization, air absorption, and absorption attributable to the variation in the path length through the detector faceplate. Absorption corrections were also applied based on the multi-scan technique. The structures were solved using direct methods, refined with difference Fourier maps and full-matrix least-squares fitting on F2 using SHELXL-97.25 In addition, the PLATON26 program was used for checking all the structures. The details of the crystallographic data and structure refinement information for the title compounds are listed in Table 1. Atomic coordinates and isotropic displacement coefficients are listed in Table S1, and bond lengths are listed in Table S2 of the ESI.†
Table 1 Crystal data and structure refinement of RbNa(HC3N3O3)·2H2O
Formula |
C6H10N6Na2O10Rb2 |
R(F) = ∑‖Fo| − |Fc‖/∑|Fo|·wR(Fo2) = [∑w(Fo2 − Fc2)2/∑w(Fo2)2]1.
|
Formula weight (g mol−1) |
543.10 |
Crystal system |
Orthorhombic |
Space group |
Pna21 (33) |
a (Å) |
15.829(18) |
b (Å) |
3.964(5) |
c (Å) |
13.068(16) |
α = β = γ |
90 |
Cell volume (Å3) |
819.8(17) |
Z
|
2 |
ρ (calcd) (g cm−3) |
2.200 |
T (K) |
293(2) |
λ (Å) |
0.71073 |
F(000) |
552 |
μ (mm−1) |
6.087 |
R/wRa (I > 2σ(I)) |
0.0458/0.1061 |
R/wRa (all data) |
0.0532/0.1085 |
GOF on F2 |
1.044 |
Powder X-ray diffraction
Powder X-ray diffraction (PXRD) patterns of the polycrystalline title compounds were collected at room temperature on a Mini-flex 600 Powder X-ray diffractometer using Cu Kα radiation (λ = 1.540598 Å) in the 2θ = 10–70° range. The PXRD patterns of the pure powder samples of all compounds showed good agreement with the calculated PXRD patterns from the single crystal models (see Fig. S1†).
Thermal analysis
Thermogravimetric analyses (TGA) were carried out using a NETZSCH STA449F3 simultaneous thermal analyser. The reference (Al2O3) and crystal samples (5–15 mg) were enclosed in Al2O3 crucibles and heated from 30 °C to 1000 °C at a heating rate of 10 °C min−1 under a constant flow of nitrogen gas.
UV-vis diffuse reflectance spectroscopy
The UV-vis diffuse reflectance powder spectra of the sample were measured at room temperature with BaSO4 as the standard with 100% reflectance. Data were collected on a PerkinElmer Lamda-950 UV/vis/NIR spectrophotometer scanning in the range of 190–800 nm. Reflectance values were converted into absorbance according to the Kubelka–Munk function.27,28
Infrared spectroscopy
IR spectra were recorded as KBr pellets on a Magna 750 Fourier transform infrared (FT-IR) spectrometer in the range of 4000–400 cm−1 (see Fig. S9†).
Birefringence
The retardation and thickness of the title compound were measured using a Nikon ECLIPSE LV100 POL polarizing microscope with 589.6 nm light. The formula for calculating birefringence was as follows:
Δn represents the birefringence, ΔR denotes the optical path difference, and T indicates the thickness of the crystal.
Second harmonic generation
Powder SHG responses were investigated using a Q-switched Nd:YAG solid-state laser with a fundamental wavelength of 1064 nm with frequency doubling at 532 nm, for visible and UV SHG, respectively. According to the model source by Kurtz–Perry,29 SHG responses depend strongly on the particle size. Polycrystalline samples of the title compounds were ground and sieved into the following particle size ranges: 25–45, 45–62, 62–75, 75–109, 109–150, and 150–212 μm. Cover slides were secured with tape in 1 mm thick plastic holders with an 8 mm diameter hole. KDP and BBO as the standard samples were prepared through grinding and sieving into identical particle size ranges in the same way. The ground samples were pressed into 1 mm thick aluminum holders containing a hole with a diameter of 8 mm and covered by glass microscope cover slides. Then these samples were put in an antiglare box and irradiated with laser waves at 1064 nm and 532 nm, respectively. In order to avoid the effect of the background flash lamp, a cutoff filter was employed. Furthermore, the second harmonic signal was selected by an interference filter (530 ± 10 nm), and then detected using a photomultiplier tube attached to a RIGOL DS1052E 50 MHz oscilloscope. The above procedures were repeated for the reference samples KDP and BBO. The proportion of the second harmonic intensity signal outputs between the title compounds and reference samples was calculated. All experiments were performed without an index-matching fluid.
Electronic structure calculations
Band structure and density of states (DOS) were determined using density functional theory (DFT) calculations provided by the CASTEP30 code in the Material Studio package. The experimental crystal structure was used without further optimization. The exchange correlation interaction was treated by the generalized gradient approximation (GGA) of Perdew–Burke–Ernzerhof (PBE).31 The energy cutoff for the plane wave was chosen as 780 eV, and the self-consistent convergence of the total energy was set to 2.0 × 10−5 eV per atom. The Monkhorst–Pack32k-point sampling of 2 × 2 × 4 in the Brillouin zone of the unit cell was selected for calculation. The interactions between the core and valence electrons were represented by the norm-conserving pseudopotentials, and the following valence configurations were observed: Na, 2s22p63s1; Rb, 4s24p65s1; C, 2s22p2; N, 2s22p3; O, 2s22p4.
SHG coefficient calculations
The classical anharmonic oscillator (AHO) model33 was adopted to calculate the SHG coefficients. The formula of the calculated second-order susceptibilities derived from the AHO model can be expressed in terms of the first-order susceptibilities as follows:
χijk(2)(−ω3;ω1;ω2) = F(2)χii(1)(ω3)χjj(1)(ω1)χkk(1)(ω2). |
The first-order susceptibility χii(1) in the low-frequency region is given by χ(1)(ω)ii = [ε(ω)ii − 1]/4π where the dielectric function
where
δ[
Ec(
k) −
Ev(
k) − ℏ
ω] denotes the energy difference between the conduction and valence bands at the
k point with absorption of a quantum ℏ
ω.
fc and
fv represent the Fermi distribution functions of the conduction and valence bands, respectively. It is well acknowledged that DFT-GGA calculations commonly underestimate the band gap of the crystal. Consequently, to match the experimental band gap, the scissor operation should be employed to shift up the conduction band energy.
Results and discussion
Crystal structure
RbNa(HC3N3O3)·2H2O crystallizes into an orthorhombic crystal system with a non-centrosymmetric space group Pna21 (no. 33). In the structure, the fundamental building blocks are the (HC3N3O3)2− groups. The bond distances of C–N and C–O vary from 1.31 to 1.38 Å and 1.20 to 1.29 Å, respectively, which accord with the values in the reported cyanurates. Also, there is one crystallographically independent Na atom in its unit cell, which is coordinated to five O atoms to form a distorted trigonal bi-pyramidal NaO5 configuration with Na–O bond lengths of 2.28–2.53 Å. The adjacent NaO5 polyhedra connect each other to form a 1D chain via sharing the common oxygen atom (Fig. 2b). Furthermore, 1D NaO5 chains were bridged via (HC3N3O3)2− groups to construct intricate 2D wavy [Na(HC3N3O3)O4]∞ layers (Fig. 2c), which were separated by Rb atoms.
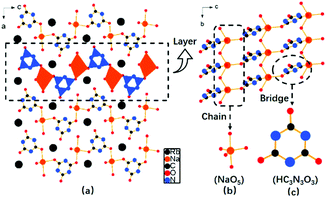 |
| Fig. 2 (a) Crystal structure of RbNa(HC3N3O3)·2H2O; (b) layers consisting of a 1D (NaO5) chain and (c) (HC3N3O3) triangular entities. | |
Thermal analysis
As shown in Fig. S3,† RbNa(HC3N3O3)·2H2O exhibits two-step decomposition in the range of 30–600 °C. The first weight loss in the temperature range of 181–151 °C was 13.30% (cal. 13.30%), which can be assigned to the removal of two water molecules from the crystal structure. The second weight loss in the temperature ranging from 385 to 396 °C was 15.14% (cal. 15.80%), accounting for the loss of a cyanic acid molecule from the (HC3N3O3)2− group.
Birefringence
As shown in Fig. S4,† the measured retardation was approximately 1922 nm. Furthermore, the measured thickness was 9.86 μm. Accordingly, the derived birefringence was 0.194. In addition, the energy dependent refractive index curves in three polarization directions, x, y and z, were calculated by DFT and are presented in Fig. S5.† The calculated birefringence value was 0.239, which is in good agreement with the experimental one, indicating that our measurement is reliable. The large birefringence values could ensure that the crystal realizes phase-matching in the UV region as short as possible. Besides, it is noteworthy that the birefringence of RbNa(HC3N3O3)·2H2O is comparable to those of typical commercial birefringent materials including CaCO3 (0.172@589 nm)34 and YVO4 (0.226@590 nm),35 and it is larger than those of the reported UV birefringent materials such as Ba2Ca(B3O6)2 (0.124@589 nm),36 Na3Ba2(B3O6)2F (0.115@532 nm),37 Ca3(BO3)2 (0.097@589 nm),38 BBO (0.119@546 nm)39 and Ca(BO2)2 (0.2471@193 nm).40
UV-Vis diffuse reflectance spectra
The UV-Vis diffuse reflectance spectra are shown in Fig. S6.† Obviously, the title compound exhibited wide transparency windows, >95%, from 266 to 800 nm, indicating that it could generate coherent light above 266 nm through direct SHG without absorption loss. Additionally, according to the Kubelka–Munk function, the optical band gap of RbNa(HC3N3O3)·2H2O can be deduced as 5.10 eV.
NLO properties
As shown in Fig. 4, the second-harmonic signals of RbNa(HC3N3O3)·2H2O were found to be about 5.3 times those of KDP at 1064 nm and 0.9 times those of BBO at 532 nm. Considering that the d36 coefficient for KDP is 0.39 pm V−1,41 the derived deff coefficient for RbNa(HC3N3O3)·2H2O is 2.07 pm V−1. In addition, since RbNa(HC3N3O3)·2H2O is isostructural to MLi(HC3N3O3)·2H2O (M = K, Rb), the SHG measurements on MLi(HC3N3O3)·2H2O (M = K, Rb) were also performed under the same conditions as with RbNa(HC3N3O3)·2H2O. It is clear that the SHG signal of RbNa(HC3N3O3)·2H2O is larger than those of the isostructural MLi(HC3N3O3)·2H2O (M = K, Rb). Therefore, it is interesting to investigate the SHG difference among these isostructural compounds.
According to the anionic group theory,42–44 the macroscopic SHG coefficients for these title compounds should originate from a geometrical addition of the microscopic second-order susceptibility of the planar (HC3N3O3)2− group. For these compounds, although only one crystallographically independent (HC3N3O3)2− group exists in the unit cell, it is clear that there are four kinds of (HC3N3O3)2− groups presenting different orientations due to the n slip plane and a slip plane of the space group. Because the four kinds of (HC3N3O3)2− groups exhibit opposite orientation in the a axis and b axis (see Fig. S2†), respectively, the contribution of NLO susceptibility mainly originates from the polarization of the (HC3N3O3)2− group along the c axis. It is noteworthy that though (HC3N3O3)2− groups present different orientations, they form the same angles θ31 and θ32 along the c axis (see Fig. 3). Since the (HC3N3O3)2− groups are approximately parallel to the a–c plane, we can define angle θ32, reflecting the level of the (HC3N3O3)2− groups parallel to the c axis, as the rotational angles θ. The rotational angles θ of KLi(HC3N3O3)·2H2O, RbLi(HC3N3O3)·2H2O and RbNa(HC3N3O3)·2H2O are 72.34°, 70.57° and 75.45°, respectively.
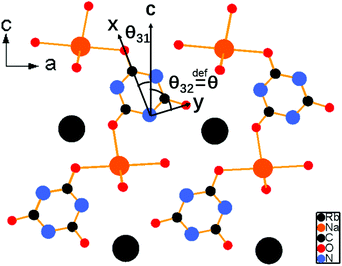 |
| Fig. 3 Rotational angles θ = θ32 of RbNa(HC3N3O3)·2H2O. | |
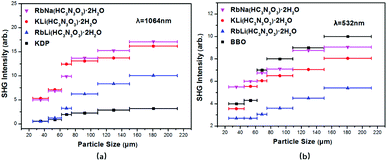 |
| Fig. 4 (a) SHG measurements of ground crystals of the title compounds with KDP as the reference at 1064 nm wavelength. (b) SHG measurements of ground crystals of the title compounds with BBO as the reference at 532 nm wavelength. | |
Further structural analysis of these crystals revealed that the different alignments among the (HC3N3O3)2− groups originated from the coordination behaviors of the counter cations, which can be distinguished by the ratios of ionic radii between the A+ (K+, Rb+) and B+ (Li+, Na+) cations. With the ratio ranging from 2.58 (Rb+/Li+) to 1.52 (Rb+/Na+), the rotational angle of (HC3N3O3)2− groups changed from 70.57° to 75.45°. These different arrangements resulted in different SHG effects due to the structural modulation of the anion groups. For the smallest ratios of ionic radii among the title compounds, all (HC3N3O3)2− groups in RbNa(HC3N3O3)·2H2O were parallel to each other, which contributed to the biggest SHG coefficient. These structures agreed well with the powder SHG experiment (see Table 2), which will be discussed in detail in a later section.
Table 2 NLO effects of RbLi(HC3N3O3)·2H2O, KLi(HC3N3O3)·2H2O and RbNa(HC3N3O3)·2H2O
Crystals |
Radius ratio of cations (A+/B+) |
Rotational angles (θ32) of (HC3N3O3)2− (deg.) |
Structural criterion C |
Densities of the (HC3N3O3)2− (N/V) (A−3) |
(N/V) × C (A−3) |
SHG coefficient (×KDP) |
RbLi(HC3N3O3)·2H2O (2) |
2.58 |
70.57 |
0.373 |
0.00533 |
0.00199 |
3.2 |
KLi(HC3N3O3)·2H2O (1) |
2.34 |
72.34 |
0.469 |
0.00558 |
0.00262 |
5.1 |
RbNa(HC3N3O3)·2H2O (3) |
1.52 |
75.05 |
0.569 |
0.00488 |
0.00277 |
5.3 |
Relationship between the structure and NLO properties
In order to investigate the relationship between the NLO properties and structure, the calculation methods reported in ref. 35 have been employed, which are described in detail in the ESI.†
Based on our previous studies,45–48 one can know that the overall nonlinear coefficients of isomorphic compounds are highly dependent on the structural criterion C. Because the C factor was determined by the arrangement of the anionic group, one can establish a relationship between the structural criterion C and the rotational angle θ. In these examples, the arrangement of (HC3N3O3)2− groups gave the greatest contribution to g333 (see Table 3), which revealed that the macroscopic SHG coefficient of the title compounds was mainly from the contribution of g333. Furthermore, we calculated the structural criterion C by the following equation:
Table 3 Geometrical factors (g) of the title compounds
Crystals |
g
311/N |
g
322/N |
g
333/N |
1
|
0.353 |
0.112 |
−0.469
|
2
|
0.264 |
0.112 |
−0.373
|
3
|
0.453 |
0.123 |
−0.569
|
Further structural analysis revealed the key role of cations in modulating the arrangement of the (HC3N3O3)2− groups, which was strongly related to the cation size and variable coordination environment of the cations. According to the coordination environment, hexa-coordinated atoms K+ and Rb+ were classified into A+ type while Li+ and Na+ were classified into B+ type. With the decrease in the ratios of ionic radii between the A+ (K+, Rb+) and B+ (Li+, Na+) cations, the rotational angles of 2, 1 and 3 (70.57°, 72.34°, and 75.05°, respectively) increased gradually. The structural criterion C of 2, 1 and 3 (0.373, 0.469, and 0.569, respectively) increased gradually with the changes of the rotational angles. Considering the structural factor (C) and the density of the (HC3N3O3)2− group (N/V), the macroscopic SHG effect of 2 was the smallest among these three NLO crystals. Although 3 had the smallest density, the macroscopic SHG effect was still the largest due to its largest rotational angles. The relationship between the structure and NLO properties was in good agreement with the PSHG measurements (shown in Table 2).
Theoretical calculations
The calculated band structure is presented in Fig. S7.† RbNa(HC3N3O3)·2H2O exhibited a direct band gap of 4.70 eV at the G point, which was underestimated compared with the experimental results of 5.10 eV due to the underestimation of the band gap with the DFT methods. Therefore, the scissor operator values of 0.11 eV were applied to the subsequent SHG calculations.
The total and partial densities of states (DOS and PDOS) for the title compound are plotted in Fig. S8.† Because the optical properties mainly originate from the electronic transition between the states close to the Fermi level, only the upper region of the valence band (VB) and the bottom region of the conduction band (CB) were presented. It is clear that the VB spreading from about −2.4 eV to the Fermi level was mostly contributions of the O 2p and N 2p orbitals and the CB between 5.1 and 5.8 eV was mainly due to the C 2p orbital. This calculated result indicated that the optical properties of the title compound are primarily due to the (HC3N3O3)2− groups.
The space group of RbNa(HC3N3O3)·2H2O belongs to class mm2; three independent SHG tensor components (d31, d32 and d33) should be taken into account. Theoretical values for the SHG coefficients were calculated using the “velocity-gauge” formula derived by Sipe et al. and are plotted in Fig. 5. The largest tensor component is d33 of 6.98 pm V−1 at a wavelength of 1064 nm (1.165 eV), which agreed well with the geometrical factor calculated by the anionic group theory. This further demonstrated that the (HC3N3O3)2− groups play a crucial role in the total SHG response.
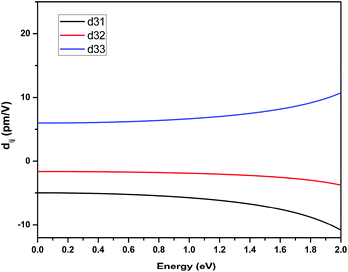 |
| Fig. 5 Calculated frequency-dependent SHG coefficient of RbNa(HC3N3O3)·2H2O. | |
Conclusions
A new mixed alkali isocyanurate, RbNa(HC3N3O3)·2H2O, has been successfully grown via a hydrothermal method. UV-Vis diffuse reflectance spectroscopy revealed that the title compound has a short UV cutoff edge of 243 nm. Additionally, linear and non-linear optical measurements showed that RbNa(HC3N3O3)·2H2O not only has a large birefringence of 0.194, but also a strong SHG effect that is 5.3 times that of KDP. These superior optical features suggest that RbNa(HC3N3O3)·2H2O may have promising applications in the UV region.
Conflicts of interest
There are no conflicts to declare.
Acknowledgements
This work was supported by the National Natural Science Foundation of China (Grant No 21975255, 51890862, U1605245, and 51425205), the National Key Research and Development Plan of Ministry of Science and Technology (Grant No 2016YFB0402104), the Strategic Priority Research Program of the Chinese Academy of Sciences (Grant No XDB20000000), the NSF of Fujian Province (2019J01020758) and the Youth Innovation Promotion Association CAS (303).
Notes and references
- M. Razeghi, Proc. IEEE, 2002, 90, 1006 CrossRef CAS.
- V. Petrov, Prog. Quantum Electron., 2015, 42, 1 CrossRef.
- Y. N. Xia, C. T. Chen, D. Y. Tang and B. C. Wu, Adv. Mater., 1995, 7, 79 CrossRef CAS.
- P. Becker, Adv. Mater., 1998, 10, 979 CrossRef CAS.
- X. L. Wang, L. K. Chen, W. Li, H. L. Huang, C. Liu, C. Chen, Y. H. Luo, Z. E. Su, D. Wu, Z. D. Li, H. Lu, Y. Hu, X. Jiang, C. Z. Peng, L. Li, N. L. Liu, Y. A. Chen, C. Y. Lu and J. W. Pan, Phys. Rev. Lett., 2016, 117, 210502 CrossRef.
- X. Dong, L. Huang, C. Hu, H. Zeng, Z. Lin, X. Wang, K. M. Ok and G. Zou, Angew. Chem., 2019, 58, 6528–6534 CrossRef CAS.
- G. H. Zou, C. S. Lin, H. Jo, G. Nam, T. S. You and K. M. Ok, Angew. Chem., 2016, 128, 12257 CrossRef.
- C. T. Chen, B. C. Wu, A. D. Jiang and G. M. You, Sci. China, Ser. B: Chem., Life Sci., Earth Sci., 1985, 28, 235 Search PubMed.
- S. Wang, N. Ye, W. Li and D. Zhao, J. Am. Chem. Soc., 2010, 132, 8779 CrossRef CAS.
- S. Zhao, J. Zhang, S. Q. Zhang, Z. Sun, Z. Lin, Y. Wu, M. Hong and J. Luo, Inorg. Chem., 2014, 53, 2521 CrossRef CAS.
- H. Yu, H. Wu, S. Pan, Z. Yang, X. Hou, X. Su, Q. Jing, K. R. Poeppelmeier and J. M. Rondinelli, J. Am. Chem. Soc., 2014, 136, 1264 CrossRef CAS.
- G. Cakmak, J. Nuss and M. Jansen, Z. Anorg. Allg. Chem., 2009, 635, 631 CrossRef CAS.
- X. Wang, Y. Wang, B. Zhang, F. Zhang, Z. Yang and S. Pan, Angew. Chem., Int. Ed., 2017, 56, 14119–14123 CrossRef CAS.
- Z. Fang, X. Jiang, M. Duan, Z. Hou, C. Tang, M. Xia, L. Liu, Z. Lin, F. Fan, L. Bai and C. Chen, Chem. – Eur. J., 2018, 24, 7856–7860 CrossRef CAS.
- M. Kalmutzki, M. Strobele, F. Wackenhut, A. J. Meixner and H. J. Meyer, Angew. Chem., Int. Ed., 2014, 53, 14260 CrossRef CAS.
- M. Kalmutzki, M. Strobele, F. Wackenhut, A. J. Meixner and H. J. Meyer, Inorg. Chem., 2014, 53, 12540 CrossRef CAS.
- F. Liang, L. Kang, X. Zhang, M.-H. Lee, Z. Lin and Y. Wu, Cryst. Growth Des., 2017, 17, 4015–4020 CrossRef CAS.
- D. Lin, M. Luo, C. Lin, F. Xu and N. Ye, J. Am. Chem. Soc., 2019, 141, 3390 CrossRef CAS.
- N. Wang, F. Liang, Y. Yang, S. Zhang and Z. Lin, Dalton Trans., 2019, 48, 2271–2274 RSC.
- X. Meng, F. Liang, J. Tang, K. Kang, W. Yin, T. Zeng, B. Kang, Z. Lin and M. Xia, Inorg. Chem. Front., 2019, 6, 2850–2854 RSC.
- X. Hao, M. Luo, C. Lin, D. Lin, L. Cao and N. Ye, Dalton Trans., 2019, 48, 12296–12302 RSC.
- X. Meng, F. Liang, K. Kang, J. Tang, Q. Huang, W. Yin, Z. Lin and M. Xia, Dalton Trans., 2019, 48, 9048–9052 RSC.
- X. Meng, F. Liang, K. Kang, J. Tang, T. Zeng, Z. Lin and M. Xia, Inorg. Chem., 2019, 58, 11289–11293 CrossRef CAS.
- X. Meng, F. Liang, J. Tang, K. Kang, Q. Huang, W. Yin, Z. Lin and M. Xia, Eur. J. Inorg. Chem., 2019, 2019, 2791 CrossRef CAS.
- G. M. Sheldrick, Acta Crystallogr., Sect. A: Found. Crystallogr., 2008, 64, 112–122 CrossRef CAS.
- A. L. Spek, J. Appl. Crystallogr., 2003, 36, 7–13 CrossRef CAS.
- J. Tauc, Mater. Res. Bull., 1970, 5, 721–729 CrossRef CAS.
- P. Kubelka and F. Munk, Z. Tech. Phys., 1931, 12(1), 886–892 Search PubMed.
- S. K. Kurtz and T. T. Perry, J. Appl. Phys., 1968, 39, 3798–3813 CrossRef CAS.
- S. J. Clark, M. D. Segall, C. J. Pickard, P. J. Hasnip, M. J. Probert, K. Refson and M. C. Payne, Z. Kristallogr., 2005, 220, 567–570 CAS.
- J. P. Perdew, K. Burke and M. Ernzerhof, Phys. Rev. Lett., 1996, 77, 3865–3868 CrossRef CAS PubMed.
- H. J. Monkhorst and J. D. Pack, Phys. Rev. B: Solid State, 1976, 13, 5188–5192 CrossRef.
- R. W. Boyd, Nonlinear Opt., 1992, 21 Search PubMed.
- G. Ghosh, Opt. Commun., 1999, 163, 95–102 CrossRef CAS.
- L. G. DeShazer, Proc. SPIE, 2002, 10, 4481 Search PubMed.
- Z. Jia, N. Zhang, Y. Ma, L. Zhao, M. Xia and R. Li, Cryst. Growth Des., 2017, 17, 558–562 CrossRef CAS.
- X. Wang, M. Xia and R. K. Li, Opt. Mater., 2014, 38, 6–9 CrossRef CAS.
- S. Zhang, X. Wu, Y. Song, D. Ni, B. Hu and T. Zhou, J. Cryst. Growth, 2003, 252, 246–250 CrossRef CAS.
- R. Appel, C. D. Dyer and J. N. Lockwood, Appl. Opt., 2002, 41, 2470–2480 CrossRef CAS.
- X. Chen, B. Zhang, F. Zhang, Y. Wang, M. Zhang, Z. Yang, K. R. Poeppelmeier and S. Pan, J. Am. Chem. Soc., 2018, 140, 16311–16319 CrossRef CAS.
- R. C. Eckardt, H. Masuda, Y. X. Fan and R. L. Byer, IEEE J. Quantum Electron., 1990, 26, 922–933 CrossRef CAS.
- N. Ye, Q. X. Chen, B. C. Wu and C. T. Chen, J. Appl. Phys., 1998, 84, 555–558 CrossRef CAS.
- C. T. Chen, Y. C. Wu and R. K. Li, Int. Rev. Phys. Chem., 1989, 8, 65–91 Search PubMed.
- C. Chen, Sci. Sin., 1979, 22, 756–776 Search PubMed.
- Y. Song, M. Luo, C. Lin and N. Ye, Chem. Mater., 2017, 29, 896–903 CrossRef CAS.
- G. Yang, G. Peng, N. Ye, J. Wang, M. Luo, T. Yan and Y. Zhou, Chem. Mater., 2015, 27, 7520–7530 CrossRef CAS.
- M. Luo, F. Liang, Y. Song, D. Zhao, F. Xu, N. Ye and Z. Lin, J. Am. Chem. Soc., 2018, 140, 3884 CrossRef CAS.
- G. Zou, N. Ye, L. Huang and X. Lin, J. Am. Chem. Soc., 2011, 133, 20001 CrossRef CAS.
Footnote |
† Electronic supplementary information (ESI) available. CCDC 1953902. For ESI and crystallographic data in CIF or other electronic format see DOI: 10.1039/c9qi01198f |
|
This journal is © the Partner Organisations 2020 |