DOI:
10.1039/C9NH00476A
(Review Article)
Nanoscale Horiz., 2020,
5, 218-234
Recent advances in carbon dots for bioimaging applications
Received
19th July 2019
, Accepted 8th October 2019
First published on 16th October 2019
Abstract
As an important member of fluorescence nanoparticles, carbon dots (CDs) not only possess exceptional chemical properties, including excellent biocompatibility, low toxicity and chemical inertness, but also exhibit promising optical properties, such as broad excitation spectra, tunable emission spectra and high photostability, thus attracting tremendous attention. Over the past decade, applications of CDs in numerous fields have been intensively investigated, ranging from biomedical analysis to optoelectronics and energy. Among these cutting-edge applications, this review article mainly outlines recent advances in the field of CD-based bioimaging and summarizes achievements in two broad categories: in vitro imaging in terms of the approaches to deliver CD-based probes into different types of cells and vital cell organelles, as well as in vivo applications that focus on distribution and uptake of CDs in mice and zebrafish, imaging-guided drug delivery and tumor therapeutics. Furthermore, the potential superiority, challenges and future directions of CDs for imaging are also presented. The purpose of this review is to highlight the importance of CD-based bioimaging and to boost its development in diverse research fields ranging from materials science to biomedicine.
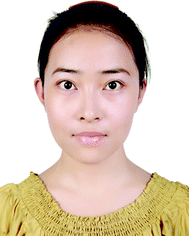
Hongxia Li
| Hongxia Li received her MS degree in 2013 from Nanjing Agricultural University and received her PhD degree in 2016 from Jilin University. Since then, she has been a postdoctoral researcher with Prof. Geyu Lu. Currently, her research interests mainly focus on the development of functional nanomaterials for biosensors and bioimaging. |
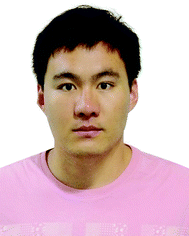
Xu Yan
| Xu Yan is currently an assistant researcher at Jilin University. He received his PhD degree from Jilin University in 2017. Since then, he has been a postdoctoral researcher with Prof. Geyu Lu and Prof. Junqiu Liu (2017–2019). His scientific interests focus on the development of functional nanomaterials for chem/biosensors. |
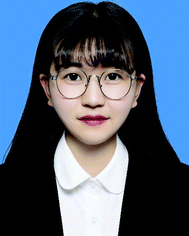
Deshuai Kong
| Deshuai Kong received her BS degree from the College of Chemistry (2018), Jilin University, China. Currently, she is studying for her MS degree at the College of Electronic Science and Engineering, Jilin University. Her research interests mainly focus on the development of functional nanomaterials for chem/biosensors. |
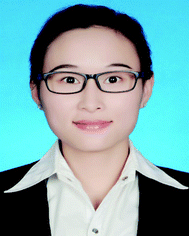
Rui Jin
| Rui Jin received her MS degree from the College of Chemistry (2016), Jilin University, China. Currently, she is studying for her PhD degree at the College of Electronic Science and Engineering, Jilin University. Her research interests mainly focus on the development of functional nanomaterials for chem/biosensors. |
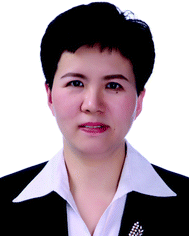
Chunyan Sun
| Chunyan Sun is a full Professor at Jilin University, China. She received her MS degree in analytical chemistry in 2002 from Beijing Normal University and received her PhD degree in 2006 from Changchun Institute of Applied Chemistry, Chinese Academy of Sciences. Her current research interests include the development of chem/biosensors for food safety. |
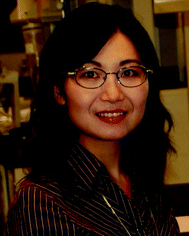
Dan Du
| Dan Du received her PhD in Analytical Chemistry from Nanjing University in 2005. She joined Central China Normal University in 2005 and was promoted to a Full Professor in 2011. Currently she is a Research Professor at Washington State University. Her research interests include functional nanomaterials for biosensing and drug delivery. She has published over 300 papers, with citations of 14 000, H-index 66. She is an Editorial member of Analytica Chimica Acta, Biosensors, and six other international journals. |
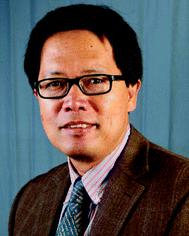
Yuehe Lin
| Yuehe Lin is a Professor at Washington State University. His research interests include electrochemistry, bioanalytical chemistry, chemical sensors and biosensors, nanomaterial synthesis and applications. He has over 20 patents and has published about 500 papers, with a total citation of 50 000, H-index 110. He is a Fellow of the American Association of the Advancement of Science (AAAS), the Royal Society of Chemistry (RSC), and the American Institute for Medical and Biological Engineering (AIMBE). He was elected to the Washington State Academy of Sciences in 2018. |
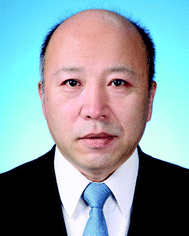
Geyu Lu
| Geyu Lu is a full Professor at Jilin University, China. He received his BS degree in electronic sciences in 1985 and MS degree in 1988 from Jilin University in China and his Dr Eng. degree in 1998 from Kyushu University in Japan. His current research interests include the development of chemical sensors and the application of functional materials. |
1. Introduction
The green fluorescent protein (GFP), which exhibits a bright green fluorescence, has opened a new area of biomedical research for monitoring protein–protein interactions, protein localization and gene expression in the past few decades, especially after the Nobel Prize in Chemistry in 2008 was awarded for its discovery and development.1,2 However, the poor photostability and weak fluorescence signal of GFPs make long-term imaging difficult, limiting their widespread applications in biomedical fields.3 Therefore, creating and exploring new fluorescent probes or fluorophore-tagged molecules become more and more urgent in in vivo and in vitro biomedical applications.
Since the original discovery of florescent carbon dots (CDs) in 2004,4 extensive investigations have been done on developing synthetic methods,5 understanding reaction mechanisms,6,7 and exploring various potential applications.8–10 Fluorescence (FL) CDs hold distinctive advantages marked by multicolor emissions, tunable optical properties, excellent photostability and prominent biocompatibility, making them promising scaffolds for biosensing,11–13 bioimaging,14,15 medical diagnosis,15–18 real-time tracking of drugs19 and tumor therapy.20,21 Due to their easy surface-functionalization and excellent biocompatibility, CDs can serve as effective tools in visual monitoring of biological processes and analysis of drug releasing kinetics. More importantly, pristine CDs can be prepared by simple, environmentally friendly, cost-effective and energy-efficient one-step synthesis procedures, such as microwave and hydrothermal methods. A wide range of natural sources (such as coffee beans,22 grass,23 lychee exocarp,24 crab shell,25 phyllanthus acidus26 and linseed27) have been employed as precursors for synthesizing CDs, indicating that CDs are easy to be prepared with less environmental impact. According to the study of Liu et al.,28 a one-pot synthesis method has been developed, in which large scale green-emissive CDs with high quantum yield can be obtained by simply keeping the mixture of triethylenetetramine and p-benzoquinone at room temperature. Thus, a wide range of methods in CD preparation provide promising opportunities for achieving significant performances for various applications, especially in vitro and in vivo bioimaging.
So far, several excellent reviews have been published to highlight the development of CDs regarding synthesis methods and properties,8,29,30 luminescence mechanisms,7,9,31–33 their applications in biosensing and bioimaging,34–36 their interaction with biomacromolecules,37 as well as the optical property regulations of red CDs in our recent review.38 Herein, we comprehensively survey the latest research on CDs’ applications in the rapidly evolving bioimaging field, which particularly focuses on the applications in imaging of different types of cells (cancer cells, stem cells and neural cells), imaging of cell organelles (nucleus, mitochondria and lysosomes) and the in vivo imaging-guided drug delivery and tumor therapeutics (Fig. 1). This review aims to provide readers with panoramic snapshots of the most recent, exciting and high-impact advances that have been made in this dynamically developing field. In the following sections, we first briefly describe the excellent properties of CDs. On this basis, we then reviewed the recent in vitro and in vivo applications of CDs. Finally, we discuss the challenges and share future perspectives.
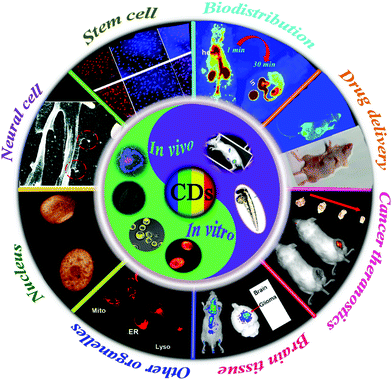 |
| Fig. 1 Schematic representation of applications of fluorescent CDs in bioimaging, including in vitro bioimaging with emphasis on cancer cells, stem cells, neurons, cell nuclei and other organelles, and in vivo imaging, especially in imaging-guided drug delivery, tumor therapeutics and brain tissues. | |
2. Properties
Due to the diverse structures of CDs, it is hard to illustrate all their properties formed from different precursors. Thus, only optical properties related to bioimaging are briefly summarized in this section.
2.1 Optical properties
Due to the strong absorption of the π-conjugated electron sp2 atomic skeleton, CDs exhibit strong optical absorption in the UV region. For example, an absorption band centered at 250–300 nm is recognized as a π–π* transition of aromatic C
C bonds, which is commonly observed in most CDs.39–41 In addition, the surface functional groups42,43 and size-dependent absorption properties44 play vital roles in the absorption characteristics of CDs. According to the study of Tetsuka et al., the functionalization with amino groups can induce a red shift in the absorption band of CDs.45 The most fascinating feature of CDs is the tunability of their FL properties, which arises from the quantum confinement effects, and the surface/edge effects of the large rigid π-conjugated structure and surface modification groups.8,15 In addition, the emission wavelengths of CDs can vary based on the excitation wavelengths, named excitation-dependent behavior.46 More interestingly, CDs are strongly two-photon luminescent upon excitation in the near-infrared region, and are highly desirable for biomedical imaging in deep tissues attributed to their reduced phototoxicity and enhanced penetration without background light.47–49 In particular, the two-photon fluorescent CDs in the research of the Zhou group not only were an excellent imaging contrast agent but also could promote release of drugs loaded in PEG-chitosan@CDs hybrid nanogels through the local heat produced by CDs under NIR irradiation.50 In addition, CDs also have excellent solubility, high photoluminescence quantum yield and outstanding stability with strong resistance to photobleaching.51–53 All above-mentioned properties of CDs lead to their potential bioimaging applications.
Currently, the FL emission light wavelength and luminescent color can be tuned from blue to red by adjusting the synthetic methods54 and changing the surface groups,55 which is highly favorable for multicolor imaging applications.56 So various synthetic methods have been developed to obtain more economical CDs in the past few years,17,57,58 such as electrochemical oxidation,59 solvothermal method,60,61 hydrothermal synthesis,62 acidic oxidation,63 microwave/ultrasonic method,64–66 rapid pyrolysis67 and MOF template assembly methods.68 Abundant biological species or natural sources, including proteins,69 amino acids,66 organic compounds70 and a variety of carbohydrates have been utilized as precursors for synthesizing CDs. Generally, the CDs synthesized from natural sources exhibit rich functional groups on the their surface, further facilitating the modifications of optical properties.27
2.2 Biocompatibility and biotoxicity
Carbon as the core element of life constitutes the backbone of biomolecules. Compared to other fluorescent nanoparticles such as metallic QDs, luminescent CDs are, therefore not surprisingly, more biocompatible with lower cytotoxicity in many investigated cell lines.13,50,71–75 Also, large amounts of research regarding in vivo cytotoxicity have demonstrated that CDs are usually nontoxic in mice and zebrafish testing.18,76–80 For example, Chen's group explored in vivo fate of CDs by means of positron emission tomography imaging and near-infrared fluorescence techniques.81 After being injected in mice via three routes (intramuscular, intravenous and subcutaneous), the CDs possessed low retention in the reticuloendothelial system, but displayed high tumor-to-background contrast at the same time. Leblanc and co-workers synthesized non-toxic carbon dots from carbon nanopowder, which had high affinity and specificity to bone-binding. These CDs have potential applications in bone-related disease imaging.79 Latterly, Du et al. designed gadolinium-doped CDs with stable FL properties to develop a novel theranostic nanoplatform for radiotherapy of tumors.82 The authors also found that the CDs were accumulated in the kidneys after vein injection and could be cleared away by the body through urination, which exhibited advanced biocompatibility. More recently, studies in rats have shown that aspirin-based CDs did not exhibit severe cytotoxicity in the liver, kidneys, spleen, heart, or lung, but at the same time possessed effective anti-inflammatory effects.49 Meanwhile no prominent phototoxicity or minimal tissue photodamage was observed in vitro experiments. Lemenager et al. explored the high-resolution cellular imaging of CDs in living cells using STED microscopy, and found no prominent phototoxicity in MCF7 cells after being incubated with CDs for 48 hours.83 Also, Das et al. synthesized sulfur and nitrogen doped CDs from whey protein, which were observed to save the cells from phototoxicity upon tracking the cells for 10 days.84 Thus, these literature studies show that CDs with optimal hypotoxicity and biocompatibility exhibit outstanding performance in the field of imaging application. Although more research studies reported that no tissue photodamage was observed in in vitro and in vivo tests,85 CDs functionalized with 2,2′-(ethylenedioxy)bis(ethylamine) on the surface were observed to have phototoxicity against cancer cells and bacteria in the research of Yang's group.86 Also, Kaur et al. found that their prepared nitrogen doped CDs exhibited significant visible light induced phototoxicity to cancerous cells, contributing to the possibility of employing them as a potential theranostic agent for cancers.87
Thus, most above methods for CD toxicity assessment were designed and carried out based on chemical toxicity while ignoring the side effects of CDs from nanoparticles (such as size-dependent toxicity) and their physical characterization (such as phototoxicity).87,88 The size, surface groups, concentration and dose, and preparation methods of CDs are the determinants of their biological activity that may lead to potential inflammation, oxidative stress, metabolic pressure and even chronic toxicity just as other carbon nanomaterials,89–92 which are not researched sufficiently. What is more, standardized methodologies and guidelines are absent, making it difficult to compare the safety/toxicity assessments from different research teams.93 Therefore, studies about the cytotoxicity of CDs, their real interactions with biological systems and their potential ecological risks should be assessed systematically for a long time.94,95
3.
In vitro imaging and in vivo biomedical applications
With their unique properties including their capability to tune surface functions, superior photostability, high brightness, prominent biocompatibility and spontaneous penetration capabilities, CDs have shown excellent potential to be used as probes for analyzing biological systems, particularly appealing for imaging-guided biomedical applications.15–17,96,97 However different types of cells or tissues have special shapes and structures, as well as corresponding biomarkers on the membrane or in the cytoplasm, leading to a specific response to foreign nanoparticles.98 Thus, special functional CDs have been synthesized on the basis of unique membrane lipids, proteins, targeting ligands and biomarkers of different cells for developing impactful methods in imaging applications.99 Herein, we firstly focus on three different vital kinds of in vitro CD-based cell imaging including cancer cell imaging,14,36,40 stem cell imaging,100 and neuron imaging,99 which provide the possibility of using CDs for in vivo applications, especially being helpful in exploring the development, diagnosis and treatment of cancers and neurological diseases, discussed in the section of in vivo biomedical applications of CDs. In addition, the imaging of organelles including nucleus imaging,101,102 mitochondrial and endoplasmic reticulum imaging etc.103 were also reviewed, which will be profitable to understand and study organelle-related diseases.
3.1
In vitro imaging
3.1.1 Cancer cell imaging.
Cancer, like ghosts, is obsessed with human health, devouring the lives of millions of people each year. Therefore, advanced methods for early cancer diagnosis are becoming more and more significant for preventing tumor development. Specifically functionalized CDs can penetrate many kinds of cancer cells and probe them more efficiently by FL imaging.9,36,71,104–110 The special recognition mechanism is primarily dependent on the interaction between functional CDs and the surface groups of cancer cells. As shown in the study of Ruan et al., PEGylated CDs were developed by using glucose and glutamic acid as precursors, and the as-prepared CDs could specifically anchor glioma cells through recognizing the surface group of angiopep-2, resulting in higher sensitivity for glioma imaging over normal brain tissues.111 In addition, our group prepared multicolor FL CDs for cell imaging and biosensors with outstanding biocompatibility, among which the yellow emission CDs were successfully taken up by breast cancer cells (MIDA-MB-231 and MIDA-MB-68) through endocytosis and mainly diffused into the cytoplasm.41,112 Wang et al. developed dual-element CDs with nitrogen and chloride. Such CDs exhibited strong pH-sensitive fluorescent emission for sensing intracellular pH and cytochrome c by multicolor imaging in HeLa cells.110 Recently, in order to save cost and simplify the synthesis procedure, more environment-friendly CDs were synthesized using natural source biomass with lower environmental impact and applied in cancer cell imaging. The Liang group24 developed natural biomass carbon dots for near-infrared fluorescence imaging-guided PDT of cancer cells and mouse tumors. Also, Ramanan and co-workers synthesized photoluminescent CDs from eutrophic algal blooms, which acted as a potential biomarker in the imaging of MCF-7 cells.40 These research studies showed that fluorescent CDs provide a new potentiality in biological studies of cancer cell identification.
Great efforts have been devoted to improving the specificity of CDs to target cancer cells. The Abdollah Salimi group14 prepared an ultrasensitive antibody-ssDNA aptamer sandwich-type fluorometric immunosensor for sensing the specific CA125 biomarker, in which a CD–antibody hybrid was used as the probe for selective imaging of the cancer cells over the OVCAR-3-line cells for ovarian cancer diagnosis. Gao et al. prepared silicon and nitrogen co-doped multifunctional carbon dots and applied their mixed solution with Fe3+ to successfully distinguish cancerous cells (A549 and Hep G2) from normal ones because of the different content of GSH by cell imaging.74 In addition, N-doped CDs coated with an antibody mimic layer were prepared to specifically recognize tumor biomarkers with high affinity.113 However, specific labeling of cancer cells with CDs remains the notable challenge in cell imaging as recognition elements and ligands on cancer cell surfaces are not found in only one kind of cancer cell. For example, folic acid conjugated CDs were used for imaging of cancer cells, but the folate receptor occurred not only in hepatoma cells or breast cancer cells. Thus, improving the specificity and targeting capability of fluorescent CDs is much more significant for tracing cancer cells.
3.1.2 Stem cell imaging.
Labeling strategies for stem cells are urgently needed for regeneration medicine in order to understand the natural organ development process114–116 and the contribution of stem cells to various tissue regeneration including bone repair,117 skin renewal118 and neurorepair.119 Due to their small size and outstanding biocompatibility, CDs can effortlessly penetrate stem cells for imaging via the endocytosis mechanism in a concentration- and time-dependent manner as other carbon nanoparticles.120 Thus, herein we reviewed CD-based probes for imaging of stem cells and monitoring their proliferation.
Zhang and co-workers developed yellow-emission CDs to label three different kinds of stem cells without any obvious impact on their proliferation or differentiation capacity during long-term imaging.121 Meanwhile, as depicted in Fig. 2A and B, Liu et al. synthesized green emission CDs for successfully probing the live stem cell.122 However, the interaction between CD probes and stem cells has not yet been studied carefully. Recently, the Yang group employed citric acid-based CDs and their derivatives for labeling and tracking of rat bone marrow mesenchymal stem cells (rBMSCs).123 The authors found that these CDs could facilitate high-efficiency osteogenic differentiation of rBMSCs toward osteoblasts by boosting osteogenic transcription and increasing matrix mineralization (Fig. 2C). Afterwards, Chen et al. reported the promoting effects of multifunctional photoluminescent CDs for neuronal differentiation of EMSCs through a non-viral gene delivery mode, because the as-prepared CDs possessed admirable properties to condense macromolecules of the plasmid DNA.99 As displayed in Fig. 2D, the synthesized porphyra polysaccharide-derived CDs exhibited not only significantly high transfection efficiency, but also high performance in neuronal differentiation of EMSCs. These findings showed that CDs would be applied as both safe and effective gene carriers to guide the differentiation of adult neuronal stem cells.
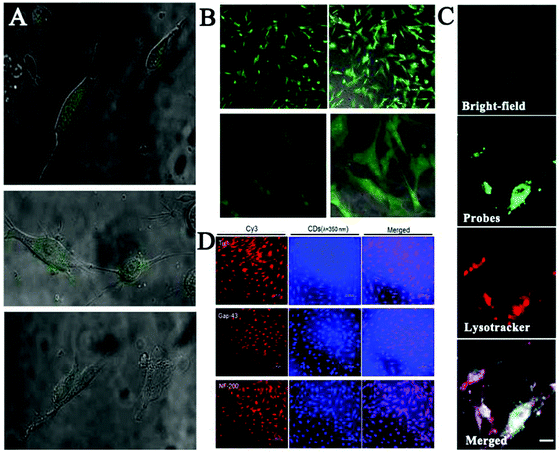 |
| Fig. 2 (A) Fluorescence (fluorescence + bright-field) images of the live stem cells and (B) the fixed stem cells labelled with the EDA-CDs.122 (C) Long-term rBMSC labeling and tracking by CDs (green) co-located with LysoTracker (red).123 (D) Immunofluorescence staining using a Cy3-conjugated secondary antibody (red) for neuronal markers including proteins of Tuj1, Map2 and Tau. The converted cells were also visualized with the bright blue fluorescence of CDs when excited at a wavelength of 350 nm.99 | |
Undoubtedly, the combination of excellent-performance CDs and various kinds of stem cells will dramatically enhance our understanding of the fate of stem cells and control their functions. Nevertheless, some vital factors such as the interaction format, cytotoxicity and the proliferation of CD probes into stem cells should be studied carefully, finally contributing to establish novel stem cell technologies, which would ultimately promote stem cell-based therapeutics for the prevention, diagnosis and treatment of human diseases.
3.1.3 Neural cell imaging.
To further improve our understanding of the properties and functions of the nervous system, development of new imaging methods to visualize the relationships among the brain structure, neuron activity and neurochemistry is in great demand. Advances in nanomaterials may help overcome the current imaging challenge in neurosciences. Some research has been done in vitro to investigate the interaction between neurons and CDs based on assessing the cytotoxicity of CDs, the application of CDs as gene carriers for drug delivery and their use in electric activity sensing and tracking. For example, Gao et al. found that the red emission CDs did not affect the PC12 cell viability with a gradual increase of CD concentration.80 However, CDs probes in neuron cells and the central nervous system (CNS) have been less investigated in terms of their potential value in the diagnosis and therapy of human CNS diseases. The Lu group developed a direct and more economical fluorescent neural tracer based on cholera toxin B-carbon dot conjugates (CTB-CDs), which could be taken up and retrogradely transported by neurons in the peripheral nervous system of rats. This tracer can be used in in vitro and in vivo bioimaging of neuron cells or tissues based on the special binding ability to gangliosides from the nerve cell membrane and nervous tissues (Fig. 3A).99 As illustrated in Fig. 3B, Khan and co-workers used dopamine (DA), an important inotropic vasopressor agent in neurological diseases, to anchor the biocompatible CDs for controlling drug release under physiological conditions.124 The tenure of DA anchored on CDs released at pH 7.4 was greatly extended to 60 h, which exhibited great biocompatibility to Neuro-2A cells and non-infliction of any anatomical distortions or hostile effects on tissues in vivo, additionally as shown in Fig. 3C. More interestingly, Nissan et al. synthesized Ga-CDs-Ga-coated substrates, which showed a 97% increase in branch number originating from the soma and played an important role in neural growth (Fig. 3D).125
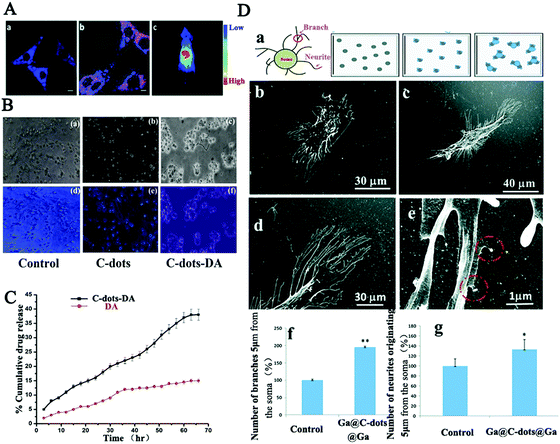 |
| Fig. 3 (A) Bioimaging of cholera toxin B-CDs in PC12 cells and in nude mice.99 (B) Fluorescence microscopy of Neuro 2A cells treated respectively with CDs-DA, DA, PBS and (C) the release rates of CDs-DA and DA with respect to time.124 (D) SH-SY5Y cell morphology demonstrating branch and neurite counting on the modified substrate, on glass, Ga-CDs-Ga coated substrates, and the HRSEM images of neurite branches and branch numbers.125 | |
Taken together, carbon dots having spontaneous penetration capabilities together with special functional modification based on the unique neural membrane lipids and proteins exhibit an excellent possibility for the development of impactful methods in the central nervous system for clinical diagnosis and therapy. However, a further and deep toxicological evaluation of their roles in the central nervous system and in the whole body is highly desirable.
3.1.4 Nucleus imaging.
Staining of nuclei is crucial to reveal their morphology and their roles in cell metabolism, growth, differentiation and heredity.126 Due to their small nanometer sizes (<10 nm), highly positive charge obtained from precursors with a quaternary ammonium group, and strong absorption capability on the membrane, CDs as newly developed nanomaterials may be incorporated into a cell nucleus for binding to the DNA and RNA. Datta et al. proved the uptake of CDs into the nucleus for the first time.101 In their study, the authors combined cationic quaternized CDs with anionic graphene oxide sheets to form biocompatible hybrid nanomaterials. Owing to the electrostatic interactions with the negatively charged components of the lipid membrane, the hybrid nanomaterials as the bioprobe could easily internalize into the nucleus of NIH/3T3 cells. After that Yin's group prepared dopamine-mimicking nitrogen-doped CDs as a nucleus-staining agent for cell imaging. The great performances of the nitrogen-doped CDs in nucleus staining, which is attributed to their small sizes, surface positive charges and dopamine-mimicking properties, were validated within four different types of cancer model cells, including rat PC12, A549, HepG2 and MD-MBA-231 (shown in Fig. 4A).102 In addition, Khan et al. discovered yellow-orange emissive CDs localizing in the nucleolus of HeLa cells for specifically binding to RNA, which was confirmed by an RNase digestion test (shown in Fig. 4B).127 Recently, in our research, the as-prepared CDs showed a hydrogen-bonding-induced emission (HBIE) phenomenon with good biocompatibility, ultrasmall sizes, and strong affinity for nucleic acid, facilitating the in situ imaging of nuclei in living cells.128
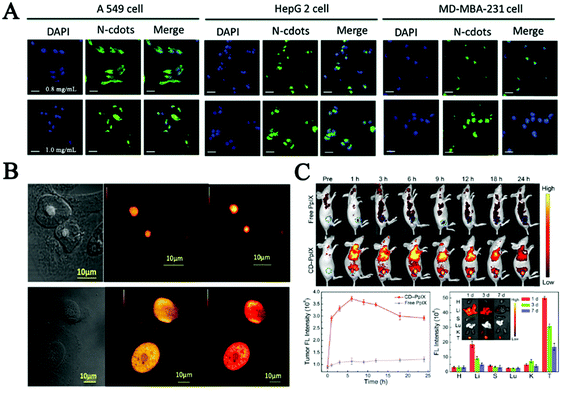 |
| Fig. 4 (A) Fluorescence images of A549, HepG 2 and MD-MBA-231 cells after incubation with medium containing different concentrations of the N-CDs for 12 h.102 (B) RNase digestion results confirmed that the carbon dots mainly bind to RNA in the nucleolus, in which CDs localized specifically in the nucleolus (a) and after digestion with RNase enzyme CDs localized all over the nucleus of native HeLa cells (b).127 (C) Fluorescence images in vivo, quantitative fluorescence analysis of the tumors after intravenous injection, and biodistribution of CD-PpIX in tumor-bearing mice for different time periods in key tissues and tumor of the mice.131 | |
Furthermore, nucleus-targeting CDs could be exploited to facilitate drug transportation or mediate gene transfection. Yang et al. employed functionalized CDs with a nuclear localization signal peptide to transport doxorubicin (DOX-CDs) into cancer cells. Cell imaging results confirmed that DOX-CDs were mainly located in the nucleus and could efficiently cause apoptosis in human lung adenocarcinoma A549 cells.129 These results firstly demonstrated the potential applications of CDs in gene-target delivery. Inspired by the above strategy, the Kim group presented a ternary complex using polyethylenimine-functionalized CDs and plasmid DNA for in vitro transfection and monitored plasmid cellular trafficking in real time. After that, low molecular weight polyethylenimine showing efficient ability for gene transfection was used to synthesize composites with CDs for delivery of siRNA and plasmid DNA in vivo.130 What's more, in the study of Hua et al., multifunctional CDs were synthesized for imaging nucleoli in both fixed cells and living cells. The in vivo studies showed that these CDs possessed a negatively charged surface. Conjugating with protoporphyrin IX (PpIX) enabled the CDs to obtain tumor-targeting ability. Therefore, applying such CDs in cancer therapy can result in effective tumor ablation with little toxicity effects after laser irradiation, as shown in Fig. 4C.131
As a summary of this section, a CD-based nanoplatform for nucleus imaging is very important for real-time live-cell tracking and drug delivery. The fast uptake and nuclear entry mechanism of CDs could be attributed to their small size and good biocompatibility. However, the reason behind the ability of multifunctional CDs to avoid lysosomal/endosomal entrapment and selectively target nucleus is not clear.
3.1.5 Imaging of other organelles.
Although the fluorescence imaging technique for monitoring cellular biological processes has become more and more mature, further efforts should be made towards subcellular imaging and organelle analysis for long-term tracking of the changes in cell morphology and cell function. These studies will be conducive to further understanding the direct relationship between organelles and major diseases.
As one of the vital subcellular organelles, mitochondria is the powerhouse producing ATP and metabolic center in cells. The dysfunction of mitochondria is responsible for many diseases including inflammation, neurodegenerative diseases, diabetes and cardiac dysfunction.132–135 Consequently, tracking the status and behavior of mitochondria is crucial to modulate cellular fate and mitochondrial diseases. CDs that have great cellular permeability, photostability, great water solubility and long tracking time have been reported as new mitochondrial-imaging probes in many investigated cell lines.103,136 Du et al. fabricated multifunctional FL nanoprobes based on mitochondria-targeting ligand-linked CDs for imaging the mitochondrial H2O2 in Raw 264.7 cells.137 Then, in order to reduce the cost and improve the photostability, Hua et al. ingeniously prepared intrinsic mitochondria targeting CDs without any further modifications of mitochondrial tropic ligands.103 As depicted in Fig. 5A, the as-produced CDs could quickly penetrate cells and specifically anchor on mitochondria via temperature-dependent transport and caveolae-mediated endocytosis, which provided great promise in mitochondrial imaging and mitochondria-targeted photodynamic cancer therapy. Furthermore, great efforts have been made to improve the mitochondria targeting capability of CDs. Wu et al. developed a fluorescent CDs based mitochondria-targetable nanoprobe (CDs-TPP) for FL imaging of peroxynitrite in mitochondria.136 The image staining with both nanoprobe and Mito-tracker showed that CDs-TPP had excellent mitochondria-targeting ability and great imaging contrast in MCF-7 cells (Fig. 5B).
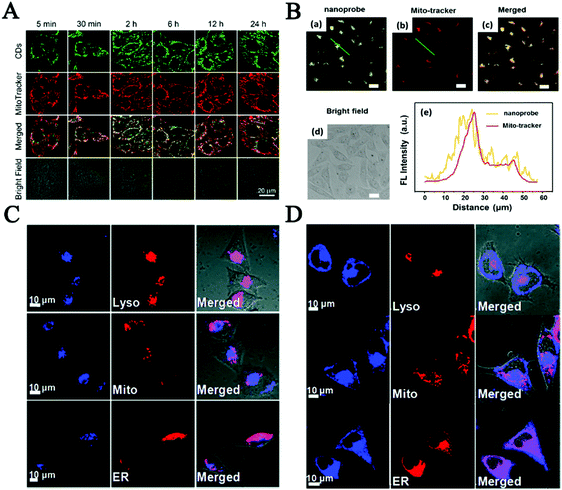 |
| Fig. 5 (A) Co-localization of CDs and mitochondria for MCF-7 cells.103 (B) Confocal images of MCF-7 cells co-stained with both CDs and Mito-tracker, and the fluorescence intensity profile of the linear region of interest across a selected single cell.136 Fluorescence images about CD co-location with lysosome probes (C) and ER tracker (D) in MCF-7 cells.144 | |
Another important subcellular organelle, lysosomes as the digestive system in cells play a vital role in undesirable protein degradation, cell signal transduction, plasma membrane repair and homeostasis, endocytosis and autophagy.138–140 So far, lots of efforts have been devoted to preparing fluorescent CDs for lysosome imaging in order to understand the relationship between abnormal lysosomes and the related diseases. The Huang group prepared functional CDs with large amounts of amino groups to specifically target lysosomes and to be used as a probe to analyze lysosomal pH changes sensitively during apoptosis of A549 and Hep-2 cells.141 In the study of Wu, abundant amine and morpholine groups were conjugated on the surface of CDs to improve their water solubility and targeting ability in lysosomes. The results indicated that the as-prepared CDs were highly selectively accumulated in acidic lysosomes of living cells but diffused less into the cytoplasm through the endocytic pathway.142 Most of these CDs require post-modification with lysosome targeting morpholine ligands. Recently our group reported intrinsic lysosome targeting CDs without any post modification but based on the acidotropic effect for lysosome's low pH value (4.5–5.5).143 What is more, inspired by the new concept of surface functionality of CDs for subcellular labeling, the Wang group made decorated CDs not only for probing lysosomes but also for the endoplasmic reticulum, which plays an important role in multiple cellular processes, including intracellular calcium homeostasis, protein secretion and lipid biosynthesis, as the quality-controlling center of proteins.144 The cellular uptake processes reflected that the CDs functionalized with amine groups mainly localized in lysosomes (Fig. 5C) and the laurylamine functionalized carbon dots finally overlapped with ER-Tracker mainly via lipid-raft endocytosis and clathrin-mediated endocytosis, as shown in Fig. 5D. These research studies would pave a new path for developing CD-based fluorescent probes for subcellular labeling. However, there is still a long way to go to develop effective CD probes for fluorescent imaging of subcellular organelles and analysis of their dynamic changes in live cells.
These potential applications for in vitro imaging reflect that CDs are highly promising to open new opportunities in biomedical applications, serving as competent nanocarriers for fabricating drug delivery systems to cancer cells and organelle-targetable nanoprobes. However, current CD-based probes still have drawbacks in some respects and much more efforts should be made in the development of multifunctional CDs with better properties, such as excellent targeting capability, easy surface functionalization, non-cytotoxicity, high quantum yield and high fluorescence stability for in vitro imaging. For instance, the emissions of CDs showed red-shift by further increasing the excitation wavelength, while the intensity is usually rather low. Also, the targeted properties and applications of CDs in in vivo imaging and clinical diagnosis should be studied in detail, which are highlighted as follows.
3.2
In vivo biomedical applications
On basis of in vitro studies, lots of endeavors have contributed to in vivo biomedical applications based on FL CDs for future clinical diagnosis and treatment in recent years.5,145–149 Yang et al., for the first time, reported the in vivo imaging of CDs by adopting three injection avenues in mice.76 Subsequently, more and more studies have been carefully performed to explore the in vivo application of CDs by animal models. In this section, we summarized the CDs’ biodistribution, clearance and uptake, and their red fluorescence imaging-guided cancer theranostics, and imaging probes to brain tissue and brain tumor.150
3.2.1 Biodistribution and uptake of CDs in mice and zebrafish.
Although CDs play a vital role in current biomedical research, they may possess chronic toxicity, low stability, and potential undesired accumulation in organs/tissues. So, CDs’ biodistribution and uptake in vivo must be evaluated. Tao et al. for the first time performed a systematic investigation of the performance of near infrared (NIR) FL CDs under in vivo conditions and analyzed the biodistribution of CDs in mice using a radiolabeling method. The results showed that the CDs first heavily accumulated in the reticuloendothelial system and kidneys, and then they were gradually cleared via renal and fecal pathways with low toxic effects in mice.77 Motivated by the above study, some researchers reported the in vivo biodistribution, metabolic pathway, and tumor uptake mechanism of the near-infrared FL CDs. According to the study of Huang et al., the CDs could be quickly excreted from the body after three different injection routes in mice, and its clearance rate was ranked as intravenous (tail vein) > intramuscular (muscle of left leg) > subcutaneous (under the skin of left leg),81 as shown in Fig. 6A. In addition, as indicated in Fig. 6B, Wang and co-workers observed that the polymer-coated nitrogen-doped CDs were accumulated within the glioma based on the enhanced permeability and retention effect (EPR), and then mainly administrated to the brain, liver and kidneys after 90 min post-injection for in vivo glioma targeting FL imaging.151 After being injected into mice, the CDs were mainly taken up by the cells in the bladder and tumor, while the liver cells showed negligible uptake of CDs. Recently, Nadia et al. investigated the biodistribution and uptake of CDs in rats and tumor mice through radioelement labelling and dynamic experiments of positron emission tomography, which showed a rapid renal clearance of CDs from the in vivo systems. But the biodistribution and pharmacokinetic properties of CDs are strongly affected by the surface charge, positive zeta potentials and hydrophilicity of the CDs,150 which were significantly accumulated in the liver and intestine (Fig. 6C). The research mentioned above showed that the toxicity, uptake, biodistribution and clearance of CDs in vivo were correlated with surface functional groups, their zeta potential value, as well as the methods of synthesis and purification.
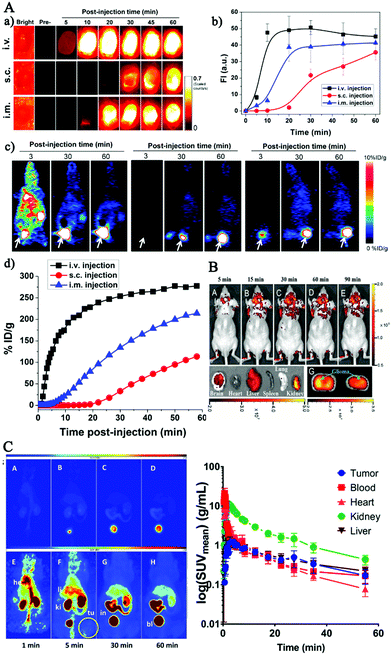 |
| Fig. 6 (A) Representative imaging of 64Cu-CDs after injection through three routes in mice (intravenous injection, subcutaneous injection, intramuscular injection).81 (B) In vivo imaging of glioma-bearing mice intravenously administered with the pN-CDs at various time points post-injection and after pN-CD administration for 90 min (F).151 (C) Maximum intensity projections obtained from a PET study after a single intravenous injection of [64Cu] Cu-NOTA-CDs in A431 tumor-bearing mice.150 | |
In summary, these works provided promising information for researchers to investigate applications of fluorescent CDs for delivering drugs, monitoring the therapeutic effects and other clinical applications in future. Meanwhile, it is worth further studying their surface modification to reduce non-specific protein adsorption onto CDs in vivo and to make sure rapid extraction and quick transport to the targeting tissue for decreasing the potential cytotoxicity of CDs when used as cancer imaging agents in diagnosis.
3.2.2 Imaging-guided drug delivery in mice.
Doxorubicin (DOX) and cisplatin(IV) chemotherapy has been used in the treatment of numerous tumors for many years through disrupting DNA in the cancer cell nucleus and preventing division of tumor cells, including liver cancer, breast cancer, lung cancer, glioma, gastric cancer, ovarian cancer and so on.152 Nevertheless, normal cells also suffer the toxicity from these drugs owing to the poor targeting of the drugs, which may potentially cause chronic damage to other organs and increase extra side effects. In order to design a drug release system accurately targeting at cancer cells, CDs with remarkable optical properties, great contrast, excellent biocompatibility and excellent sensitivity in fluorescent imaging have been applied to discriminate cancer cells from normal cells as the imaging-guided nanocarriers for specifically labeling and monitoring chemotherapy drugs.153–157
A Förster resonance energy transfer-based CD imaging-guided platform has been developed by Zheng's group for drug delivery. This platform exhibited excellent biocompatibility, stable fluorescence, convenient cell imaging, and real-time monitoring capability of drug release.154 However, its practical in vivo application is unsatisfactory due to its insensitive surface characteristics to complex tumor microenvironments. With increasing demand for specific fluorescent bioimaging agents, Feng and co-workers designed a CD-based cisplatin prodrug-loaded nanocarrier, which could achieve charge convertibility when the pH of the tumor extracellular microenvironment changed, leading to imaging-guided drug delivery.155 The charge-convertible CDs not only exhibited enhanced cellular internalization and effective activation of cisplatin prodrug in the reductive cytosol, but also displayed strong tumor growth-inhibition capability and little side effects in vivo. Inspired by the distinction of pH values between tumor tissues and paracancerous tissues, Gong et al. fabricated a pH-controlled DOX-releasing nanocarrier named PNHCDs (phosphorus and nitrogen doped hollow CDs) with properties of high anticancer drug loading rate, rapid cellular-uptake, and small particle size, hollow structure, and abundant phosphate/hydroxyl/pyridinic/pyrrolic-like surface functional groups, which showed highly effective tumor growth inhibition capability and improved targeted cancer therapy efficacy.158 However, it is still a great challenge for cancer drugs to conquer the barrier of the nuclear membrane and lysosomal/endosomal entrapment, and ultimately achieve nucleus-anchored drug delivery and photodynamic therapy. So nuclear drug delivery systems based on CDs have been developed for tumor treatments. Pan's group developed an online imaging drug delivery and NIR irradiation treatment platform by conjugating FeN-CDs with folic acid (FA), riboflavin (Rf), and anticancer drug DOX to form a GP-Rf-FA-FeN-CDs-DOX complex.159 Both in vivo and in vitro tests were performed using the developed complex and the results are shown in Fig. 7, which revealed that the developed complex could deliver DOX to targeted cells, and release them intracellularly under NIR irradiation, resulting in effective tumor elimination through chemo-photo synergistic therapeutic means. The research of the Zhang group also achieved a combination of targeted bioimaging, hyaluronidase analysis and targeted drug delivery by using polyethylenimine modified CDs (P-CDs) and Hyaluronic acid conjugated-Dox (HA-Dox). The results showed that the P-CDs/HA-Dox could specifically target CD44 receptors on cancer cells and penetrate them due to the high affinity between HA and CD44 receptors. Moreover, under the activation of HAase, P-CDs/HA-Dox restored the fluorescence of P-CDs, leading to enzyme-triggered Dox release for effective treatment of cancer.160
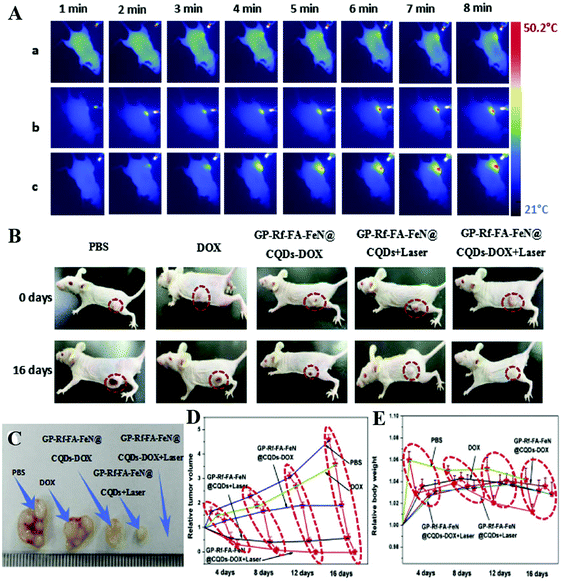 |
| Fig. 7 Thermal images of tumor-bearing mice exposed to 671 nm laser radiation after injection of the CDs-DOX complex for different times (A), the real photographs of tumor-bearing mice (B) and tumor sizes (C), growth curves (D) and weight curves (E) of mice after injection of the CDs-DOX complex for 16 days as well as controls.159 | |
All above work proves the capability of CDs as smart drug nanocarriers with enhanced chemotherapy effects and provides strategies to improve the potential clinical applications of CDs in imaging-guided cancer theranostics. However, selective targeting and prolonging the residence time in cancer tissues, as well as the biological toxicity to normal tissues of these anticancer drug delivery complexes based on CD imaging remain worth studying.
3.2.3 Red fluorescence imaging-guided cancer theranostics.
As we all know, biocompatible CDs possess great potential as a nanoplatform for clinical biomedical applications, especially in optical cancer theranostics. Moreover, the spontaneous FL emission of biological molecules and the background light scattering of biological samples in the short-wavelength region strongly interfere with the excitation and emission of CDs in both near-infrared (NIR-I and NIR-II) windows, and therefore, providing deeper FL penetration into the tissue sample and achieving the efficient imaging contrast still remain a great challenge.77,161 Ge and co-workers first developed novel red-emissive CDs with a strong photoacoustic response and high photothermal conversion efficiency (η ≈ 38.5%). These CDs were used for living mice imaging through intravenous injection.162 The results showed that the CDs exhibited the enhanced permeability and retention (EPR) effect and accumulated in the HeLa tumor area. A significant FL signal was observed in the tumor area in comparison with other tissues after being treated with intravenous injections of CDs in tumor-bearing nude mice. Soon after, Ge et al. prepared red emitting CDs by utilizing polythiophene benzoic acid as the carbon source.163 The synthesized CDs emitted bright FL in the red region and possessed dual photodynamic and photothermal effects with singlet oxygen (1O2) generation and high photothermal conversion efficiency under 635 nm laser irradiation. As shown in Fig. 8A, these unique characteristics made CDs a red-light-triggered theranostic agent for imaging-guided photodynamic–photothermal combination therapy within the therapeutic window (600–1000 nm).
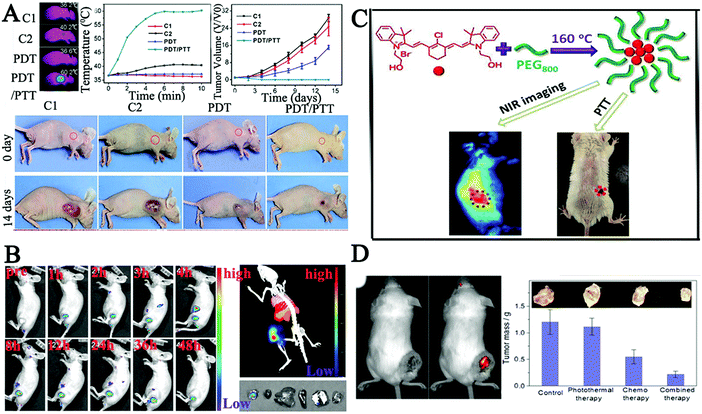 |
| Fig. 8 (A) Photothermal imaging and treatment effects of PDT/PTT in HeLa bearing tumor mice after being injected with CDs via the tail vein.163 (B) In vivo imaging of PCCN at different time points after intravenous injection and images of the tumor, liver, spleen, and lung as red fluorescence after PCCN treatment.166 (C) Synthesis of new CDs with intrinsic theranostic abilities, imaging in model mouse bearing tumors after the intravenous injection of CDs and the tumor-bearing mouse after PTT.54 (D) Therapeutic effect on tumor in tumor-bearing mouse after treatment with DOX-cohesive CD hybrid nanogels.168 | |
Alternatively, doping of nitrogen atoms into CDs is propitious to obtain the red-shifted PL emission,164,165 which is due to the electron-doping effect of the doped graphitic nitrogen atoms. Inspired by these results, Zheng and co-workers assembled multifunctional CDs using C3N4 to enhance red region absorption and promote an in vivo water splitting process. The authors assembled CDs with the polymer to overcome tumor hypoxia using a protoporphyrin photosensitizer, a tumor-targeting Arg-Gly-Asp motif and a linker molecule polyethylene glycol (PCCN).166 Cell viability tests indicated that the water splitting CDs could completely inhibit the hypoxia-triggered PDT resistance, with excellent growth inhibition of cancer cells. In vivo experiments also demonstrated that PCCN had superior ability to overcome tumor hypoxia as shown in Fig. 8B. Meanwhile, Zheng et al. prepared new NIR CDs (CyCD) from a hydrophobic cyanine dye and poly(ethylene glycol) via a solvothermal process, which showed preferential uptake of tumor cells and high photothermal conversion efficiency.54 The results demonstrated that the CyCD was an outstanding candidate for efficient NIR FL cancer imaging and therapy in vivo as shown in Fig. 8C. In order to further increase the level of red-shift, Ye's group utilized fluorine and nitrogen to co-dope by a one-step microwave-assisted carbonation route, resulting in CDs with red FL emission.167 Zhou's group also reported pH-sensitive chitosan CD hybrid nanogels bearing –NH2/–OH/–COOH groups (CCHNs, 65 nm) for highly efficient NIR imaging-guided cancer therapy with low side effects.168 The pH/NIR dual-responsive properties of CCHNs not only displayed high colloidal stability, and bright and stable FL from the UV to NIR wavelength, but also showed efficient NIR photothermal conversion and intelligent drug release, which indicated that CCHNs showed a great promise as a multifunctional intelligent nanoplatform, Fig. 8D. In addition, metal particles could enhance the fluorescence intensity and improve the NIR absorption properties due to the localized surface plasmons. A combination of N-doped CDs and transition metal ions (Cu) was designed for the application of cancer theranostic nanoplatforms.169 The results showed in vivo photothermal/photodynamic therapy (PTT/PDT) of cancer via Cu, N-CDs as a kind of IR thermal imaging agent to monitor the temperature dynamics in the photo-therapeutic process.
The above studies of this section about red FL imaging-guided cancer theranostics in vivo showed similar results, proving in principle that these functional red emission CDs with targeted abilities not only are promising to be good candidates for efficient NIR fluorescent cancer imaging, but can also serve as red-light-triggered theranostic agents for imaging-guided photodynamic–photothermal combination therapy in vivo. Unfortunately, these promising CDs are almost rapidly cleared from the body of mice and the short retention time has impeded their biological applications. Thus, it is urgent to develop new strategies to overcome these limitations. Recently, Zheng's group utilized mitochondria loaded with doxorubicin hydrochloride as an efficient delivery system of CDs for imaging guided cancer therapy, with prolonged retention time and enhanced therapeutic effect in a mouse model.170 Meanwhile, it is becoming important to develop novel CDs with special capability for overcoming the tumor microenvironment such as hypoxia and substantially increasing PDT efficacy as reported in the research of Jia.171 Therefore, much more work about how to improve wide-range systemic biodistribution and prolong the retention time of the red FL CDs in various cancer tissues should be carried out.
3.2.4 Imaging probes to brain tissues and tumors.
Delivering fluorescent probes to brain tumors and normal nerve terminals presents one of the most challenging issues in neuroscience applications due to the blocking effect of the blood brain barrier (BBB). BBB penetration of CDs strongly depends on their small particle sizes and hydrophilic surface properties, which extend the circulation time and promote passive targeting via enhancing the permeability and retention effect.172,173
It has been reported that CDs with small sizes (>5 nm) exhibited great BBB crossing capabilities and possessed strong preference to accumulate in the brain over other organs, thus bringing a new prospect in the treatment of malignant glioma. Moreover, the CDs could target a human glioma xenografted in mice brain,16 and epifluorescence imaging indicated that they displayed a maximum uptake in the brain just 5 min after the tail vein injection, and then the CDs were quickly cleared by mice from the tumor site. Subsequently, Zheng et al. synthesized novel tunable full-color emitting CDs (CDs-Asp) with excellent biocompatibility by using glucose and aspartic acid (Asp) for targeting toward glioma in a mouse model, which is the most common primary tumor in the central nervous system.174In vivo imaging showed that high-contrast biodistribution fluorescent signals of the Asp-functional CDs were detected in the glioma mass 20 min after tail vein injection (Fig. 9A), indicating that the ability of these CDs to freely penetrate the BBB and accurately target the glioma site rather than normal brain tissues (the hippocampus and cortical layer 24 h after injection) (Fig. 9B). Therefore, Asp-modified CDs as a FL probe had the guided targeting potential for diagnosis of glioma, one of the important intracranial primary tumors.
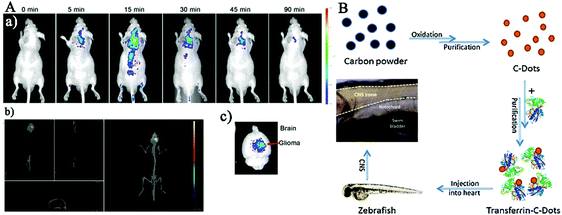 |
| Fig. 9 (A) The imaging of glioma-bearing mice after tail intravenous injection of CDs-Asp at different times and the CDs-Asp distribution in the brain 20 min after injection. (B) Distribution of CDs-Asp in glioma, hippocampus and cortical layer 24 h after injection as the green fluorescence.174 | |
Also, the biocompatible nitrogen-doped CDs (N-CDs) could cross the BBB in a concentration-dependent manner as demonstrated in the real-time live-cell imaging and in vitro BBB model in Lu's study.175 Therefore, these PEI-passivated N-CDs with BBB-penetration capabilities hold promise for traceable drug delivery to the brain. Based on the advantage that CDs exhibit great BBB crossing capabilities and a tendency to accumulate in the brain, the possibility of using transferrin-conjugated CDs to transport drugs across the BBB to target cells has been intensively explored. Borisova et al. analyzed the neuroactive effects of fluorescent CDs on the key characteristics of both GABA (glutamate and γ-aminobutyric acid) and glutamatergic neurotransmission in isolated rat brain nerve terminals.176 The results indicated that CDs in a dose-dependent manner decreased exocytotic release of [3H] GABA and L-[14C] glutamate, attenuated the initial velocity of Na+-dependent transporter-mediated uptake of [3H] GABA and L-[14C] glutamate, and increased the neurotransmitter ambient level of nerve terminals. The combination of the fluorescent and neuromodulatory features of CDs enables their potential usage in probing and visualization of key processes in nerve terminals and related neurological processes in theranostics.
This part of results highlights the promising applications of red emission CDs in the development of intelligent nanomedicine that possesses both diagnostic and therapeutic functions. Current red fluorescence CD-based probes still have some drawbacks and are far from meeting the practical requirements. For instance, the emissions of CDs showed red-shift by further increasing the excitation wavelength, while the intensity is usually rather low.
4. Summary and perspectives
In the past decade, enormous progress has been achieved in biomedical applications of fluorescent CDs, which consist of the most valuable and intriguing group of nanomaterials due to their unprecedented imaging sensitivity, penetration depth in the long-wavelength region, surface multifunctional capabilities, and cost-effective and eco-friendly synthetic methods. We have elaborately discussed the recent advances in in vitro bioimaging based on CDs, concentrating on the imaging of cancer cells, stem cells, neural cells, nucleus and cell organelles. The imaging guided in vivo biomedical applications have also been systematically introduced. We highlight impressive studies mainly of past three years, including preparations of novel CDs through modifying surface and doping, as well as their applications in in vitro and in vivo imaging. Despite the great progress gained within a short period, the fundamental mechanism and chronic toxicity of CDs in clinical applications are still needed to be investigated.
First of all, there is no doubt that many modification methods of CDs have been developed to improve the targeting ability of CDs in various cells or subcellular cells for their outstanding imaging properties including sensitivity, efficiency and delivery. However, further exploitation and investigations of CDs conjugated with new functional groups or target molecules on their surface would help design a multifunctional platform for specific cell type targeting, specific cancer diagnosis and therapy, and nervous system disease treatment applications. Furthermore, CDs with strong FL emissions in the NIR spectral regions or up-conversion FL and strong BBB penetrating ability are highly desired and need to be further developed. Even through excellent biocompatibility of CDs has been reported in various studies that were conducted on different cell lines, in mice or zebrafish, the careful and systematical evaluation of CDs’ toxicity is urgently needed for clinical diagnosis and treatments. Moreover, the mechanism of CDs interacting with stem cells and neurons has not been fully understood so far, thus, more work is needed to be done in future. The aspects discussed above will provide reliable basis for effective preparation of CDs for specific biomedical applications. Meanwhile, the applications of CD-based nanoprobes in bioimaging-guided diagnosis of cancer and nervous diseases need to be further explored. New therapy methods that combine CDs with therapeutic agents such as anticancer drugs, photodynamic therapy agents and photothermal therapy agents are expected to improve cancer treatment efficacy.
Conflicts of interest
The authors declare no competing financial interest.
Acknowledgements
Dr Xu Yan acknowledge the financial support from the National Nature Science Foundation of China (No. 61831011, 61833006, 31901777, and 21806051), the Science and Technology Development Program of Jilin Province (No. 2017052016JH), the fundamental Research Funds for the Central Universities, and the national Postdoctoral Program for Innovative Talents (BX201700096). The authors would like to acknowledge Dr Suiqiong Li for editing the manuscript.
References
- S. F. Zhang, C. Ma and M. Chalfie, Cell, 2004, 119, 137–144 CrossRef CAS PubMed.
- S. J. Remington, Protein Sci., 2011, 20, 1509–1519 CrossRef CAS.
- Y. Fu, J. Zhang and J. R. Lakowicz, Biochem. Biophys. Res. Commun., 2008, 376, 712–717 CrossRef CAS PubMed.
- X. Xu, R. Ray, Y. Gu, H. J. Ploehn, L. Gearheart, K. Raker and W. A. Scrivens, J. Am. Chem. Soc., 2004, 126, 12736–12737 CrossRef CAS.
- P. Miao, K. Han, Y. G. Tang, B. D. Wang, T. Lin and W. B. Cheng, Nanoscale, 2015, 7, 1586–1595 RSC.
- L. Cao, M. J. Meziani, S. Sahu and Y. P. Sun, Acc. Chem. Res., 2013, 46, 171–180 CrossRef CAS.
- I. Y. Goryacheva, A. V. Sapelkin and G. B. Sukhorukov, TrAC, Trends Anal. Chem., 2017, 90, 27–37 CrossRef CAS.
- F. L. Yuan, S. H. Li, Z. T. Fan, X. Y. Meng, L. Z. Fan and S. H. Yang, Nano Today, 2016, 11, 565–586 CrossRef CAS.
- M. Han, S. J. Zhu, S. Y. Lu, Y. B. Song, T. L. Feng, S. Y. Tao, J. J. Liu and B. Yang, Nano Today, 2018, 19, 201–218 CrossRef CAS.
- N. Nimi, A. Saraswathy, S. S. Nazeer, N. Francis, S. J. Shenoy and R. S. Jayasree, Biomaterials, 2018, 171, 46–56 CrossRef CAS.
- L. L. Lu, C. C. Feng, J. Xu, F. Y. Wang, H. J. Yu, Z. A. Xu and W. Zhang, Biosens. Bioelectron., 2017, 92, 101–108 CrossRef CAS.
- M. X. Gao, L. Yang, Y. Zheng, X. X. Yang, H. Y. Zou, J. Han, Z. X. Liu, Y. F. Li and C. Z. Huang, Chem. – Eur. J., 2017, 23, 2171–2178 CrossRef CAS.
- B. Zhi, Y. Cui, S. Y. Wang, B. P. Frank, D. N. Williams, R. P. Brown, E. S. Melby, R. J. Hamers, Z. Rosenzweig, D. H. Fairbrother, G. Orr and C. L. Haynes, ACS Nano, 2018, 12, 5741–5752 CrossRef CAS.
- S. Hamd-Ghadareh, A. Salimi, F. Fathi and S. Bahrami, Biosens. Bioelectron., 2017, 96, 308–316 CrossRef CAS.
- Q. Liu, B. D. Guo, Z. Y. Rao, B. H. Zhang and J. R. Gong, Nano Lett., 2013, 13, 2436–2441 CrossRef CAS.
- S. B. Ruan, J. Qian, S. Shen, J. H. Zhu, X. G. Jiang, Q. He and H. L. Gao, Nanoscale, 2014, 6, 10040–10047 RSC.
- J. Zhang and S. H. Yu, Mater. Today, 2016, 19, 382–393 CrossRef CAS.
- D. K. Khajuria, V. B. Kumar, D. Karasik and A. Gedanken, ACS Appl. Mater. Interfaces, 2017, 9, 18557–18565 CrossRef CAS.
- L. Wang, B. Q. Li, F. Xu, Y. Li, Z. H. Xu, D. Q. Wei, Y. J. Feng, Y. M. Wang, D. C. Jia and Y. Zhou, Biomaterials, 2017, 145, 192–206 CrossRef CAS.
- X. A. Miao, X. L. Yan, D. Qu, D. B. Li, F. F. Tao and Z. C. Sun, ACS Appl. Mater. Interfaces, 2017, 9, 18549–18556 CrossRef CAS PubMed.
- X. Peng, R. Wang, T. J. Wang, W. N. Yang, H. Wang, W. Gu and L. Ye, ACS Appl. Mater. Interfaces, 2018, 10, 1084–1092 CrossRef CAS.
- P. C. Hsu and H. T. Chang, Chem. Commun., 2012, 48, 3984–3986 RSC.
- S. Liu, J. Q. Tian, L. Wang, Y. W. Zhang, X. Y. Qin, Y. L. Luo, A. M. Asiri, A. O. Al-Youbi and X. P. Sun, Adv. Mater., 2012, 24, 2037–2041 CrossRef CAS.
- M. Y. Xue, J. J. Zhao, Z. H. Zhan, S. L. Zhao, C. Q. Lan, F. G. Ye and H. Liang, Nanoscale, 2018, 10, 18124–18130 RSC.
- Y. Y. Yao, G. Gedda, W. M. Girma, C. L. Yen, Y. C. Ling and J. Y. Chang, ACS Appl. Mater. Interfaces, 2017, 9, 13887–13899 CrossRef CAS.
- R. Atchudan, T. N. J. I. Edison, K. R. Aseer, S. Perumal, N. Karthik and Y. R. Lee, Biosens. Bioelectron., 2018, 99, 303–311 CrossRef CAS.
- Y. Song, X. Yan, Z. H. Li, L. B. Qu, C. Z. Zhu, R. F. Ye, S. Q. Li, D. Du and Y. H. Lin, J. Mater. Chem. B, 2018, 6, 3181–3187 RSC.
- M. L. Liu, L. Yang, R. S. Li, B. B. Chen, H. Liu and C. Z. Huang, Green Chem., 2017, 19, 3611–3617 RSC.
- P. Roy, P. C. Chen, A. P. Periasamy, Y. N. Chen and H. T. Chang, Mater. Today, 2015, 18, 447–458 CrossRef CAS.
- X. T. Zheng, A. Ananthanarayanan, K. Q. Luo and P. Chen, Small, 2015, 11, 1620–1636 CrossRef CAS.
- S. J. Zhu, Y. B. Song, X. H. Zhao, J. R. Shao, J. H. Zhang and B. Yang, Nano Res., 2015, 8, 355–381 CrossRef CAS.
- A. Sciortino, E. Marino, B. van Dam, P. Schall, M. Cannas and F. Messina, J. Phys. Chem. Lett., 2016, 7, 3419–3423 CrossRef CAS.
- S. Y. Tao, S. J. Zhu, T. L. Feng, C. L. Xia, Y. B. Song and B. Yang, Mater. Today Chem., 2017, 6, 13–25 CrossRef.
- S. Y. Lim, W. Shen and Z. Q. Gao, Chem. Soc. Rev., 2015, 44, 362–381 RSC.
- X. C. Sun and Y. Lei, TrAC, Trends Anal. Chem., 2017, 89, 163–180 CrossRef CAS.
- H. J. Zhang, B. X. Zhang, C. X. Di, M. C. Ali, J. Chen, Z. Li, J. Si, H. Zhang and H. D. Qiu, Nanoscale, 2018, 10, 5342–5349 RSC.
- Z. L. Peng, X. Han, S. H. Li, A. O. Al-Youbi, A. S. Bashammakh, M. S. El-Shahawi and R. M. Leblanc, Coord. Chem. Rev., 2017, 343, 256–277 CrossRef CAS.
- Z. J. Zhu, Y. L. Zhai, Z. H. Li, P. Y. Zhu, S. Mao, C. Z. Zhu, D. Du, L. A. Belfiore, J. G. Tang and Y. H. Lin, Mater. Today, 2019 DOI:10.1016/j.mattod.2019.05.003.
- P. C. Hsu, Z. Y. Shih, C. H. Lee and H. T. Chang, Green Chem., 2012, 14, 917–920 RSC.
- V. Ramanan, S. K. Thiyagarajan, K. Raji, R. Suresh, R. Sekar and P. Ramamurthy, ACS Sustainable Chem. Eng., 2016, 4, 4724–4731 CrossRef CAS.
- X. Yan, Y. Song, C. Z. Zhu, H. X. Li, D. Du, X. G. Su and Y. H. Lin, Anal. Chem., 2018, 90, 2618–2624 CrossRef CAS.
- V. Nguyen, J. H. Si, L. H. Yan and X. Hou, Carbon, 2016, 108, 268–273 CrossRef CAS.
- S. Mukherjee, E. Prasad and A. Chadha, Phys. Chem. Chem. Phys., 2017, 19, 7288–7296 RSC.
- S. Kim, S. W. Hwang, M. K. Kim, D. Y. Shin, D. H. Shin, C. O. Kim, S. B. Yang, J. H. Park, E. Hwang, S. H. Choi, G. Ko, S. Sim, C. Sone, H. J. Choi, S. Bae and B. H. Hong, ACS Nano, 2012, 6, 8203–8208 CrossRef CAS PubMed.
- H. Tetsuka, R. Asahi, A. Nagoya, K. Okamoto, I. Tajima, R. Ohta and A. Okamoto, Adv. Mater., 2012, 24, 5333–5338 CrossRef CAS.
- Y. Y. Wang, Y. Li, Y. Yan, J. Xu, B. Y. Guan, Q. Wang, J. Y. Li and J. H. Yu, Chem. Commun., 2013, 49, 9006–9008 RSC.
- P. W. Gong, L. Sun, F. Wang, X. C. Liu, Z. Q. Yan, M. Z. Wang, L. Zhang, Z. Z. Tian, Z. Liu and J. M. You, Chem. Eng. J., 2019, 356, 994–1002 CrossRef CAS.
- X. Ye, Y. Xiang, Q. Wang, Z. Li and Z. Liu, Small, 2019, 1901673 CrossRef.
- Y. Zhan, T. Geng, Y. Liu, C. Hu, X. Zhang, B. Lei, J. Zhuang, X. Wu, D. Huang, G. Xiao and B. Zou, ACS Appl. Mater. Interfaces, 2018, 10, 27920–27927 CrossRef CAS.
- H. Wang, J. Di, Y. B. Sun, J. P. Fu, Z. Y. Wei, H. Matsui, A. D. Alonso and S. Q. Zhou, Adv. Funct. Mater., 2015, 25, 5537–5547 CrossRef CAS.
- H. Wang, C. Sun, X. R. Chen, Y. Zhang, V. L. Colvin, Q. Rice, J. Seo, S. Y. Feng, S. N. Wang and W. W. Yu, Nanoscale, 2017, 9, 1909–1915 RSC.
- L. Shi, J. H. Yang, H. B. Zeng, Y. M. Chen, S. C. Yang, C. Wu, H. Zeng, O. Yoshihito and Q. Q. Zhang, Nanoscale, 2016, 8, 14374–14378 RSC.
- C. X. Huang, H. L. Dong, Y. Su, Y. Wu, R. Narron and Q. Yong, Nanomaterials, 2019, 9, 387 CrossRef CAS.
- M. Zheng, Y. Li, S. Liu, W. Q. Wang, Z. G. Xie and X. B. Jing, ACS Appl. Mater. Interfaces, 2016, 8, 23533–23541 CrossRef CAS.
- H. Ding, S. B. Yu, J. S. Wei and H. M. Xiong, ACS Nano, 2016, 10, 484–491 CrossRef CAS.
- X. H. Wang, K. G. Qu, B. L. Xu, J. S. Ren and X. G. Qu, Nano Res., 2011, 4, 908–920 CrossRef CAS.
- A. D. Zhao, Z. W. Chen, C. Q. Zhao, N. Gao, J. S. Ren and X. G. Qu, Carbon, 2015, 85, 309–327 CrossRef CAS.
- D. W. Zhang, N. Papaioannou, N. M. David, H. Luo, H. Gao, L. C. Tanase, T. Degousee, P. Samori, A. Sapelkin, O. Fenwick, M. M. Titirici and S. Krause, Mater. Horiz., 2018, 5, 423–428 RSC.
- D. Zhou, Y. C. Zhai, S. N. Qu, D. Li, P. T. Jing, W. Y. Ji, D. Z. Shen and A. L. Rogach, Small, 2017, 13, 1602055 CrossRef PubMed.
- S. H. Li, L. Y. Wang, C. C. Chusuei, V. M. Suarez, P. L. Blackwelder, M. Micic, J. Orbulescu and R. M. Leblanc, Chem. Mater., 2015, 27, 1764–1771 CrossRef CAS.
- J. Zhan, B. J. Geng, K. Wu, G. Xu, L. Wang, R. Y. Guo, B. Lei, F. F. Zheng, D. Y. Pan and M. H. Wu, Carbon, 2018, 130, 153–163 CrossRef CAS.
- Y. L. Shi, A. Pramanik, C. Tchounwou, F. Pedraza, R. A. Crouch, S. R. Chavva, A. Vangara, S. S. Sinha, S. Jones, D. Sardar, C. Hawker and P. C. Ray, ACS Appl. Mater. Interfaces, 2015, 7, 10935–10943 CrossRef CAS.
- J. Zhang, Y. Yuan, G. L. Liang and S. H. Yu, Adv. Sci., 2015, 2, 1500002 CrossRef PubMed.
- L. L. Zhang, Y. J. Han, J. B. Zhu, Y. L. Zhai and S. J. Dong, Anal. Chem., 2015, 87, 2033–2036 CrossRef CAS PubMed.
- L. B. Li, B. Yu and T. Y. You, Biosens. Bioelectron., 2015, 74, 263–269 CrossRef CAS PubMed.
- D. Mosconi, D. Mazzier, S. Silvestrini, A. Privitera, C. Marega, L. Franco and A. Moretto, ACS Nano, 2015, 9, 4156–4164 CrossRef CAS.
- M. C. Ortega-Liebana, N. X. Chung, R. Limpens, L. Gomez, J. L. Hueso, J. Santamaria and T. Gregorkiewicz, Carbon, 2017, 117, 437–446 CrossRef CAS.
- Z. G. Gu, D. J. Li, C. Zheng, Y. Kang, C. Woll and J. Zhang, Angew. Chem., Int. Ed., 2017, 56, 6853–6858 CrossRef CAS PubMed.
- J. Wang, C. F. Wang and S. Chen, Angew. Chem., Int. Ed., 2012, 51, 9297–9301 CrossRef CAS.
- X. L. Wu, Y. Song, X. Yan, C. Z. Zhu, Y. Q. Ma, D. Du and Y. H. Lin, Biosens. Bioelectron., 2017, 94, 292–297 CrossRef CAS PubMed.
- M. Havrdova, K. Hola, J. Skopalik, K. Tomankova, P. A. Martin, K. Cepe, K. Polakova, J. Tucek, A. B. Bourlinos and R. Zboril, Carbon, 2016, 99, 238–248 CrossRef CAS.
- S. Y. Park, C. Y. Lee, H. R. An, H. Kim, Y. C. Lee, E. C. Park, H. S. Chun, H. Y. Yang, S. H. Choi, H. S. Kim, K. S. Kang, H. G. Park, J. P. Kim, Y. Choi, J. Lee and H. U. Lee, Nanoscale, 2017, 9, 9210–9217 RSC.
- Y. Song, C. Z. Zhu, J. H. Song, H. Li, D. Du and Y. H. Lin, ACS Appl. Mater. Interfaces, 2017, 9, 7399–7405 CrossRef CAS PubMed.
- G. Gao, Y. W. Jiang, H. R. Jia, J. J. Yang and F. G. Wu, Carbon, 2018, 134, 232–243 CrossRef CAS.
- X. Yan, Y. Song, C. Z. Zhu, J. H. Song, D. Du, X. G. Su and Y. H. Lin, ACS Appl. Mater. Interfaces, 2016, 8, 21990–21996 CrossRef CAS PubMed.
- S. T. Yang, L. Cao, P. G. J. Luo, F. S. Lu, X. Wang, H. F. Wang, M. J. Meziani, Y. F. Liu, G. Qi and Y. P. Sun, J. Am. Chem. Soc., 2009, 131, 11308–11309 CrossRef CAS.
- H. Q. Tao, K. Yang, Z. Ma, J. M. Wan, Y. J. Zhang, Z. H. Kang and Z. Liu, Small, 2012, 8, 281–290 CrossRef CAS.
- C. S. Ke, C. C. Fang, J. Y. Yan, P. J. Tseng, J. R. Pyle, C. P. Chen, S. Y. Lin, J. X. Chen, X. J. Zhang and Y. H. Chan, ACS Nano, 2017, 11, 3166–3177 CrossRef CAS PubMed.
- Z. L. Peng, E. H. Miyanji, Y. Q. Zhou, J. Pardo, S. D. Hettiarachchi, S. H. Li, P. L. Blackwelder, I. Skromne and R. M. Leblanc, Nanoscale, 2017, 9, 17533–17543 RSC.
- W. L. Gao, H. H. Song, X. Wang, X. Liu, X. B. Pang, Y. Zhou, B. Gao and X. J. Peng, ACS Appl. Mater. Interfaces, 2018, 10, 1147–1154 CrossRef CAS.
- X. L. Huang, F. Zhang, L. Zhu, K. Y. Choi, N. Guo, J. X. Guo, K. Tackett, P. Anilkumar, G. Liu, Q. M. Quan, H. S. Choi, G. Niu, Y. P. Sun, S. Lee and X. Y. Chen, ACS Nano, 2013, 7, 5684–5693 CrossRef CAS PubMed.
- F. Y. Du, L. R. Zhang, L. Zhang, M. M. Zhang, A. H. Gong, Y. W. Tan, J. W. Miao, Y. H. Gong, M. Z. Sun, H. X. Ju, C. Y. Wu and S. Q. Zou, Biomaterials, 2017, 121, 109–120 CrossRef CAS.
- G. Lemenager, E. De Luca, Y. P. Sun and P. P. Pompa, Nanoscale, 2014, 6, 8617–8623 RSC.
- B. Das, P. Dadhich, P. Pal and S. Dhara, RSC Adv., 2016, 6, 60794–60805 RSC.
- Y. H. Chien, K. K. Chan, S. H. K. Yap and K. T. Yong, J. Chem. Technol. Biotechnol., 2018, 93, 1519–1528 CrossRef CAS.
- M. M. Al Awak, P. Wang, S. Y. Wang, Y. A. Tang, Y. P. Sun and L. J. Yang, RSC Adv., 2017, 7, 30177–30184 RSC.
- N. Kaur, V. Sharma, P. Tiwari, A. K. Saini and S. M. Mobin, Sens. Actuators, B, 2019, 291, 275–286 CrossRef CAS.
- E. Priyadarshini, K. Rawat, T. Prasad and H. B. Bohidar, Colloids Surf., B, 2018, 163, 355–361 CrossRef CAS PubMed.
- P. Kumarathasan, D. Breznan, D. Das, M. A. Salam, Y. Siddiqui, C. MacKinnon-Roy, J. W. Guan, N. de Silva, B. Simard and R. Vincent, Nanotoxicology, 2015, 9, 148–161 CrossRef CAS PubMed.
- S. H. Ouyang, X. G. Hu and Q. X. Zhou, ACS Appl. Mater. Interfaces, 2015, 7, 18104–18112 CrossRef CAS PubMed.
- B. Q. Luan, T. Huynh, L. Zhao and R. H. Zhou, ACS Nano, 2015, 9, 663–669 CrossRef CAS PubMed.
- M. Chen, S. Zhou, Y. Zhu, Y. Z. Sun, G. M. Zeng, C. P. Yang, P. Xu, M. Yan, Z. F. Liu and W. Zhang, Chemosphere, 2018, 206, 255–264 CrossRef CAS PubMed.
- A. Dhawan and V. Sharma, Anal. Bioanal. Chem., 2010, 398, 589–605 CrossRef CAS PubMed.
- W. Li, Y. J. Zheng, H. R. Zhang, Z. L. Liu, W. Su, S. Chen, Y. L. Liu, J. L. Zhuang and B. F. Lei, ACS Appl. Mater. Interfaces, 2016, 8, 19939–19945 CrossRef CAS PubMed.
- J. Chen, R. Z. Dou, Z. Z. Yang, X. P. Wang, C. B. Mao, X. Gao and L. Wang, Nanotoxicology, 2016, 10, 818–828 CrossRef CAS PubMed.
- H. J. Zhao, J. K. Duan, Y. C. Xiao, G. H. Tang, C. G. Wu, Y. Zhang, Z. H. Liu and W. Xue, Chem. Mater., 2018, 30, 3438–3453 CrossRef CAS.
- B. J. Geng, D. W. Yang, D. Y. Pan, L. Wang, F. F. Zheng, W. W. Shen, C. Zhang and X. K. Li, Carbon, 2018, 134, 153–162 CrossRef CAS.
- J. Du, N. Xu, J. Fan, W. Sun and X. Peng, Small, 2019, 1805087 CrossRef PubMed.
- N. Zhou, Z. Y. Hao, X. H. Zhao, S. Maharjan, S. J. Zhu, Y. B. Song, B. Yang and L. J. Lu, Nanoscale, 2015, 7, 15635–15642 RSC.
- J. X. Chen, Q. Wang, J. Zhou, W. W. Deng, Q. T. Yu, X. Cao, J. P. Wang, F. X. Shao, Y. Li, P. Ma, M. Spector, J. N. Yu and X. M. Xu, Nanoscale, 2017, 9, 10820–10831 RSC.
- K. K. R. Datta, O. Kozak, V. Ranc, M. Havrdova, A. B. Bourlinos, K. Safarova, K. Hola, K. Tomankova, G. Zoppellaro, M. Otyepka and R. Zboril, Chem. Commun., 2014, 50, 10782–10785 RSC.
- Y. F. Kang, Y. W. Fang, Y. H. Li, W. Li and X. B. Yin, Chem. Commun., 2015, 51, 16956–16959 RSC.
- X. W. Hua, Y. W. Bao, Z. Chen and F. G. Wu, Nanoscale, 2017, 9, 10948–10960 RSC.
- F. L. Yuan, L. Ding, Y. C. Li, X. H. Li, L. Z. Fan, S. X. Zhou, D. C. Fang and S. H. Yang, Nanoscale, 2015, 7, 11727–11733 RSC.
- Z. T. Fan, Y. C. Li, X. H. Li, L. Z. Fan, S. X. Zhou, D. C. Fang and S. H. Yang, Carbon, 2014, 70, 149–156 CrossRef CAS.
- K. Jiang, S. Sun, L. Zhang, Y. Lu, A. G. Wu, C. Z. Cai and H. W. Lin, Angew. Chem., Int. Ed., 2015, 54, 5360–5363 CrossRef CAS PubMed.
- K. Jiang, S. Sun, L. Zhang, Y. H. Wang, C. Z. Cai and H. W. Lin, ACS Appl. Mater. Interfaces, 2015, 7, 23231–23238 CrossRef CAS PubMed.
- H. K. Sadhanala and K. K. Nanda, Carbon, 2016, 96, 166–173 CrossRef CAS.
- L. H. Shi, Y. Y. Li, X. F. Li, X. P. Wen, G. M. Zhang, J. Yang, C. Dong and S. M. Shuang, Nanoscale, 2015, 7, 7394–7401 RSC.
- N. Wang, A. Q. Zheng, X. Liu, J. J. Chen, T. Yang, M. Chen and J. H. Wang, ACS Appl. Mater. Interfaces, 2018, 10, 7901–7909 CrossRef CAS PubMed.
- S. B. Ruan, J. Qian, S. Shen, J. T. Chen, J. H. Zhu, X. G. Jiang, Q. He, W. L. Yang and H. L. Gao, Bioconjugate Chem., 2014, 25, 2252–2259 CrossRef CAS PubMed.
- H. X. Li, X. Yan, S. P. Qiao, G. Y. Lu and X. G. Su, ACS Appl. Mater. Interfaces, 2018, 10, 7737–7744 CrossRef CAS PubMed.
- B. Demir, M. M. Lemberger, M. Panagiotopoulou, P. X. M. Rangel, S. Timur, T. Hirsch, B. T. S. Bui, J. Wegener and K. Haupt, ACS Appl. Mater. Interfaces, 2018, 10, 3305–3313 CrossRef CAS PubMed.
- D. J. Prockop, Science, 2001, 293, 211–212 CrossRef CAS PubMed.
- J. Kaslin, V. Kroehne, J. Ganz, S. Hans and M. Brand, Development, 2017, 144, 3388 CrossRef PubMed.
- T. Kim, J. E. Lemaster, F. Chen, J. Li and J. V. Jokerst, ACS Nano, 2017, 11, 9022–9032 CrossRef CAS PubMed.
- H. Lin, J. Sohn, H. Shen, M. T. Langhans and R. S. Tuan, Biomaterials, 2019, 203, 96–110 CrossRef CAS PubMed.
- K. A. U. Gonzales and E. Fuchs, Dev. Cell, 2017, 43, 387–401 CrossRef CAS PubMed.
- L. P. Nucci, H. R. Silva, V. Giampaoli, J. B. Mamani, M. P. Nucci and L. F. Gamarra, Stem Cell Res. Ther., 2015, 6, 27 CrossRef PubMed.
- W. Shang, X. Zhang, M. Zhang, Z. Fan, Y. Sun, M. Han and L. Fan, Nanoscale, 2014, 6, 5799–5806 RSC.
- M. Zhang, L. L. Bai, W. H. Shang, W. J. Xie, H. Ma, Y. Y. Fu, D. C. Fang, H. Sun, L. Z. Fan, M. Han, C. M. Liu and S. H. Yang, J. Mater. Chem., 2012, 22, 7461–7467 RSC.
- J. H. Liu, L. Cao, G. E. LeCroy, P. Wang, M. J. Meziani, Y. Y. Dong, Y. F. Liu, P. J. G. Luo and Y. P. Sun, ACS Appl. Mater. Interfaces, 2015, 7, 19439–19445 CrossRef CAS PubMed.
- D. Shao, M. M. Lu, D. Xu, X. Zheng, Y. Pan, Y. B. Song, J. Y. Xu, M. Q. Li, M. Zhang, J. Li, G. F. Chi, L. Chen and B. Yang, Biomater. Sci., 2017, 5, 1820–1827 RSC.
- M. S. Khan, S. Pandey, A. Talib, M. L. Bhaisare and H. F. Wu, Colloids Surf., B, 2015, 134, 140–146 CrossRef CAS PubMed.
- I. Nissan, V. B. Kumar, Z. Porat, D. Makovec, O. Shefi and A. Gedanken, J. Mater. Chem. B, 2017, 5, 1371–1379 RSC.
- T. Gao, S. L. Wang, W. W. Lv, M. A. Liu, H. L. Zeng, Z. Chen, J. Dong, Z. P. Wu, X. P. Feng and W. B. Zeng, Chem. Commun., 2018, 54, 3578–3581 RSC.
- S. Khan, N. C. Verma, Chethana and C. K. Nandi, ACS Appl. Nano Mater., 2018, 1, 483–487 CrossRef CAS.
- H. F. Liu, J. Yang, Z. H. Li, L. H. Xiao, A. A. Aryee, Y. Q. Sun, R. Yang, H. M. Meng, L. B. Qu, Y. H. Lin and X. B. Zhang, Anal. Chem., 2019, 91, 9259–9265 CrossRef CAS PubMed.
- L. Yang, Z. R. Wang, J. Wang, W. H. Jiang, X. W. Jiang, Z. S. Bai, Y. P. He, J. Q. Jiang, D. K. Wang and L. Yang, Nanoscale, 2016, 8, 6801–6809 RSC.
- L. Q. Wang, X. Y. Wang, A. Bhirde, J. B. Cao, Y. Zeng, X. L. Huang, Y. P. Sun, G. Liu and X. Y. Chen, Adv. Healthcare Mater., 2014, 3, 1203–1209 CrossRef CAS PubMed.
- X. W. Hua, Y. W. Bao and F. G. Wee, ACS Appl. Mater. Interfaces, 2018, 10, 16924 CrossRef CAS PubMed.
- X. Guo, X. Y. Sun, D. Hu, Y. J. Wang, H. Fujioka, R. Vyas, S. Chakrapani, A. U. Joshi, Y. Luo, D. Mochly-Rosen and X. Qi, Nat. Commun., 2016, 7, 12646 CrossRef CAS PubMed.
- A. M. Kabat and E. J. Pearce, Science, 2017, 356, 488–489 CrossRef CAS PubMed.
- W. X. Zong, J. D. Rabinowitz and E. White, Mol. Cell, 2016, 61, 667–676 CrossRef CAS PubMed.
- C. Lopez-Crisosto, C. Pennanen, C. Vasquez-Trincado, P. Morales, R. Bravo-Sagua, A. F. G. Quest, M. Chiong and S. Lavandero, Nat. Rev. Cardiol., 2017, 14, 342–360 CrossRef CAS.
- X. X. Wu, S. Sun, Y. H. Wang, J. L. Zhu, K. Jiang, Y. M. Leng, Q. H. Shu and H. W. Lin, Biosens. Bioelectron., 2017, 90, 501–507 CrossRef CAS.
- F. K. Du, Y. H. Min, F. Zeng, C. M. Yu and S. Z. Wu, Small, 2014, 10, 964–972 CrossRef CAS.
- F. F. Yu, Z. Y. Chen, B. L. Wang, Z. Jin, Y. F. Hou, S. M. Ma and X. D. Liu, Tumor Biol., 2016, 37, 1427–1436 CrossRef CAS PubMed.
- A. Ciechanover and Y. T. Kwon, Exp. Mol. Med., 2015, 47, e147 CrossRef CAS PubMed.
- L. A. Huber and D. Teis, Curr. Opin. Cell Biol., 2016, 39, 8–14 CrossRef CAS PubMed.
- Q. Q. Zhang, T. Yang, R. S. Li, H. Y. Zou, Y. F. Li, J. Guo, X. D. Liu and C. Z. Huang, Nanoscale, 2018, 10, 14705–14711 RSC.
- L. L. Wu, X. L. Li, Y. F. Ling, C. S. Huang and N. Q. Jia, ACS Appl. Mater. Interfaces, 2017, 9, 28222–28232 CrossRef CAS PubMed.
- H. F. Liu, Y. Q. Sun, Z. H. Li, J. Yang, A. A. Aryee, L. B. Qu, D. Du and Y. H. Lin, Nanoscale, 2019, 11, 8458–8463 RSC.
- E. Shuang, Q. X. Mao, X. L. Yuan, X. L. Kong, X. W. Chen and J. H. Wang, Nanoscale, 2018, 10, 12788–12796 RSC.
- K. D. Wegner and N. Hildebrandt, Chem. Soc. Rev., 2015, 44, 4792–4834 RSC.
- W. J. Zhao, Y. H. Li, S. Yang, Y. Chen, J. Zheng, C. H. Liu, Z. H. Qing, J. S. Li and R. H. Yang, Anal. Chem., 2016, 88, 4833–4840 CrossRef CAS.
- Y. F. Kong, J. Chen, H. W. Fang, G. Heath, Y. Wo, W. L. Wang, Y. X. Li, Y. Guo, S. D. Evans, S. Y. Chen and D. J. Zhou, Chem. Mater., 2016, 28, 3041–3050 CrossRef CAS PubMed.
- G. Mendoza, I. O. de Solorzano, I. Pintre, S. Garcia-Salinas, V. Sebastian, V. Andreu, M. Gimeno and M. Arruebo, Nanoscale, 2018, 10, 2970–2982 RSC.
- J. P. Li, S. W. Yang, Y. Deng, P. W. Chai, Y. C. Yang, X. Y. He, X. M. Xie, Z. H. Kang, G. Q. Ding, H. F. Zhou and X. Q. Fan, Adv. Funct. Mater., 2018, 28, 1800881 CrossRef.
- N. Licciardello, S. Hunoldt, R. Bergmann, G. Singh, C. Mamat, A. Faramus, J. L. Z. Ddungu, S. Silvestrini, M. Maggini, L. De Cola and H. Stephan, Nanoscale, 2018, 10, 9880–9891 RSC.
- Y. Wang, Y. Meng, S. S. Wang, C. Y. Li, W. Shi, J. Chen, J. X. Wang and R. Q. Huang, Small, 2015, 11, 3575–3581 CrossRef CAS PubMed.
- R. Xu, G. D. Zhang, J. H. Mai, X. Y. Deng, V. Segura-Ibarra, S. H. Wu, J. L. Shen, H. R. Liu, Z. H. Hu, L. X. Chen, Y. Huang, E. Koay, Y. Huang, J. Liu, J. E. Ensor, E. Blanco, X. W. Liu, M. Ferrari and H. F. Shen, Nat. Biotechnol., 2016, 34, 414–418 CrossRef CAS PubMed.
- L. Y. Liao, J. Liu, E. C. Dreaden, S. W. Morton, K. E. Shopsowitz, P. T. Hammond and J. A. Johnson, J. Am. Chem. Soc., 2014, 136, 5896–5899 CrossRef CAS PubMed.
- J. Tang, B. Kong, H. Wu, M. Xu, Y. C. Wang, Y. L. Wang, D. Y. Zhao and G. F. Zheng, Adv. Mater., 2013, 25, 6569–6574 CrossRef CAS.
- T. Feng, X. Z. Ai, G. H. An, P. P. Yang and Y. L. Zhao, ACS Nano, 2016, 10, 5587 CrossRef CAS.
- M. Zheng, S. Liu, J. Li, D. Qu, H. F. Zhao, X. G. Guan, X. L. Hu, Z. G. Xie, X. B. Jing and Z. C. Sun, Adv. Mater., 2014, 26, 3554–3560 CrossRef CAS PubMed.
- Y. Shu, J. Lu, Q. X. Mao, R. S. Song, X. Y. Wang, X. W. Chen and J. H. Wang, Carbon, 2017, 114, 324–333 CrossRef CAS.
- X. J. Gong, Q. Y. Zhang, Y. F. Gao, S. M. Shuang, M. M. F. Choi and C. Dong, ACS Appl. Mater. Interfaces, 2016, 8, 11288–11297 CrossRef CAS PubMed.
- M. Zhang, W. T. Wang, N. L. Zhou, P. Yuan, Y. T. Su, M. N. Shao, C. Chi and F. Y. Pan, Carbon, 2017, 118, 752–764 CrossRef CAS.
- N. Gao, W. Yang, H. L. Nie, Y. Q. Gong, J. Jing, L. J. Gao and X. L. Zhang, Biosens. Bioelectron., 2017, 96, 300–307 CrossRef CAS PubMed.
- D. Li, P. T. Jing, L. H. Sun, Y. An, X. Y. Shan, X. H. Lu, D. Zhou, D. Han, D. Z. Shen, Y. C. Zhai, S. N. Qu, R. Zboril and A. L. Rogach, Adv. Mater., 2018, 30, 1705913 CrossRef PubMed.
- J. C. Ge, Q. Y. Jia, W. M. Liu, L. Guo, Q. Y. Liu, M. H. Lan, H. Y. Zhang, X. M. Meng and P. F. Wang, Adv. Mater., 2015, 27, 4169–4177 CrossRef CAS PubMed.
- J. C. Ge, Q. Y. Jia, W. M. Liu, M. H. Lan, B. J. Zhou, L. Guo, H. Y. Zhou, H. Y. Zhang, Y. Wang, Y. Gu, X. M. Meng and P. F. Wang, Adv. Healthcare Mater., 2016, 5, 665–675 CrossRef CAS PubMed.
- S. N. Qu, X. Y. Wang, Q. P. Lu, X. Y. Liu and L. J. Wang, Angew. Chem., Int. Ed., 2012, 51, 12215–12218 CrossRef CAS.
- H. Peng, Y. Li, C. L. Jiang, C. H. Luo, R. J. Qi, R. Huang, C. G. Duan and J. Travas-Sejdic, Carbon, 2016, 100, 386–394 CrossRef CAS.
- D. W. Zheng, B. Li, C. X. Li, J. X. Fan, Q. Lei, C. Li, Z. S. Xu and X. Z. Zhang, ACS Nano, 2016, 10, 8715–8722 CrossRef CAS PubMed.
- W. N. Yang, H. Zhang, J. X. Lai, X. Y. Peng, Y. P. Hu, W. Gu and L. Ye, Carbon, 2018, 128, 78–85 CrossRef CAS.
- H. Wang, S. Mukherjee, J. H. Ji, P. Banerjee, Q. W. Chen and S. Q. Zhou, ACS Appl. Mater. Interfaces, 2017, 9, 18639–18649 CrossRef CAS PubMed.
- X. L. Guo, Z. Y. Ding, S. M. Deng, C. C. Wen, X. C. Shen, B. P. Jiang and H. Liang, Carbon, 2018, 134, 519–530 CrossRef CAS.
- W. Q. Li, Z. G. Wang, S. J. Hao, L. P. Sun, M. Nisic, G. Cheng, C. D. Zhu, Y. Wan, L. Ha and S. Y. Zheng, Nanoscale, 2018, 10, 3744–3752 RSC.
- Q. Y. Jia, J. C. Ge, W. M. Liu, X. L. Zheng, S. Q. Chen, Y. M. Wen, H. Y. Zhang and P. F. Wang, Adv. Mater., 2018, 30, 1706090 CrossRef PubMed.
- A. Singh, W. Kim, Y. Kim, K. Jeong, C. S. Kang, Y. Kim, J. Koh, S. D. Mahajan, P. N. Prasad and S. Kim, Adv. Funct. Mater., 2016, 26, 7057–7066 CrossRef CAS PubMed.
- M. Qian, Y. Du, S. S. Wang, C. Y. Li, H. L. Jiang, W. Shi, J. Chen, Y. Wang, E. Wagner and R. Q. Huang, ACS Appl. Mater. Interfaces, 2018, 10, 4031–4040 CrossRef CAS PubMed.
- M. Zheng, S. B. Ruan, S. Liu, T. T. Sun, D. Qu, H. F. Zhao, Z. G. Xie, H. L. Gao, X. B. Jing and Z. C. Sun, ACS Nano, 2015, 9, 11455–11461 CrossRef CAS PubMed.
- S. S. Lu, S. S. Guo, P. X. Xu, X. R. Li, Y. M. Zhao, W. Gu and M. Xue, Int. J. Nanomed., 2016, 11, 6325–6336 CrossRef CAS PubMed.
- T. Borisova, A. Nazarova, M. Dekaliuk, N. Krisanova, N. Pozdnyakova, A. Borysov, R. Sivko and A. P. Demchenko, Int. J. Biochem. Cell Biol., 2015, 59, 203–215 CrossRef CAS PubMed.
Footnote |
† These authors contributed equally to this work. |
|
This journal is © The Royal Society of Chemistry 2020 |
Click here to see how this site uses Cookies. View our privacy policy here.