DOI:
10.1039/D0NA00304B
(Communication)
Nanoscale Adv., 2020,
2, 2720-2725
Beam generation and structural optimization of size-selected Au923 clusters
Received
17th April 2020
, Accepted 18th May 2020
First published on 18th May 2020
Abstract
A size-selected beam of Au923±20 clusters is generated in a gas-phase condensation cluster source equipped with a lateral time-of-flight mass selector. The beam current reaches up to 9.13 nA for small clusters and 80 pA for Au923±20 clusters, which are then analyzed using a scanning transmission electron microscope. Four types of metastable structures are observed for the Au923±20 clusters, including ino-decahedron (Dh), cuboctahedron and icosahedron (Ih). The proportion of bulk-favorable cuboctahedron (i.e. face center cubic (Fcc)) structure takes up only 10–20%, while the penta-rotating symmetrical structures (Dh/Ih) are the dominant ones which take up over three quarters. Changing the beam condition may optimize the clusters from Dh-dominant to the Ih-dominant phase, which paves the way towards nanoparticle control beyond the diameters.
Clusters are a series of materials composed of several to hundreds of atoms. The limited atom numbers and large specific surface area indicate that clusters cannot easily form periodical structures and can show numerous other structures.1–3 Finding new structures is the fundamental task of the cluster science, based on which numerous novel properties have been discovered.4,5 One of the attractive element is gold. Due to the strong relativistic effect, the decrease in the energy of the 6s electron and the increase in that of the 5d electrons along with s-d hybridization occur in gold,6,7 playing a key role in the formation of novel structures, such as cages,8 planar structures,7 Dh, Ih5 and tetrahedron.9 The evolution of the cluster structure of gold with respect to size/atomic number,10,11 temperature,12,13 substrates14,15 and even gas environments16 has been studied in detail, but only few studies commit to the directional structure control of these clusters.17 The precise control of the cluster structural transformation with certain atomic numbers and high yield are of great importance for the precise study of properties and further practical applications. Not only catalysis18 but optical nanoclusters19 also require the precise controlled clusters with both atomic number and nanostructure precision.
Au923 is a magic number cluster20 with sufficient yield in our experiment. The structures of the as-prepared Au923 through a magnetron sputtering cluster source in STEM include Dh, Fcc and Ih.21 The direct structure control of such a big cluster from the original Au atoms will be of great importance beyond the size control, which has been achieved for a long time. Here, we study the structural optimization of the size-selected Au923 clusters through our magnetron sputtering cluster source. The original highest proportion of the Dh structure with considerable yield can be conversed to another penta-rotating symmetrical Ih structure with proper conditions; however, the bulk-favorite Fcc structure remains only a little part between 10% and 20%.
A magnetron sputtering gas-phase condensation cluster source equipped with a lateral time-of-flight mass selector22,23 is used for cluster preparation and its schematic is shown in Fig. 1(a). Clusters with a mass resolution of about 4% can be produced. The process of cluster formation is shown in Fig. 1(b). Energetic gold atoms are sputtered out by bombarding the gold target with ionized argon atoms and then cooled down through the multi-body collision with He atoms.24,25 Then, the cooled Au atoms aggregate with each other and gradually grow up. By controlling the collision time we can enrich clusters within a certain atomic number range. The as-prepared clusters finally soft-land on ultrathin carbon films. The morphology and size of the as-synthesized Au923±20 clusters are studied using a spherical-aberration-corrected STEM, carried out on an FEI Themis Z at the National Graphene Center in Wuxi, China. In order to diminish the statistical error for size and structural statistics, over 50 images are collected for each group of clusters under different synthesis conditions. Four types of structures are observed, including Dh, Fcc, Ih and an unknown structure, which cannot be classified due to the blurred image. To classify the structures, the simulated STEM image atlas of each possible structure is obtained. By comparing the simulation and experimental images, the structure of each cluster in the STEM image can be assigned. Except for a normally prepared Au923±20 clusters (group 1) with the highest beam current of about 80 pA, we prepared other three groups of Au923±20 clusters by changing the preparation conditions from group 1, which are shown in Table 1 below with five sets of condensation parameters. We primarily changed the sputtering power, He gas flow and the condensation length while slightly changing Ar gas to sustain a steady and detectable deposition current. The total pressure in the condensation chamber is changed when we change the helium and argon gas flows.
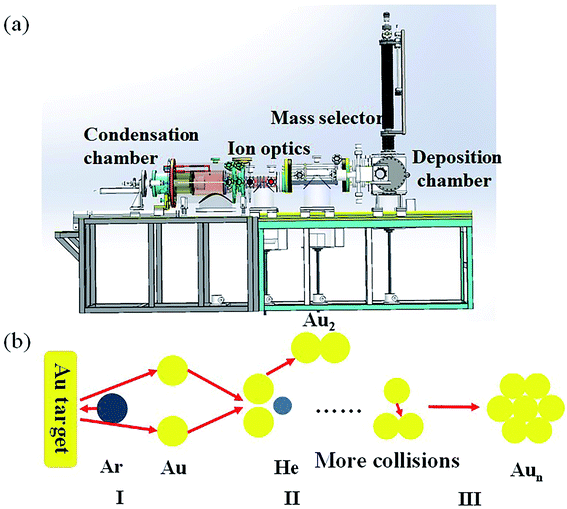 |
| Fig. 1 Schematic of the magnetron sputtering gas-phase condensation cluster source with time-of-flight mass-selector and the formation process of clusters. (a) The schematic of the cluster source. The source is composed of four parts, namely condensation chamber, ion optics, TOF mass selector and the deposition chamber. (b) The formation process of clusters in the condensation chamber. (I) The hot gold atoms (golden) are sputtered out by argon atoms (gray). (II and III) The sputtered atoms are cooled down by multi-body collisions with helium gas (blue) and aggregate with each other. | |
Table 1 The variation of synthesis conditions for the different structure proportions of Au923±20 clusters
Group number |
Sputtering power (W) |
Helium flow (sccm) |
Argon flow (sccm) |
Condensation pressure (Pa) |
Condensation length (cm) |
1 |
20 |
154 |
176 |
54 |
27 |
2 |
60 |
15 |
184 |
17 |
12 |
3 |
80 |
11 |
179 |
16 |
12 |
4 |
120 |
11 |
183 |
17 |
12 |
The yield of the Au923±20 clusters versus the condensation length is shown in Fig. 2(a). The collision times between gold atoms, clusters and helium atoms increases with the condensation length, leading to a higher cluster yield. The mass spectra of small gold clusters and the deposition current of the Au923±20 clusters versus time are shown in Fig. 2(b). For small clusters, there exist discrete mass spectra, while the yields of Au2 and Au3 are 9.13 nA and 6.64 nA, respectively. The inset shows the optimized deposition beam current of the Au923±20 clusters versus time. When we switch off and switch on the mass selection pulse to stop and restart cluster deposition, the beam current shows an obvious decrease to zero, followed by rebound. In this way, we confirm that the deposition current of the Au923±20 clusters is real rather than the background noise, and the maximum steady deposition current for Au923±20 is about 80 pA. In Fig. 2(c), the STEM images of the Au923±20 clusters with low magnification clearly indicate their uniform spherical shape. The average size is 3.64 ± 0.11 nm, as shown in Fig. 2(d), after measuring over 100 clusters. The narrow FWHM with the dense distribution shows that we successfully synthesized the Au923±20 clusters with a high yield. Three sets of matches between simulated and experimental STEM images for typical structures of the Au923±20 clusters are shown in Fig. 3(a)–(c). Each simulated structure has some typical characteristics to distinguish from others. Taking Fig. 3(c) for instance, the circular rings with a bright dot in it is a typical feature of the Ih structure in STEM. In this manner, we classify the cluster in Fig. 3(c) as an Ih structure, while the three turns of stripes and the straight stripe distinguish them from each other (Fig. 3(a) and (b)). In Fig. 3(d), the fuzzy STEM image is attributed to either the naturally amorphous structure or the specific angle facing the electron beam cannot match each of the simulated images; therefore, we classify this as an unknown structure. By comparing simulated and experimental images, clusters with different structures observed in STEM are classified and counted. The proportions of the above four structures considerably change in the four groups, as shown in Fig. 4(a)–(d). The dark gray, red, blue and green parts in the pie charts represent Dh, Fcc, Ih and an unknown structure, respectively. In Fig. 4(a), namely, group 1, a statistical result of the normal synthesis condition is observed where the Dh structure occupies a majority of all clusters with a proportion of 65.45%. The Ih structure only accounts for a small part of 10.92%. Moreover, the bulk-favorable cuboctahedron (i.e. Fcc) structure only takes a small part of 18.18%. However, in group 2, with a condensation length of 12 cm, the lowest helium gas flow, a sputtering power of 60 W, and an obvious increase of Ih structure from 10.92% to 31.65% were observed. Simultaneously, the Dh structure shows a rapid decrease to 44.3%, which may indicate a structure transformation from Dh to Ih. In this condition, the Fcc structure had a slight decrease to 13.92%. When we continue to increase the sputtering power to 80 W while keeping other parameters almost unchanged, the structure proportion continues to change. As shown in Fig. 4(c), the Ih structure reaches 55.64%, while the Dh structure declines to only 25%. Furthermore, the Fcc structure shows a very slight decrease to 12.1%. When further increasing the sputtering power to 120 W, the transformation to the Ih structure slows down and only reaches 58.76%. In Fig. 4(e) the visualizing trend about the two structures Dh and Ih versus different sputtering power is shown. The dark gray and blue balls refer to the proportion of the Dh and Ih structures. The orange one refers to the sum of the proportions of the two structures, while the orange dashed line represents the average proportion of it. It is obvious that the proportions of Dh and Ih structures show considerable changes in a variety of synthesis conditions when the Ih structure rises from 10.92% to 58.76% and the Dh structure falls from 65.45% to 19.59%. Furthermore, we note that the sum of these two structures occupy a quite stable percentage of about 77.83% for all groups. Therefore, it might be possible that the transformation from Dh to Ih is the major process in our structural control.
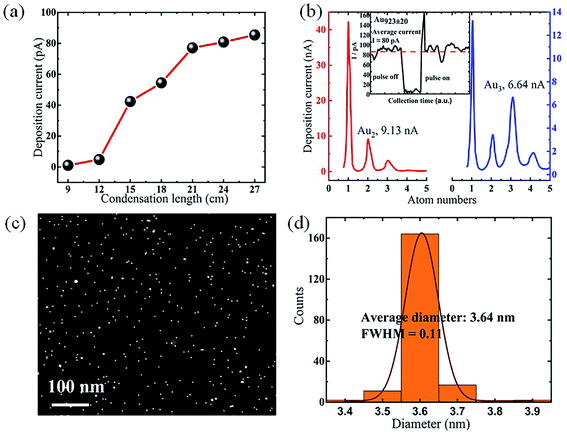 |
| Fig. 2 Influences of the gold cluster yield and the size statistics. (a) The influence of the condensation length on the Au923±20 cluster yield. The deposition current increases monotonously as the condensation length increases. (b) Yields of gold clusters. The controllable maximum beam current of Au2 and Au3 clusters are 9.13 and 6.64 nA, respectively. The inset shows the maximum steady deposition current of Au923±20 clusters versus time. When we switch off the pulse to stop collection, an obvious decrease in the deposition current from 80 pA to about 0 appears. (c) The uniform distribution of the as-prepared Au923±20 clusters in the STEM image of in a low magnification. (d) Size statistics of Au923±20 clusters. The average size is 3.67 nm and the FWHM is 0.11 nm. | |
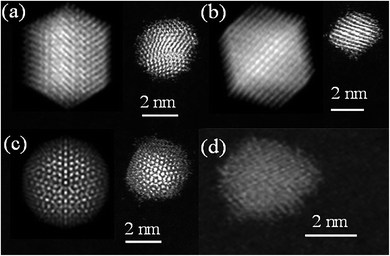 |
| Fig. 3 Comparison between the simulated STEM images and the experimental ones for different structures. (a) On the top left corner is the simulated STEM image of a Dh structure, while the top right one is an experimental result. (b) The simulated STEM image and an experimental result of an Fcc structure. (c) The simulated STEM image and an experimental result of an Ih structure. (d) The experimental STEM images that cannot match with each simulated STEM ones. | |
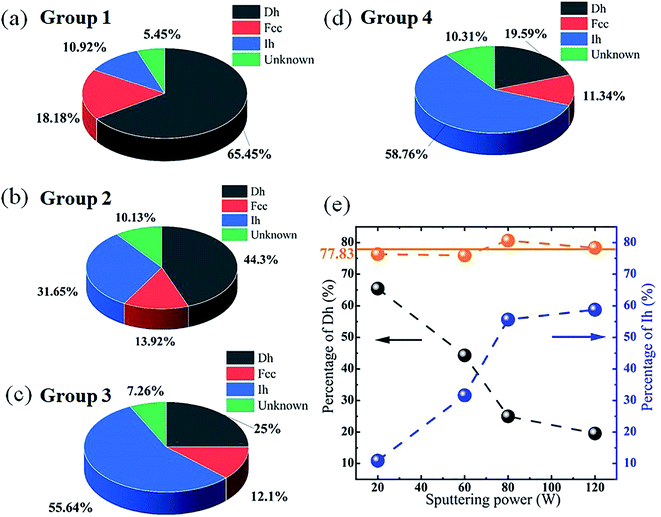 |
| Fig. 4 The structural statistic of the Au923±20 clusters in different synthesis conditions. (a) The normal synthesis condition for the Au923±20 clusters with 20 W sputtering power, 27 cm condensation length, and high helium gas flow. (b)–(d) The changed synthesis condition with the lowest helium gas flow and the shortest condensation length of 12 cm. The sputtering power increased from 20 to 60, 80 and 120 W. (e) The dark gray and blue balls refer to the proportion of Dh and Ih structures, while the orange one refers to the sum of proportions of the two structure. The orange-dashed line represents the average proportion of the sum. | |
Discussion
The yield of clusters with certain atom numbers reaches maximum when we have a suitable condensation condition to control the collision time during the cluster growth. However, the cluster yield is partially limited because only part of atoms aggregates into the (923 ± 20) range. The beam consumption in the chamber occupies a large part in the total loss; therefore, the aerodynamic optimization of chamber is required to effectively guide the beam out of the chamber. As for the structure control, the Au923±20 clusters prepared in the gas phase are mostly in penta-rotating symmetrical structures (Dh or Ih structures) and the proportion of the sum of these two structures takes up over three quarters. Nevertheless, the proportion of bulk-favorable cuboctahedron structure takes up only 10–20%. It needs further study that at which atom numbers the bulk-favorable structure begin to be the thermodynamically most stable structure. This atom numbers could be a limit for bulk-like Au nanoparticles.10 The effective structure control indicates that the proportions of different cluster structures are controllable by changing the synthesis condition in the condensation chamber. As shown by previously reported studies,5,26 the vibrational frequencies in Ih structures are smaller than Dh ones, while Dh structures have softer vibrations than Fcc ones. Considering the entropic contributions in cluster structure transitions, we can simply make a point that for a given atomic numbers of clusters, Ih structures prefer higher temperature than Dh and Fcc ones. In our experiments, by decreasing the condensation length as well as the flow of helium gas, the collision times of clusters with helium gas decreased, thus the heat carried away from clusters reduced, creating high temperature during cluster growth.27,28 The increased sputtering power leads to the excessive sputtered energetic gold atoms, which further results in insufficient cooling within the gold atom gas. In this manner, we increase the average temperature of clusters, and the preferred structural transition from Dh to Ih happens. The bulk-favorite Fcc structures are influenced in the same way by the gradually significant entropic contributions. Note that the increased temperature suppresses the previously small proportion of it. These changes lead to an evolution in Dh fractions.29,30 However, there remain some problems. First, the cluster yield shows a great decrease to several pA when we change the synthesis conditions because the increased temperature, the insufficient helium gas and the shortest condensation length influence the average collision times in the aggregation of atoms,27,31 creating a harsh environment for the cluster growth, particularly for such a large cluster. Second, the proportion of penta rotation structures is almost invariable, which may indicate that there exists an upper limit in the Ih structure if the most probable structure transforming happens from the Dh to the Ih structure. Finally, the separation32 and preservation of the metastable clusters33,34 are needed for further applications. In our study, the structure change during the STEM can easily happen during the irradiation of electron beam.
Conclusion
In this study, atomically precise Au923±20 clusters are generated in a gas-phase condensation cluster source equipped with a lateral time-of-flight mass selector. The beam current reaches up to 9.13 nA for small clusters and 80 pA for the Au923±20 clusters. For Au923±20, the average size is confirmed by STEM to be 3.64 ± 0.11 nm with a narrow distribution FWHM = 0.11 nm. By adjusting the synthesizing conditions in the condensation chamber, we successfully enrich the Ih clusters of size 923 ± 20 gold atoms to be the absolute majority from 10.92% to 58.76%, while the original majority Dh structure falls from 65.45% to 19.59%.
Conflicts of interest
The authors declare no conflict of interest.
Acknowledgements
We gratefully acknowledge the financial support of the National Key R&D Program of China (2017YFA0303203), the National Natural Science Foundation of China (91622115, 11522432, 11574217, U1732273, U1732159, 61822403, 11874203, 11904165 and 11904166), the Natural Science Foundation of Jiangsu Province (BK20160659), and the Fundamental Research Funds for the Central Universities.
References
- T. P. Martin, Shells of atoms, Phys. Rep., 1996, 273, 199–241 CrossRef CAS.
-
R. L. Johnston, Atomic and molecular clusters, CRC Press, 2002 Search PubMed.
- W. A. De Heer, The physics of simple metal clusters: experimental aspects and simple models, Rev. Mod. Phys., 1993, 65, 611 CrossRef CAS.
- J. Wang, G. Wang and J. Zhao, Density-functional study of Au n (n = 2–2 0) clusters: lowest-energy structures and electronic properties, Phys. Rev. B: Condens. Matter Mater. Phys., 2002, 66, 035418 CrossRef.
- F. Baletto and R. Ferrando, Structural properties of nanoclusters: energetic, thermodynamic, and kinetic effects, Rev. Mod. Phys., 2005, 77, 371 CrossRef CAS.
- A. Y. Tsivadze, G. Ionova, V. Mikhalko, I. Ionova and G. Gerasimova, The fine structure of plasmon bands in gold nanoparticles, Prot. Met. Phys. Chem. Surf., 2013, 49, 166–168 CrossRef CAS.
- H. Häkkinen, M. Moseler and U. Landman, Bonding in Cu, Ag, and Au clusters: relativistic effects, trends, and surprises, Phys. Rev. Lett., 2002, 89, 033401 CrossRef PubMed.
- S. Bulusu, X. Li, L.-S. Wang and X. C. Zeng, Evidence of hollow golden cages, Proc. Natl. Acad. Sci. U. S. A., 2006, 103, 8326–8330 CrossRef CAS PubMed.
- J. Li, X. Li, H.-J. Zhai and L.-S. Wang, Au20: a tetrahedral cluster, Science, 2003, 299, 864–867 CrossRef CAS PubMed.
- C. L. Cleveland,
et al., Structural evolution of smaller gold nanocrystals: the truncated decahedral motif, Phys. Rev. Lett., 1997, 79, 1873 CrossRef CAS.
- N. Shao,
et al., Probing the Structural Evolution of Medium-Sized Gold Clusters: Aun−(n = 27–35), J. Am. Chem. Soc., 2010, 132, 6596–6605 CrossRef CAS PubMed.
- Y. Wang, S. Teitel and C. Dellago, Melting of icosahedral gold nanoclusters from molecular dynamics simulations, J. Chem. Phys., 2005, 122, 214722 CrossRef PubMed.
- D. Foster, T. Pavloudis, J. Kioseoglou and R. Palmer, Atomic-resolution imaging of surface and core melting in individual size-selected Au nanoclusters on carbon, Nat. Commun., 2019, 10, 1–8 CrossRef CAS PubMed.
- D. Ricci, A. Bongiorno, G. Pacchioni and U. Landman, Bonding trends and dimensionality crossover of gold nanoclusters on metal-supported MgO thin films, Phys. Rev. Lett., 2006, 97, 036106 CrossRef PubMed.
- Q. Li, D. Yin, J. Li and F. L. Deepak, Atomic-scale understanding of gold cluster growth on different substrates and adsorption-induced structural change, J. Phys. Chem. C, 2018, 122, 1753–1760 CrossRef CAS.
- K.-J. Hu,
et al., Atomic resolution observation of a size-dependent change in the ripening modes of mass-selected Au nanoclusters involved in CO oxidation, J. Am. Chem. Soc., 2015, 137, 15161–15168 CrossRef CAS PubMed.
- S. R. Plant, L. Cao and R. E. Palmer, Atomic structure control of size-selected gold nanoclusters during formation, J. Am. Chem. Soc., 2014, 136, 7559–7562, DOI:10.1021/ja502769v.
- G. Sun and P. Sautet, Metastable structures in cluster catalysis from first-principles: Structural ensemble in reaction conditions and metastability triggered reactivity, J. Am. Chem. Soc., 2018, 140, 2812–2820 CrossRef CAS PubMed.
- C. Yu,
et al., Optical properties of size selected neutral Ag clusters: electronic shell structures and the surface plasmon resonance, Nanoscale, 2018, 10, 20821–20827 RSC.
- Z. Wang and R. Palmer, Mass spectrometry and dynamics of gold adatoms observed on the surface of size-selected Au nanoclusters, Nano Lett., 2012, 12, 91–95 CrossRef CAS PubMed.
- Z. Wang and R. Palmer, Determination of the ground-state atomic structures of size-selected Au nanoclusters by electron-beam-induced transformation, Phys. Rev. Lett., 2012, 108, 245502 CrossRef CAS PubMed.
- B. Von Issendorff and R. Palmer, A new high transmission infinite range mass selector for cluster and nanoparticle beams, Rev. Sci. Instrum., 1999, 70, 4497–4501 CrossRef CAS.
- H. Haberland, H. Kornmeier, C. Ludewigt, A. Risch and M. Schmidt, A double/triple
time-of-flight mass spectrometer for the study of photoprocesses in clusters, or how to produce cluster ions with different temperatures, Rev. Sci. Instrum., 1991, 62, 2621–2625 CrossRef CAS.
-
M. Fárník, Molecular dynamics in free clusters and nanoparticles studied in molecular beams, ICT Prague Press, Institute of Chemical Technology Prague, 2011 Search PubMed.
- M. Schwartzkopf,
et al., From atoms to layers: in situ gold cluster growth kinetics during sputter deposition, Nanoscale, 2013, 5, 5053–5062 RSC.
- J. P. K. Doye and F. Calvo, Entropic effects on the structure of Lennard-Jones clusters, J. Chem. Phys., 2002, 116, 8307–8317 CrossRef CAS.
- E. Kesälä, A. Kuronen and K. Nordlund, Molecular dynamics simulation of pressure dependence of cluster growth in inert gas condensation, Phys. Rev. B: Condens. Matter Mater. Phys., 2007, 75, 174121 CrossRef.
- E. Blaisten-Barojas and M. Zachariah, Molecular-dynamics study of cluster growth by cluster–cluster collisions, Phys. Rev. B: Condens. Matter Mater. Phys., 1992, 45, 4403 CrossRef PubMed.
- P. J. Kelly and R. D. Arnell, Magnetron sputtering: a review of recent developments and applications, Vacuum, 2000, 56, 159–172 CrossRef CAS.
- M. Seah, Universal equation for argon gas cluster sputtering yields, J. Phys. Chem. C, 2013, 117, 12622–12632 CrossRef CAS.
- S. Pratontep, S. Carroll, C. Xirouchaki, M. Streun and R. Palmer, Size-selected cluster beam source based on radio frequency magnetron plasma sputtering and gas condensation, Rev. Sci. Instrum., 2005, 76, 045103 CrossRef.
- T. Suetsuna,
et al., Separation of N2@C60 and N@C60, Chem.–Eur. J., 2002, 8, 5079–5083 CrossRef CAS.
- A. Simo,
et al., Formation mechanism of silver nanoparticles stabilized in glassy matrices, J. Am. Chem. Soc., 2012, 134, 18824–18833 CrossRef CAS PubMed.
-
J. F. Hund, Surface deposition and encapsulation of metallic clusters, University of Missouri-Rolla, 2004 Search PubMed.
Footnote |
† These authors contributed equally to this work. |
|
This journal is © The Royal Society of Chemistry 2020 |