DOI:
10.1039/C9OB02471A
(Review Article)
Org. Biomol. Chem., 2020,
18, 180-190
The reticent tautomer: exploiting the interesting multisite and multitype reactivity of 4-pyrrolin-2-ones
Received
15th November 2019
, Accepted 29th November 2019
First published on 30th November 2019
Abstract
4-Pyrrolin-2-ones are a lesser known tautomeric relative of the 3-pyrrolin-2-ones. Despite their infrequent appearance in the literature, they are very interesting and useful compounds. They have highly controllable, multisite and multitype reactivities which are covered in this review. The applications of these transformations show how the 4-pyrrolin-2-ones make excellent intermediates en-route to a range of key alkaloids. Innovative, fast and adaptable syntheses of the 4-pyrrolin-2-ones and their onward use via cascade reaction sequences are also presented to complete the case for commending these compounds as highly versatile and valuable synthetic building blocks.
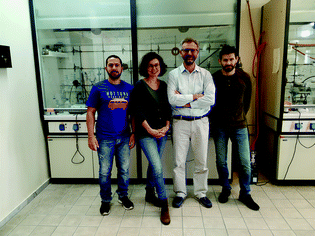 From left to right in the photo: Manolis Sofiadis, Tamsyn Montagnon, Georgios Vassilikogiannakis and Dimitris Kalaitzakis | Georgios Vassilikogiannakis obtained his Ph.D. (1998) with Prof. Orfanopoulos from the University of Crete. From 1999–2002, he was a postdoctoral fellow at the Scripps Research Institute with Prof. Nicolaou. His independent career started (2002) at the University of Crete, where he was promoted to full Professor (2013). He is a member of the advisory boards of OBC and EurJOC and the editorial board of ChemPhotoChem. He is the recipient of ERC starting and PoC grants. Tamsyn Montagnon obtained her Ph.D. (2000) with Prof. Parsons from the University of Sussex. From 2001–2004, she was a postdoctoral fellow at the Scripps Research Institute with Prof. Nicolaou where she co-authored the book “Molecules That Changed the World”. She is currently working as a Researcher at the University of Crete. Dimitris Kalaitzakis obtained his Ph.D. (2006) with Prof. Smonou from the University of Crete. In 2011 he joined the group of Prof. Vassilikogiannakis as a postdoctoral fellow. He is currently working as a Researcher at the University of Crete. Manolis Sofiadis obtained his M.Sc. (2016) with Prof. Vassilikogiannakis from the University of Crete. He is currently in the final year of his Ph.D. studies in the same research group. The research interests of the authors are in the field of sustainable synthetic organic chemistry with a particular focus on the development and application of new singlet oxygen-based synthetic methodologies. |
Introduction
We have two objectives for this review. At first they might seem distinct and separate, but we will argue that there is an intrinsic connection between the two which relates to the opportunities that present themselves when new flexible reactive intermediates are identified. Firstly, we want to highlight the unique versatility of the under-investigated 4-pyrrolin-2-ones 2. And, secondly, through our work on the multisite reactivity of these compounds, we want to illustrate some of the key principles behind the successful design of cascade reaction sequences because such sequences can be really powerful tools in the drive towards more efficient and greener chemistry.
Beginning with the latter of these two objectives, the advantages that come from including tandem back-to-back reactions in a synthetic strategy have long been appreciated.1,2 From the purest forms of domino reaction3 right through to telescoped reaction sequences,4 their use confers enhanced efficiencies across a number of different metrics. For instance; the innate step-economy5 of these sequences allows for rapid and dramatic increases in molecular complexity to be realised via simple one pot procedures.1b In addition, waste is minimised; for example, due to the elimination of intermediate purification steps. As such, tandem sequences are highly compliant tools in the contemporary quest for ever more sustainable6 and ideal syntheses.7 However, knowing the many advantages of tandem sequences does not facilitate their conception and design which are altogether more complex matters requiring access to multiple reaction pathways and a finely honed sense of both relative reactivity and reaction condition parameters.
Our group has spent the last two decades developing tandem one-pot sequences targeting a broad range of bioactive molecules and motifs;8 and, for us, the key ingredient to success has always been the triggered generation and subsequent manipulation of a relatively unstable intermediate bearing multiple potential sites for reaction. Using this approach we have been able to develop many cascade sequences in which one reaction follows on seamlessly from the previous one with ease.8 This strategy also proved to be highly adept at minimising non-strategic redox reactions9 and functional group interconversions,7 as well as, in avoiding protecting group use10 within syntheses of diverse oxygen-rich bioactive targets that would normally see a plethora of such non-productive steps. For a long time, the intermediate of choice for us was the enedione motif C obtained from furan photooxygenation (Scheme 1).8 Using this intermediate, however, had limitations because, despite having four contiguous reaction sites available, these sites are too similar and all electrophilic meaning that the reactions that could be applied were somewhat restricted and regiochemistry frequently had to be governed by deliberate proximal placement of an intramolecular nucleophile.8
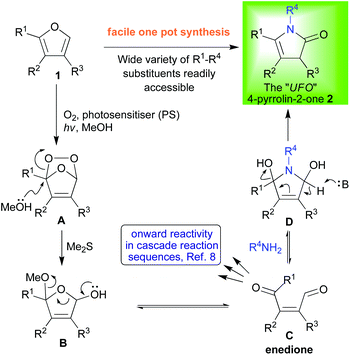 |
| Scheme 1 Synthesis of a versatile new intermediate, 4-pyrrolin-2-one 2. | |
In 2012, we unveiled a new and general route to an altogether more satisfying intermediate (1 → 2, Scheme 1).11 In the beginning, it was known within our group as the “UFO” because it was fragile and elusive, appearing only as a very UV active “unidentified flying object” on the tlc plates used to follow the progress of our reactions. Fortunately, we were quickly able to isolate and identify it as being a 4-pyrrolin-2-one 2. The synthetic potency of this reticent tautomer (vide infra) emanates both from the ease with which R1–R4 can be varied at will and from the differential reactivity which each of the core framework's four carbons exhibits (2, C2–5, Scheme 2). In this mini-review, we will take an expedited tour around this framework and examine its reactions in an effort to illustrate just how versatile this oft overlooked intermediate can be, but before we can set out on this journey some complex familial relationships need to be untangled.
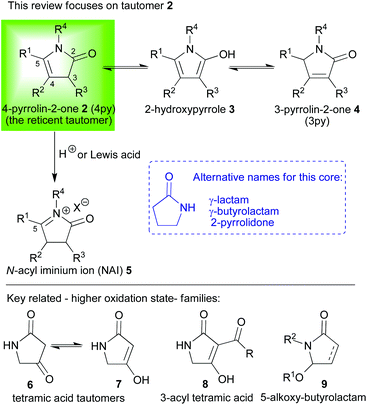 |
| Scheme 2 Compound names and family relationships that are important to this review. | |
To provide context for this review of recent work; our reticent tautomer 2 is one of the three tautomers shown in Scheme 2 (2, 3 and 4) and it is reticent because it lives in the shadow of its far more stable sibling, the 3-pyrrolin-2-one 4.12 This scheme also introduces some of the alternate nomenclature frequently employed when discussing these compounds (e.g. γ-lactams and γ-butyrolactams which both describe a larger set of pyrrolidone compounds). The pyrrolin-2-one family has for many years attracted background attention due to its proliferation in natural products and bioactive synthetic targets.12 Indeed, the ubiquity of its members further expands if one includes their higher oxidation state cousins; such as the tetramic acids13 or the 5-alkoxy butyrolactams14 (6–9, Scheme 2), which also commonly appear as bioactive synthetic targets. However, the lion's share of all the work published concerning this family has been focused around the 3-pyrrolin-2-one 4 tautomer;12 and this is despite the fact that the 4-pyrrolin-2-one 2 tautomer is frequently an intermediate in its reactions.15,16b Some investigations have even gone so far as to conclude that the 4-pyrrolin-2-one 2 tautomer could not be isolated in a pure form or at all.16 This misnomer has been disproved and our own experience is that if care is taken to avoid acidic conditions (for example, when conducting purifications, drops of NEt3 should be added to the eluent for column chromatographic separations), 4-pyrrolin-2-ones 2 can not only be isolated, but can be readily handled and manipulated.
Above and beyond the enhanced stability exhibited by the 3-pyrrolin-2-ones 4 over the 4-pyrrolin-2-ones 2, a paucity of truly general methods for the latter's direct synthesis has also restricted its appearance in synthetic methodologies. We had the opportunity to redress the balance and investigate the chemistry of this tautomer more thoroughly after we developed a unique and general synthesis of 4-pyrrolin-2-ones (2, Scheme 1).11 Our work has illustrated how a diverse array of 4-pyrrolin-2-ones 2 can be readily accessed via the photoxygenation of simple furans 1 whose substitution patterns, which directly translate into substitutions of the 4-pyrrolin-2-ones 2 products, are easy to manipulate (R1–R3, Scheme 1). Variation at the nitrogen (R4, Scheme 1) was also extremely facile with this new synthesis. The flexibility of this new method for synthesis of 4-pyrrolin-2-ones 2 is crucial to both the generality and utility of all the new chemistry subsequently elaborated. When thinking about where to start discussing the chemistry of 4-pyrrolin-2-ones 2 there is one obvious place to begin because one reaction type, the Pictet–Spengler,17 has monopolised the field and so it is here that we shall begin our tour.
Reactions of 4-pyrrolin-2-ones
[1] Reaction of aromatic nucleophiles with NAI 5 (rxn at C-5)
In this Pictet–Spengler type reaction, an N-acyl iminium ion (5, NAI, Scheme 2)18 is generated in situ and is then subjected to nucleophilic attack by an electron rich aromatic moiety; it can be inter-15c,i,j,k,19a or intramolecular15a,d,f,g,h,19 (Tables 1 and 2). It is a good place to start not only because it is the most well-studied of the reactions under consideration, but also because the lines between the 3-pyrrolin-2-one 4 and the 4-pyrrolin-2-one 2 tautomers are most blurred here with both being used as the starting substrate. When starting from the 3-pyrrolin-2-one 4 it has been transformed in situ to the 4-pyrrolin-2-one 2 by using strongly acidic reaction conditions15a,d,f–k or metal catalysis.15c,f The catalysis is frequently, but not always, accompanied by heating of the reaction mixture. Obviously, some of these methods are milder, and, therefore more broadly applicable, than others; however, the main advantage in using this transformation which applies across almost all of the exemplars shown in Tables 1 and 2, is the way it allows for the rapid construction of complex polycyclic alkaloid frameworks. Indeed, it has proved to be an excellent route into syntheses of both the erythrina alkaloids19e (entry 4, Table 2) and a variety of indole alkaloid frameworks15c,e,g,19c,d,f,20 (Table 1 and entries 3, 5 and 6, Table 2), both of which represent a highly important class of bioactive targets. This ability to rapidly construct polycyclic frameworks is further enhanced when a one pot operation is employed to synthesise the pyrrolin-2-one substrate and then react it through its corresponding NAI in a Pictet–Spengler-type reaction15c,d,f,g,19a,c–f,20 (entries 1 and 2, Table 1 and 3–6, Table 2). Furthermore, the most general and useful one pot cascade reaction sequences are those wherein the starting substrate is also easily synthesised with readily accessible variations in its substitution patterns15g,19a,c,d,f (entry 1, Table 1 and entries 3 and 6, Table 2). Finally, a recent important development in this field was achieved when some such reaction sequences were conducted on a large scale (with very high conversions and productivities ranging from 0.23 to 0.41 mmol min−1) using a prototype continuous flow reactor19a (entry 1, Table 1). In this nebulizer driven apparatus, the initial furan photooxygenation occurred in an aerosol which had excellent light penetration properties and large gas–liquid interface areas; thus, addressing many of the inherent problems associated with scaling up photochemistry and/or biphasic reactions and maximising effectiveness. Upon condensation of the aerosol in a collecting vessel, all subsequent steps in the sequence were rapidly completed to furnish a range of interesting products (10 and 21).
Table 1 Intermolecular reactions of the pyrrolin-2-ones with aromatic nucleophiles
Table 2 Intramolecular Pictet–Spengler type reactions of the pyrrolin-2-ones
[2] Other reactions of the pyrrolin-2-ones occurring via the N-acyliminium ion (rxn at C-5)
In the previous section we saw how aromatic moieties can be induced to react with pyrrolin-2-ones via the NAI 5 which is formed in situ. Other nucleophiles, both carbon-15b,19d or heteroatom15e,19b–d,21 -centred, can also react with these intermediate NAIs 5.
In our first set of first examples, a pendent hydroxyl group can readily react to form very useful Meyers’(-type) bicyclic lactams22 (32
11,15f or 34,21Scheme 3) when it has been incorporated into the 4-pyrrolin-2-one 2 as part of the substitution on the nitrogen (1e→ [2k→5k] →32 a very general one pot TFA-catalysed cyclisation; or, 33→2k→ [5k] →34 a more specific BF3·OEt2-catalysed cyclisation).
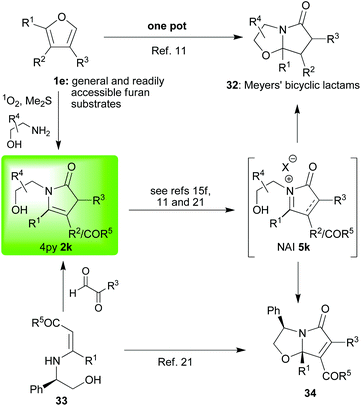 |
| Scheme 3 Synthesis of Meyers’-type bicyclic lactams via 4-pyrrolin-2-ones. | |
The examples in Scheme 4 primarily show two things; firstly, they illustrate the wide range of nucleophiles that can be employed to react with NAIs of type 5,15b,e,19a,c,d but they also reiterate the generality of the one pot method beginning from simple and readily accessible furans.19a,c,d Also of note here are the observations19c,d revealing that, when a choice of two possible products exists (Pictet–Spengler type versus another nucleophile), careful choice of the reaction conditions to be employed (Lewis or Brønsted acid) can lead to delivery of either one of the two possible products exclusively (2m→35a or 2m→21a,bScheme 5); an element of control which is vital if applications in complex systems are to be attempted.
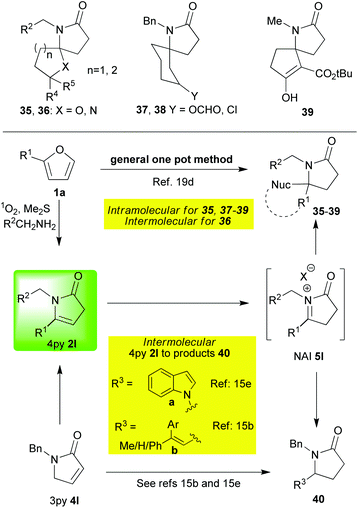 |
| Scheme 4 A wide range of nucleophiles react with NAIs 5 derived from 4pys 2. | |
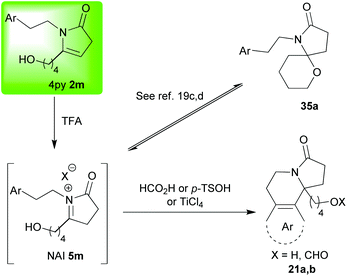 |
| Scheme 5 Tunability of the reaction of NAIs 5 with nucleophiles. | |
Overall and akin to the Pictet Spengler variants described in the previous section, the reactions of an NAI 5 with alternative nucleophiles (shown in Schemes 3–5) have proven to be powerful tools for the creation of polycyclic fused- or spiro- ring systems that are commonly found in synthetic targets.
[3] Redox chemistry of 4-pyrrolin-2-ones (oxidation state changes at C-3,4,5)
Stepping away from the popular NAI-related chemistry of the pyrrolin-2-ones, published investigations become much sparser, but it is fair to say that the allure of the work involved increases and the real versatility of the 4-pyrrolin-2-ones 2 starts to become more apparent. One of the most interesting reactions of the 4-pyrrolin-2-ones 2 that we discovered was a methylene blue catalysed proton-coupled-electron-transfer (PCET) oxidation which afforded either 5-hydroxy-1H-pyrrol-2(5H)-ones 41 or their dehydrated counterparts 42 (Scheme 6).23,24 This unusual and mild reaction (2n → 41), which was not light catalysed and took place in the dark, is presumed to follow a mechanism that has been summarised in Scheme 6. We proved that methylene blue was fulfilling a unique dual role in the full sequence (1f → 41); first as a photosensitiser (furan photooxidation using singlet oxygen, 1f → 2n) and then as a redox catalyst (4-pyrrolin-2-one oxidation using triplet oxygen, 2n → 41). It is of note that we propose that this reaction proceeds via the third fleeting tautomer, the 2-hydroxypyrrole 3, once again reminding us of the complex interconnectivity exhibited within this family of tautomers. We were able to develop this chemistry so that one pot protocols took us direct from a starting furan substrate to one of the products from a very interesting range (Scheme 7A–C),24 including, the natural products pandamarine 43,24d pandanusines A and B, as well as, pandalizines A–C24a,c and key motifs such as the pyrrolizidines 44,24c indolizidines 45
24c and 3-acyl-5-alkoxy-butyrolactams 9a.24b
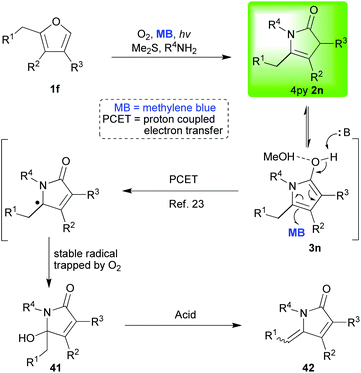 |
| Scheme 6 Methylene blue's dual role: oxidation of furans and 4-pyrrolin-2-ones. | |
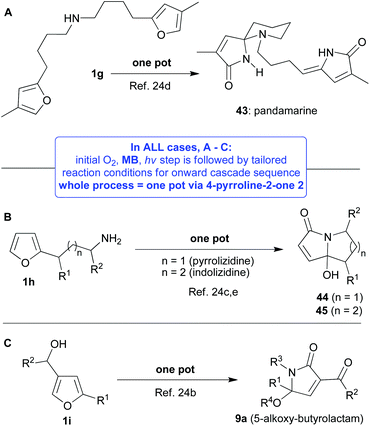 |
| Scheme 7 Applications of methylene blue dual role cascade reaction sequences. | |
It was during this phase of our investigations into the chemistry of the 4-pyrrolin-2-ones 2 that we were able to prove that basic nitrogen functionalities could be included in all the oxidative steps of these cascade sequences without protection (Scheme 7A and B).24c–e This advance was very fundamental and expanded the importance and utility of the work dramatically. The synthesis of pandamarine 43 (Scheme 7A) was particularly exciting not only because it was the first total synthesis reported for this natural product and it was only six steps in total, but also because the central cascade reaction sequence was a double one with two furan motifs undergoing oxidation in parallel and in the presence of an unprotected secondary amine.24d We were also able to elucidate some biosynthetic details during this investigation which had been unclear at the beginning.
The pyrrolizidine 44 and indolizidine 45 synthesis24c (Scheme 7B) was also deemed to be important as the fused azabicyclic izidine core makes up a significant percentage of all known natural alkaloids and its simple, general and effective construction has proven to be much more difficult to achieve than it might first appear on paper. And, finally in this section our smooth one pot synthesis of 3-acyl-5-alkoxy-butyrolactams 9a (Scheme 7C) opened the door to new methodologies focusing on the many further oxidised relatives of the 4-pyrrolin-2-ones 2 that commonly appear in nature and as bioactive targets for synthesis.
While our group has focused on elaborating the 4-pyrrolin-2-one skeleton 2 using oxidative methods –vide supra– others have searched for reliable ways to enantioselectively reduce this core's motif; thus, providing access to key saturated lactams which are also ubiquitous targets (Scheme 8).25 Hydrogen combined with either iridium (2o → 46)25a or rhodium (2o → 47)25b and a chiral ligand have proved successful. The latter, however, is restricted to substrates bearing an electron withdrawing group at C-4.
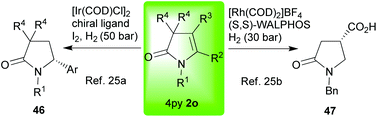 |
| Scheme 8 Enantioselective reduction of 4-pyrrolin-2-ones. | |
[4] Formal [3 + 3]-annulations of 4-pyrrolin-2-ones (rxn at C-4 & 5)
The octahydroindole skeleton (OHI skeleton, 53, Scheme 10) is another framework that is prevalent amongst bioactive alkaloid natural products; so finding new and effective methods for its synthesis makes for a valuable endeavour. To this end, one way of looking at the 4-pyrrolin-2-one framework 2 is to see a relatively electron-rich dienophile primed to participate in an inverse electron demand Diels–Alder reaction. With this idea in mind, we started to work on developing a new reaction sequence wherein the 4-pyrrolin-2-one 2, derived from a suitably substituted furan 1jvia our, by now, well-established procedure, would be partnered with an α,β-unsaturated carbonyl compound 48 in a hetero-Diels–Alder reaction (Scheme 9).26 During the investigation, a number of different traits were observed for this first mild hetero-Diels Alder step which led us to conclude that it was indeed a concerted [4 + 2]-cycloaddition. Despite the potential utility of the intermediate tetrahydropyranopyrrolone 49, we chose not to stop here, but to employ acid catalysis to orchestrate the reopening of the dihydropyran unit (49 → 50); an operation that would be followed immediately by N-acyl enamine addition to the carbonyl to form, after dehydration, the unsaturated six membered carbocycle 51. Overall, the sequence may be described as formally yielding the [3 + 3]-annulation products. We were able to successfully develop this advanced protecting group-free sequence in order to obtain an attractive range of related products with impressive economies (primarily step-, but also atom-, waste- etc.).26 Furthermore, the final stages of this sequence could be tailored in accordance with the product desired. Indeed, even a reduction step mediated by a BF3·OEt2/Et3SiH or TFA/Et3SiH combination could be tagged onto the end to yield the saturated OHI skeleton (53, Scheme 10). As such, this investigation served to exemplify the degree to which cascade complexity can be pushed if both the design and implementation are undertaken with care; for instance, the impressive transformation of 1j into 53 was achieved through what was in essence an operationally very simple one pot procedure.
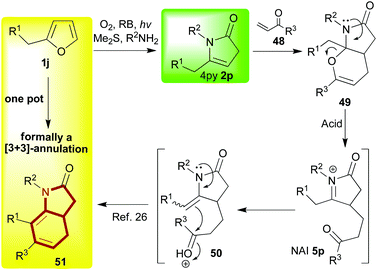 |
| Scheme 9 Rapid access to unsaturated product 51. | |
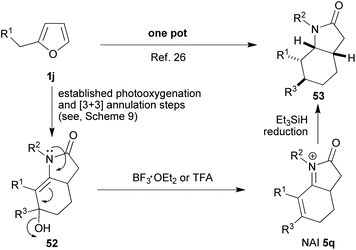 |
| Scheme 10 One pot access to the saturated octahydroindole (OHI) skeleton. | |
Another application for this [3 + 3]-annulation chemistry was then developed in a project which also drew on the power that methylene blue has to affect further oxidations of 4-pyrrolidinones 2 in the dark with molecular oxygen acting as the terminal oxidant (see, section 3 text and Scheme 6).23 The structural units successfully targeted in this new work27 were 2-oxindoles, isatins and 3-hydroxy-2-oxindoles; once again these are motifs which often appear in alkaloids –in this case aromatic ones- that are considered to be important due to their biological activities (Scheme 11).
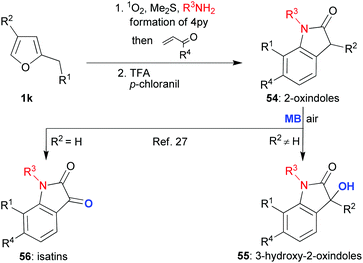 |
| Scheme 11 Combining the [3 + 3]-annulation and MB dual activity methodologies to target a range of aromatic alkaloids. | |
An intramolecular aza-Paternò–Büchi reaction, occurring between specific 4-pyrrolin-2-one 2 moieties (at C-4 and C-5) and a pendent imine, which affords unique fused polycyclic motifs, has also been reported.28
[5] Organocatalysed reactions of 4-pyrrolin-2-ones (rxns at C5/N & C3/C4)
With a large body of work behind us, we were intrigued to see if we could answer some of the remaining challenges in 4-pyrrolin-2-one 2 chemistry. Firstly, we wanted to know whether we could use organocatalysis to access various enantioenriched alkaloid skeletons, and, secondly, we wanted to know whether we could controllably substitute at C-3; a position whose potential reactivity was severely underexplored given that the major focus had always been on the C-4 and C-5 positions (vide supra). We also intended to look at substituting at the nitrogen so that, overall, this investigation would show selective nucleophilicity at four out of the five positions on the 4-pyrrolin-2-one 2 ring; thus, reinforcing the idea that 4-pyrrolin-2-ones 2 show truly remarkable and controllable multisite reactivity and versatility. Our idea was to employ LUMO-lowering organocatalysis in the [3 + 2]-annulations of the 4-pyrrolin-2-ones 2r with α,β-unsaturated aldehydes 57 (Scheme 12) in a transformation that could selectively generate up to four new stereocentres.29 The enantioenriched bicyclic lactam products that we would be synthesising lack general and effective syntheses, and, furthermore, they could serve as very useful intermediates in the synthesis of a range of important asymmetric alkaloid targets.30 When these ideas were implemented29 a very interesting switch in mechanism was observed dependent on the degree of substitution of the lactam nitrogen (2r → 59 or 2r → 60, Scheme 13). A number of experimental observations supported the hypothesis given in this scheme. Thus, when the lactam nitrogen is not substituted (R = H, 2r → 60, Schemes 12 and 13) a vinylogous Michael reaction (reaction at C-5) occurs generating an optically pure quaternary stereocenter followed by hemiaminalisation. This latter step is reversible and reduction with sodium borohydride is readily achieved to afford hydroxyl-lactams 61 (Scheme 13). The products were obtained with excellent diastereomeric and enantiomeric excesses. It should be noted that a number of other groups have studied base catalysed additions to C-5 starting from the 3-pyrrolin-2-one 4 tautomer (for a recent example starting from a mixture of 3py and 4py see Scheme 14).31 Returning to our investigation, when the nitrogen was substituted (R ≠ H, 2r → 59, Schemes 12 and 13) a different reaction pathway is followed. In this case, the first step is a Michael reaction between the lactam (reaction at C-3) and the activated aldehyde 57. In the second step, enamide addition to the aldehyde functionality affords the final bicyclic lactam 59. This transformation required the addition of benzoic acid to proceed; it may be that this reagent accelerates the second H-bond directed cyclisation step in which the aldehyde is trapped by the enamide. Notably, the entire sequence 1j → 59 could be done in one pot without compromising the yield or diastereo-/enantioselectivity.
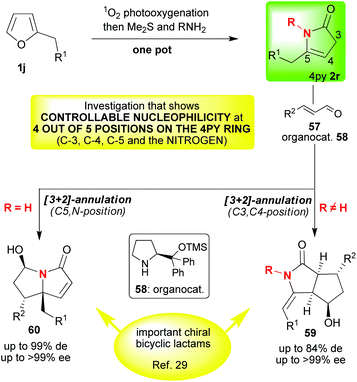 |
| Scheme 12 Synthesis of important enantioenriched bicyclic lactams from furans via 4-pyrrolin-2-ones. | |
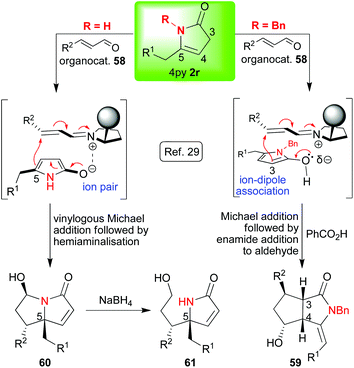 |
| Scheme 13 Mechanistic switch dependent on nitrogen substitution. | |
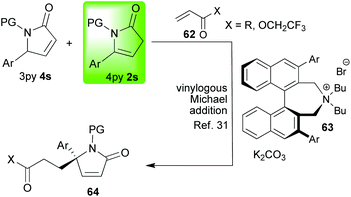 |
| Scheme 14 A recent example of base catalysed additions to C-5 starting from a mixture of 3py and 4py. | |
Mukherjee and co-workers have published their own elegantly conceived contributions for the organocatalysed functionalisation of the C-3 position of the 4-pyrrolin-2-ones of type 2t bearing an ester group at C-4.32 In an illustrative example,32b it was shown that the C-3 position could be substituted using Michael additions (2t → 66, Scheme 15). A number of different chiral amine organocatalysts were investigated with high diastereo- and enantioselectivities achieved.
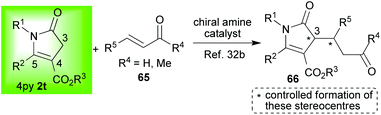 |
| Scheme 15 Organocatalysed substitution at C-3 of 4-pyrrolin-2-ones. | |
These examples29,32 represent the first time that efforts have focused on substitution at C-3, and, thus, they complete our tour around the 4-pyrrolin-2-one 2 framework which has shown that each of the contiguous centres (C-3 to C-5 and then on to the nitrogen – four contiguous centres in total) which make up the framework of the pyrrolidone can be manipulated and substituted, at will, by choosing one from a range of mild reactions. The reactivities at each position are exceptional in their complementarity such that regioselectivity has never been a problem. It is extremely rare to find a structural unit with so many contiguous centres that can all be controllably substituted as a result of their unique reactivity profile and it is this unusually strong flexibility that makes the 4-pyrrolin-2-one tautomer 2 so interesting and versatile.
Conclusions
In this article, we have been able to emphasise the remarkable breadth of reaction type for which the 4-pyrrolin-2-ones 2 can act as substrates. Scheme 16 has been added to illustrate the impressive diversity in the range of scaffolds that our group has been able to access using cascade reaction sequences starting from readily accessible furans and proceeding via these uniquely flexible 4-pyrrolin-2-ones 2. We have proved that we were not only able to make these intermediates via simple sustainable cascade sequences, but we could continue the onward reaction as part of the same one pot, highly efficient processes. We have also highlighted work from other groups that adds further to the motifs that can be easily attained from this versatile building block. Further, in conducting this survey, we hope that we have been able to present a convincing argument that the secret to 4-pyrrolin-2-ones's 2 versatility lies in exactly the two characteristics which also make them ideal intermediates for including in the designs for new cacscade reaction sequences; namely, perfectly balanced reactivity (just stable enough to handle and manipulate, yet perfectly poised to react under mild conditions) and possessed of exceptional differential reactivity at adjacent centres (highly tunable and selective chemistry). All this work attests to the value of this lesser known, reticent tautomer whose chemistry is so oft overlooked because of the dominance its sibling's (the 3-pyrrolin-2-one's 4) more mundane chemistry.
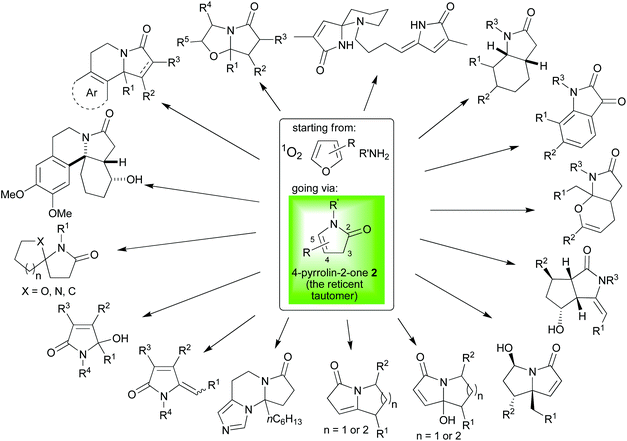 |
| Scheme 16 Selected motifs accessed by our group using cascade reaction sequences starting from readily accessible furans and proceeding via the key 4-pyrrolin-2-one 2 intermediate. | |
Conflicts of interest
There are no conflicts to declare.
Acknowledgements
The research leading to these results has received funding from the European Research Council under the European Union's Seventh Framework Programme (FP7/2007-2013)/ERC grant agreement no. 277588. We thank the Greek General Secretariat of Research and Technology for matching (reward) funds (KA: 4143 and 4154). We also thank the Alexander S. Onassis Public Benefit Foundation for the Ph.D. fellowship of Manolis Sofiadis (G ZM 063-1/2016-2017).
Notes and references
- For useful discussions of both the definitions of tandem reactions and their benefits, see;
(a) S. E. Denmark and A. Thorarensen, Chem. Rev., 1996, 96, 137–166 CrossRef CAS PubMed;
(b) Y. Hayashi, Chem. Sci., 2016, 7, 866–880 RSC.
- For applications of cascade sequences in synthesis, see; K. C. Nicolaou, T. Montagnon and S. A. Snyder, Chem. Commun., 2003, 551–564 RSC.
- L. F. Tietze, Chem. Rev., 1996, 96, 115–136 CrossRef CAS PubMed.
-
N. G. Anderson, Practical process research & development, Academic Press, San Diego, Calif.; London, 2000 Search PubMed.
- P. A. Wender and B. L. Miller, Nature, 2009, 460, 197–201 CrossRef CAS PubMed.
- C.-J. Li and B. M. Trost, Proc. Natl. Acad. Sci. U. S. A., 2008, 105, 13197–13202 CrossRef CAS PubMed.
-
(a) T. Newhouse, P. S. Baran and R. W. Hoffmann, Chem. Soc. Rev., 2009, 38, 3010–3021 RSC;
(b) T. Gaich and P. S. Baran, J. Org. Chem., 2010, 75, 4657–4673 CrossRef CAS PubMed.
-
(a) A. Kouridaki, M. Sofiadis, T. Montagnon and G. Vassilikogiannakis, Eur. J. Org. Chem., 2015, 7240–7243 CrossRef CAS;
(b) D. Kalaitzakis, M. Triantafyllakis, I. Alexopoulou, M. Sofiadis and G. Vassilikogiannakis, Angew. Chem., Int. Ed., 2014, 53, 13201–13205 CrossRef CAS PubMed;
(c) M. Triantafyllakis, M. Tofi, T. Montagnon, A. Kouridaki and G. Vassilikogiannakis, Org. Lett., 2014, 16, 3150–3153 CrossRef CAS PubMed;
(d) D. Noutsias, I. Alexopoulou, T. Montagnon and G. Vassilikogiannakis, Green Chem., 2012, 14, 601–604 RSC;
(e) D. Noutsias, A. Kouridaki and G. Vassilikogiannakis, Org. Lett., 2011, 13, 1166–1169 CrossRef CAS PubMed;
(f) M. Tofi, K. Koltsida and G. Vassilikogiannakis, Org. Lett., 2009, 11, 313–316 CrossRef CAS PubMed;
(g) M. Tofi, T. Montagnon, T. Georgiou and G. Vassilikogiannakis, Org. Biomol. Chem., 2007, 5, 772–777 RSC;
(h) T. Georgiou, M. Tofi, T. Montagnon and G. Vassilikogiannakis, Org. Lett., 2006, 8, 1945–1948 CrossRef CAS PubMed;
(i) N. Sofikiti, M. Tofi, T. Montagnon, G. Vassilikogiannakis and M. Stratakis, Org. Lett., 2005, 7, 2357–2359 CrossRef CAS PubMed. For reviews, see;
(j) T. Montagnon, D. Kalaitzakis, M. Triantafyllakis, M. Stratakis and G. Vassilikogiannakis, Chem. Commun., 2014, 50, 15480–15498 RSC;
(k) T. Montagnon, M. Tofi and G. Vassilikogiannakis, Acc. Chem. Res., 2008, 41, 1001–1011 CrossRef CAS PubMed.
- N. Z. Burns, P. S. Baran and R. W. Hoffmann, Angew. Chem., Int. Ed., 2009, 48, 2854–2867 CrossRef CAS PubMed.
- S. Young and P. S. Baran, Nat. Chem., 2009, 1, 193 CrossRef PubMed.
- D. Kalaitzakis, T. Montagnon, I. Alexopoulou and G. Vassilikogiannakis, Angew. Chem., Int. Ed., 2012, 51, 8868–8871 CrossRef CAS PubMed.
- For reviews, see;
(a) A. Y. Egorova and Z. Y. Timofeeva, Chem. Heterocycl. Compd., 2004, 40, 1243–1261 CrossRef CAS;
(b)
E. T. Pelkey, S. J. Pelkey and J. G. Greger, De Novo Synthesis of 3-Pyrrolin-2-Ones, Elsevier Ltd, 2015, vol. 115 Search PubMed.
-
(a) B. J. L. Royles, Chem. Rev., 1995, 95, 1981–2001 CrossRef CAS;
(b) X. Mo, Q. Li and J. Ju, RSC Adv., 2014, 4, 50566–50593 RSC.
- For a review, see; B. Nay, N. Riache and L. Evanno, Nat. Prod. Rep., 2009, 26, 1044–1062 RSC.
-
(a) K. Jebali, A. Planchat, H. Amri, M. Mathé-Allainmat and J. Lebreton, Synthesis, 2016, 48, 1502–1517 CrossRef CAS;
(b) M. Wang, B. Gao and H. Huang, Tetrahedron Lett., 2015, 56, 5533–5536 CrossRef CAS;
(c) M. T. Petersen and T. E. Nielsen, Org. Lett., 2013, 15, 1986–1989 CrossRef CAS PubMed;
(d) Q. Cai, X. W. Liang, S. G. Wang, J. W. Zhang, X. Zhang and S. L. You, Org. Lett., 2012, 14, 5022–5025 CrossRef CAS PubMed;
(e) Y. Xie, Y. Zhao, B. Qian, L. Yang, C. Xia and H. Huang, Angew. Chem., Int. Ed., 2011, 50, 5682–5686 CrossRef CAS PubMed;
(f) E. Ascic, J. F. Jensen and T. E. Nielsen, Angew. Chem., Int. Ed., 2011, 50, 5188–5191 CrossRef CAS PubMed;
(g) C. A. Holloway, M. E. Muratore, R. I. Storer and D. J. Dixon, Org. Lett., 2010, 12, 4720–4723 CrossRef CAS PubMed;
(h) J. Pérard-Viret, F. Souquet, M.-L. Manisse and J. Royer, Tetrahedron Lett., 2010, 51, 96–98 CrossRef;
(i) G. Wille and W. Steglich, Synthesis, 2001, 759–762 CrossRef CAS;
(j) V. Bocchi, G. Casnati and G. P. Gardini, Tetrahedron Lett., 1971, 683–684 CrossRef CAS;
(k) V. Bocchi and G. P. Gardini, Org. Prep. Proced. Int., 1969, 1, 271–277 CAS.
-
(a) J. C. Hubert, J. B. P. A. Wijnberg and W. N. Speckamp, Tetrahedron, 1975, 31, 1437–1441 CrossRef CAS;
(b) J. T. Baker and S. Sifniades, J. Org. Chem., 1979, 44, 2798–2800 CrossRef CAS;
(c) R. T. Watson, V. K. Gore, K. R. Chandupatla, R. K. Dieter and J. P. Snyder, J. Org. Chem., 2004, 69, 6105–6114 CrossRef CAS PubMed.
-
(a) E. D. Cox and J. M. Cook, Chem. Rev., 1995, 95, 1797–1842 CrossRef CAS;
(b) J. Stöckigt, A. P. Antonchick, F. Wu and H. Waldmann, Angew. Chem., Int. Ed., 2011, 50, 8538–8564 CrossRef PubMed.
-
(a) W. N. Speckamp and H. Hiemstra, Tetrahedron, 1985, 41, 4367–4416 CrossRef CAS;
(b) B. E. Maryanoff, H.-C. Zhang, J. H. Cohen, I. J. Turchi and C. A. Maryanoff, Chem. Rev., 2004, 104, 1431–1628 CrossRef CAS PubMed;
(c) P. Wu and T. E. Nielsen, Chem. Rev., 2017, 117, 7811–7856 CrossRef CAS PubMed.
-
(a) G. I. Ioannou, T. Montagnon, D. Kalaitzakis, S. A. Pergantis and G. Vassilikogiannakis, ChemPhotoChem, 2018, 2, 860–864 CrossRef CAS PubMed;
(b) G. I. Ioannou, D. Kalaitzakis and G. Vassilikogiannakis, Eur. J. Org. Chem., 2016, 3304–3306 CrossRef CAS;
(c) D. Kalaitzakis, T. Montagnon, G. I. Ioannou, E. Antonatou and G. Vassilikogiannakis, ARKIVOC, 2015,(iii), 154–166 Search PubMed;
(d) D. Kalaitzakis, E. Antonatou and G. Vassilikogiannakis, Chem. Commun., 2014, 50, 400–402 RSC;
(e) D. Kalaitzakis, T. Montagnon, E. Antonatou and G. Vassilikogiannakis, Org. Lett., 2013, 15, 3714–3717 CrossRef CAS PubMed;
(f) D. Kalaitzakis, T. Montagnon, E. Antonatou, N. Bardají and G. Vassilikogiannakis, Chem. – Eur. J., 2013, 19, 10119–10123 CrossRef CAS PubMed.
- M. E. Muratore, C. A. Holloway, A. W. Pilling, R. I. Storer, G. Trevitt and D. J. Dixon, J. Am. Chem. Soc., 2009, 131, 10796–10797 CrossRef CAS PubMed.
- C. Agami, A. Beauseigneur, S. Comesse and L. Dechoux, Tetrahedron Lett., 2003, 44, 7667–7669 CrossRef CAS.
- M. D. Groaning and A. I. Meyers, Tetrahedron, 2000, 56, 9843–9873 CrossRef CAS.
- D. Kalaitzakis, A. Kouridaki, D. Noutsias, T. Montagnon and G. Vassilikogiannakis, Angew. Chem., Int. Ed., 2015, 54, 6283–6287 CrossRef CAS PubMed.
-
(a) D. Kalaitzakis, K. Daskalakis, M. Triantafyllakis, M. Sofiadis and G. Vassilikogiannakis, Org. Lett., 2019, 21, 5467–5470 CrossRef CAS PubMed;
(b) M. Sofiadis, J. Sarris, T. Montagnon, D. Kalaitzakis and G. Vassilikogiannakis, Eur. J. Org. Chem., 2018, 4523–4526 CrossRef CAS;
(c) D. Kalaitzakis, M. Triantafyllakis, M. Sofiadis, D. Noutsias and G. Vassilikogiannakis, Angew. Chem., Int. Ed., 2016, 55, 4605–4609 CrossRef CAS PubMed;
(d) D. Kalaitzakis, D. Noutsias and G. Vassilikogiannakis, Org. Lett., 2015, 17, 3596–3599 CrossRef CAS PubMed;
(e) T. Montagnon, D. Kalaitzakis, M. Sofiadis and G. Vassilikogiannakis, Org. Biomol. Chem., 2016, 14, 8636–8640 RSC.
-
(a) Q. Yuan, D. Liu and W. Zhang, Org. Lett., 2017, 19, 1144–1147 CrossRef CAS PubMed;
(b) H. R. Campello, J. Parker, M. Perry, P. Ryberg and T. Gallagher, Org. Lett., 2016, 18, 4124–4127 CrossRef CAS PubMed.
- D. Kalaitzakis, M. Triantafyllakis, G. I. Ioannou and G. Vassilikogiannakis, Angew. Chem., Int. Ed., 2017, 56, 4020–4023 CrossRef CAS PubMed.
- M. Triantafyllakis, K. Sfakianaki, D. Kalaitzakis and G. Vassilikogiannakis, Org. Lett., 2018, 20, 3631–3634 CrossRef CAS PubMed.
- E. Kumarasamy, S. K. Kandappa, R. Raghunathan, S. Jockusch and J. Sivaguru, Angew. Chem., Int. Ed., 2017, 56, 7056–7061 CrossRef CAS PubMed.
- D. Kalaitzakis, M. Sofiadis, M. Triantafyllakis, K. Daskalakis and G. Vassilikogiannakis, Org. Lett., 2018, 20, 1146–1149 CrossRef CAS PubMed.
-
O. Jeger and V. Prelog, in The Alkaloids: Chemistry and Physiology, ed. R. H. Manske, Academic Press, Inc., New York, 1960, p. 319 Search PubMed.
- A. Arlt, H. Toyama, K. Takada, T. Hashimoto and K. Maruoka, Chem. Commun., 2017, 53, 4779–4782 RSC.
-
(a) B. Ray and S. Mukherjee, Tetrahedron, 2019, 75, 3292–3298 CrossRef CAS;
(b) S. J. Singha Roy and S. Mukherjee, J. Org. Chem., 2018, 83, 12071–12085 CrossRef CAS PubMed;
(c) S. J. Singha Roy and S. Mukherjee, Org. Biomol. Chem., 2017, 15, 6921–6925 RSC.
|
This journal is © The Royal Society of Chemistry 2020 |