DOI:
10.1039/D0NA00106F
(Paper)
Nanoscale Adv., 2020,
2, 1949-1955
On the formation of superoxide radicals on colloidal ATiO3 (A = Sr and Ba) nanocrystal surfaces†
Received
7th February 2020
, Accepted 15th April 2020
First published on 16th April 2020
Abstract
Controlling the surface chemistry of colloidal semiconductor nanocrystals is critical to exploiting their rich electronic structures for various technologies. We recently demonstrated that the hydrothermal synthesis of colloidal nanocrystals of SrTiO3, a technologically-relevant electronic material, provided a strong negative correlation between the presence of an O2-related surface defect and hydrazine hydrate [W. L. Harrigan, S. E. Michaud, K. A. Lehuta, and K. R. Kittilstved, Chem. Mater., 2016, 28(2), 430]. When hydrazine hydrate is omitted during the aerobic hydrothermal synthesis, the surface defect is observed. However, it can be removed by either the addition of hydrazine hydrate or by purging the reaction solution with argon gas before the hydrothermal synthesis. We also propose that the formation of the O2-related defect is mediated by the reduction of dissolved O2 by lactate anions that are present from the titanium precursor. This work helps elucidate the nature of the O2-related defect as a superoxide anion
and presents a mechanism to explain its formation during the hydrothermal synthesis of SrTiO3 and related BaTiO3 nanocrystals.
Introduction
SrTiO3 and BaTiO3 are wide-gap semiconductors that have received much attention due to their rich defect chemistries that enable a wide range of electrical properties from insulating to metallic.1 In addition to exhibiting prototypical quantum paraelectric behavior,2 these semiconductors are also promising host lattices for visible-light photocatalytic H2O splitting and CO2 reduction,3–9 sensors,10 and memristors.11,12 Recent advances in the colloidal synthesis13–20 of ternary metal oxide nanocrystals (NCs) including SrTiO3 and BaTiO3 has generated further interest in the emergent phenomena that arise in these technologically-relevant materials when prepared with nanometer dimensions.
Most of the interesting applications of bulk SrTiO3 results from the presence of two native n-type defects: oxygen vacancies (VO) and self-trapped Ti3+ ions. These defects increase the carrier density and mid-gap trapped states which give rise to tunable blue light emission21 but also greatly influence light absorption in the visible region that has been shown to enhance photocatalytic performance in bulk powders.22 For example, superoxide radicals
present on the surface of Ti(IV)-based metal oxides play an essential role in photocatalysis and degradation of organic pollutants.23–25 These
are generally produced by post-synthetic treatments of reduced titanium oxide-based materials with molecular oxygen (O2) or H2O2. Yu et al. generated surface
by injecting electrons from photoinduced excited states into adsorbed O2 on the surface of colloidal TiO2 nanoparticles.26 Another potential use of
is the catalytical decomposition of H2O2 on the surface of titanium oxides27 and oxidative coupling of alkyl radicals.28 In most of these cases, the metal oxide surface needs to be activated prior to
formation by irradiation or suitable chemical treatments.
We recently reported the synthesis29 of colloidal SrTiO3 NCs by modified hydrothermal methods15,17,18 that are readily suspended in non-polar solvents. Electron paramagnetic resonance (EPR) and optical spectroscopies showed that the SrTiO3 NCs can display spectroscopic signatures that were assigned to an oxygen-related surface defect that is sensitive to the presence of hydrazine hydrate during synthesis. However, the role of hydrazine in the formation of this surface defect remained speculative. Hydrazine has been previously used as an additive during the hydrothermal synthesis of SrTiO3 and BaTiO3 NCs. Sun and co-workers have studied the absorption of hydrazine on the surface of BaTiO3 nanoparticles.30 Experimental and simulation analysis indicated that only amorphous BaTiO3 particles are obtained without the addition of hydrazine. Similarly, Fujinami et al. claimed that SrTiO3 NCs without cubic shape were obtained when synthesis was carried out in the absence of hydrazine.17 However, our recent report presented evidence that near-cubic colloidal SrTiO3 NCs can be prepared with or without the addition of hydrazine.29
Herein we report a systemic investigation of this correlation between hydrazine and the presence of this surface defect in colloidal SrTiO3 NCs. We hypothesize that the hydrazine may scavenge molecular O2 inside the closed reaction vessel during synthesis, thus providing an inert atmosphere that would inhibit
formation on the NC surface. To test the role of hydrazine, we performed the hydrothermal synthesis both with and without hydrazine prepared in ambient conditions, and also without hydrazine but under anaerobic conditions after purging the reaction solution with argon gas. Reactions performed with hydrazine or under anaerobic conditions produced the same observations and strongly suggest that hydrazine is indeed removing O2 inside the closed reaction vessel. These experiments also strengthen our assignment of the surface-related defect species as
ions for the SrTiO3 NCs samples prepared aerobically without hydrazine. We propose that lactate ligands of the Ti(IV) precursor reduce O2 to
ions during the hydrothermal synthesis (200 °C and basic conditions). To support this mechanism and generality of
formation and the importance of lactate ions, we prepared BaTiO3 NCs from the same lactate-containing Ti(IV) precursors as well as a lactate-free precursor.
defects are observed only in NCs prepared from precursor that contain lactate ligands under aerobic conditions. These results provide convincing evidence that the choice of precursor can lead to unique surface chemistry and the formation of
defects on titanate-based NCs.
Experimental
Materials
Strontium hydroxide octahydrate (Sr(OH)2·8H2O, 99%, Alfa Aesar), barium hydroxide octahydrate (Ba(OH)2·8H2O, 99.95%, Acros Organics), titanium(IV) bis(ammonium lactate) dihydroxide (TALH, 50% in water, Alfa Aesar), titanium(IV) tert-butoxide (Ti(OBu)4, 99%, Acros Organics), 1-butanol (99%, Acros Organics), tetramethylammonium hydroxide (NMe4OH, Acros Organics), sodium hydroxide (NaOH, Certified ACS, Fisher Chemical), hydrazine hydrate (99%, Acros Organics), oleic acid (90%, Fisher Chemical), oleylamine (50%, TCI America), ethanol (200 proof, PHARMCO-AAPER) and hexanes (optima grade, Fisher Chemicals) were all used as received.
Synthesis of colloidal SrTiO3 NCs from TALH
Synthesis of colloidal SrTiO3 NCs was carried out by the hydrothermal method as reported previously by our group.29 In a typical procedure, 1.25 mmol of each of TALH and Sr(OH)2 were dissolved in 30 mL of distilled water in 45 mL Teflon-lined autoclave. The pH of the solution was then adjusted to 12.1 with a 10 M NMe4OH solution followed by the addition of oleic acid (2.5 mmol). The reaction vessel was then sealed and heated to 200 °C in oven for 24 hours. The resulting NCs were collected, washed with ethanol three times and suspended in hexanes. This procedure described above is referred herein as method-I.
Method-I was modified to prepare two different sets of colloidal NCs. In the first modification referred to as method-II, hydrazine hydrate (5 mmol) was added in reaction mixture before adding oleic acid. The pH of the solution remained at 12.1 after addition of hydrazine hydrate. The last modification, method-III, was identical to method-I but the reaction solution was purged with argon gas for 60 minutes prior to closing the Teflon-lined reaction vessel.
Synthesis of colloidal BaTiO3 NCs from TALH
Synthesis of colloidal BaTiO3 NCs was also carried out by a similar hydrothermal method and TALH precursor but included constant magnetic stirring. In a typical preparation, 1.5 mmol of each TALH and Ba(OH)2 were dissolved in 24 mL distilled water followed by addition of 6 mL of NaOH (5 M). The reaction solution was then transferred to a 45 mL Teflon-lined autoclave and oleylamine (6 mmol) and oleic acid (6 mmol) were added. The pH of the reaction solution was 12. The sealed autoclave was placed in custom-made aluminum block housing that was heated to 215 °C and stirred constantly for 24 h using a stirring hotplate. After the synthesis, autoclave was cooled to room temperature and the solid product was collected, washed with EtOH, and dissolved in nonpolar solvents such hexanes to produce a transparent solution. This procedure described above is referred herein as method-A. The method-A was modified to prepare another set of BaTiO3 NCs referred as method-B. In this modification, hydrazine hydrate (5 mmol) was added before adding the oleylamine and oleic acid. Method-A and -B for BaTiO3 mimics method-I and -II for the SrTiO3 NCs.
Synthesis of BaTiO3 NCs from Ti(OBu)4
Synthesis of lactate-free BaTiO3 NCs was done using an aerobic hydrothermal method adopted from literature.31 In a typical experiment, aqueous solutions containing 1 mmol of Ba(OH)2 dissolved in 5 mL water and 12.5 mmol of NaOH dissolved in 5 mL water were mixed with 10 mL of 1-butanol containing 1 mmol Ti(OBu)4 and 2.5 mL of oleic acid. The pH of the solution was 12.2. The resulting precursor solution was transferred to a 45 mL autoclave, closed under ambient conditions, and heated to 180 °C for 18 hours. The resulting product was collected, washed with EtOH several times and dispersed in non-polar solvents such as hexanes.
Physical characterization
All measurements were collected at room-temperature unless specified otherwise. Electronic absorption spectra were collected on colloidal suspensions in air-tight quartz cuvettes with 1 cm pathlengths (Cary 50 Bio). EPR spectra were measured at X-band frequency (9.6 GHz) with a Bruker Elexsys-500 equipped with a Super High QE (ER4123SHQE) cavity. Transmission electron microscopy (TEM) images of NCs deposited onto copper grids (CF400-CU-50, Electron Microscopy Sciences) with a 3 nm carbon coating (JEOL TEM-2000FX). Powder X-ray diffraction patterns were collected in the Bragg–Brentano configuration with a Cu Kα source (Rigaku SmartLab SE). FTIR spectra were collected using Bruker Alpha-P equipped with a diamond attenuated total reflectance (ATR) crystal. High resolution electrospray ionization mass spectra (ESI-MS) were collected in negative ion mode with Bruker MicroTOF-II. In a typical sample preparation for ESI-MS, the aqueous reaction mixture from the hydrothermal synthesis was centrifuged to separate NCs from the rest of the water-soluble side-products. The aqueous supernatant was used for ESI-MS measurements with no further purification.
Results and discussion
The crystallinity and phase purity of the SrTiO3 NCs was confirmed by powder X-ray diffraction (see ESI†). All samples matched to cubic SrTiO3 in the Pm
m space group.32 The similar powder patterns among the samples suggests that modification of hydrothermal synthesis either by adding hydrazine hydrate or degassing the precursor solution has no effect on the crystalline phase or phase purity. TEM images also demonstrate cuboidal morphology of the SrTiO3 NCs prepared by method-III in agreement with previously reported NC shapes made by method-I or method-II (see ESI†).29
FTIR absorption measurements collected on SrTiO3 NCs prepared from each method are shown in Fig. 1. The prominent peaks at 1540 cm−1 and 1450 cm−1 correspond to the symmetric and asymmetric stretches of the bound carboxylate head group of the surface oleate ligand and is observed in each sample. Aliphatic bands from the oleate surface ligands also appear ca. 2900 cm−1. All samples thus indicate the NC surfaces are passivated by oleate ligands within the sensitivity of the instrument. These spectra are similar to prior reports of SrTiO3 made in the similar method17 but at pH 13.5. There is no evidence of N–H stretches from hydrazine in the NCs prepared by method-II that would appear around 3400 cm−1.33 This observation suggests that hydrazine is not being adsorbed on the surface of SrTiO3 NCs. Furthermore, hydrazine adsorption is not expected as it is known to decompose rapidly at this reaction temperature (200 °C) in the presence of oxygen to possible products of N2 and H2O.34 Fujinami et al. also observed essentially no change in pH of the reaction solution after SrTiO3 NC synthesis regardless of whether hydrazine was present, which was speculated to play a different role than decomposing to NH3.17
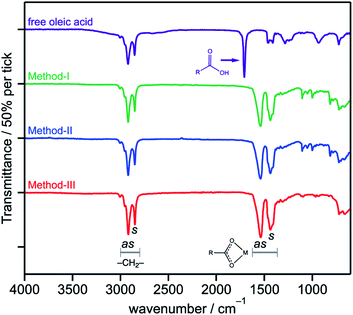 |
| Fig. 1 FTIR spectra of solvent-evaporated SrTiO3 NCs prepared by method-I (green), method-II (blue) and method-III (red). The top spectrum belongs to oleic acid (purple). The s and as next to the peaks refer to the symmetric and asymmetric stretches associated with the aliphatic C–H or carboxylate vibrations. | |
The electronic absorption spectrum of all colloidal SrTiO3 NCs dispersed in hexanes is dominated by the band edge absorption at ca. 3.25 eV consistent with bulk SrTiO3 (see Fig. 2).35 However, NCs prepared by the aerobic hydrothermal method-I also exhibit broad absorption throughout the entire visible region and appear reddish-brown (see color photographs shown in Fig. 2 inset). The broad sub-bandgap absorption is absent in SrTiO3 samples prepared using either method-II or method-III.
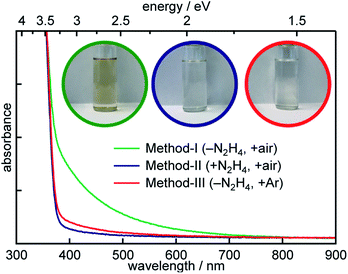 |
| Fig. 2 Electronic absorption spectra of colloidal SrTiO3 NCs dispersed in hexanes prepared by method-I (green), method-II (blue) and method-III (red). Optical densities were matched at ∼350 nm. Color photographs of NCs dispersed in hexanes are shown in insets. | |
The origin of the visible absorption in method-I NCs suggests the presence of defects with sub-bandgap levels. Native point defects such as VO or Ti3+ are also known to give rise to visible light absorption in bulk SrTiO3. Electronic transitions from the valence band to different VO charge states have been observed between 450 and 550 nm.36–38 Rice et al. recently attributed a transition involving the VO to an absorption band at ca. 405 nm in reduced SrTiO3 crystals.39 Similar VO defects have also been described as polarons. However, the electronic transition of the localized electron trapped on Ti3+ sites to the conduction band minimum is broad and centered in the near-IR region.40,41 To explore the origin of the brownish coloration of the SrTiO3 NCs prepared by method-I, we carried out EPR spectroscopy measurements on all three types of SrTiO3 NCs.
The room temperature, X-band EPR spectrum of the SrTiO3 NCs prepared by method-I is shown in Fig. 3. These NCs exhibit a narrow resonance near 351 mT corresponding to g-value of 2.003. Upon addition of hydrazine (method-II) or purging the precursors with argon (method-III), this EPR signal disappears. Carter et al. have identified four types of oxygen-related defects on the surface of TiO2 (anatase-type) using EPR spectroscopy and the signal at g = 2.003 was assigned to surface-adsorbed paramagnetic superoxide radicals (
S = 1/2).42 Those authors also propose the formation involves electron transfer from trapped electrons at Ti3+ sites to surface-O2 to produce
We also tentatively assigned the g = 2.003 signal to
in our initial report on the synthesis and characterization of SrTiO3 NCs.29 We also demonstrated in a more recent report that this g = 2.003 signal can be increased upon exposing photochemically reduced (Ti3+-rich) Cr3+-doped SrTiO3 NCs to air.41 Others have also reported a TiO2-based NCs treated with H2O2 that display similar absorption in the visible region originating from surface-adsorbed
defects.43,44 Based on the similarity to previous reports and observations,41,43 we attribute the reddish-brown coloration and EPR resonance at g = 2.003 observed in Fig. 2 and 3, respectively, to the presence of
radicals on the surface of SrTiO3 NCs prepared under aerobic hydrothermal conditions (method-I). We further assign the visible absorption to defect-induced sub-bandgap states that give rise to charge transfer-like transitions.
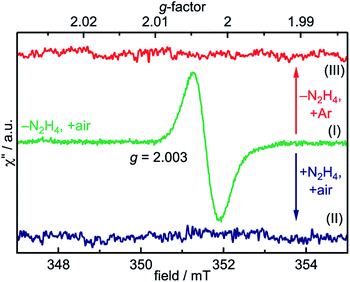 |
| Fig. 3 Room temperature EPR spectra of NCs prepared by method-I (middle/green), method-II (bottom/blue), and method-III (top/red). | |
Our working hypothesis is that the
ions are formed via reduction of dissolved O2 during the hydrothermal synthesis. The lack of
formation when prepared by either method-II or method-III is thus presumably due to a very low O2 content in the reaction solution during the hydrothermal synthesis. Despite these results, we do not have a direct mechanistic understanding of how the
is formed during synthesis. One outstanding question that we sought to answer is the identity of the electron donor. The
formation at TiO2 surfaces is believed to proceed via electron transfer from Ti3+ sites to adsorbed O2.45,46 In bulk SrTiO3, oxygen vacancies are thought to exist between adjacent localized Ti3+ sites,47,48 which could serve as an electron donor. However, no spectroscopic evidence for the existence of VO and any localized Ti3+ defects were found in SrTiO3 colloidal NCs. It is possible that these species are only formed under hydrothermal conditions during synthesis but suggests the presence of an alternative electron donors such as lactate ions.
The hydrothermal synthesis of SrTiO3 NCs involves the hydrolysis of titanium(IV) bis(ammonium lactato) dihydroxide (TALH) as the titanium source under basic conditions. Möckel et al. have proposed that the hydrolysis of TALH to form TiO2 NCs produces free ammonium lactate in neutral or slightly acidic conditions.49 Seisenbaeva et al. have proposed hydrolysis of TALH forms a stable, tetrameric (NH4)8Ti4O4(lactate)8 cluster that serves as a precursor to the formation of TiO2 NCs under basic conditions.50 In both of above mentioned references, lactate ions are commonly observed as hydrolysis by-product. Lactate ions have been shown to serve as electron donors in various chemical and biological systems to produce O2-based radicals.51,52 Therefore, we hypothesize that the lactate ions reduce dissolved O2 under extremely high pH to produce
and the corresponding by-product pyruvate anions as described by Scheme 1.
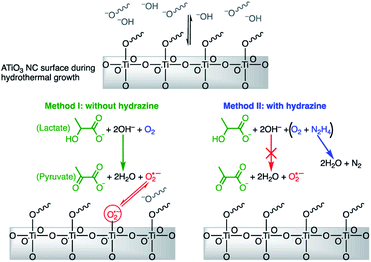 |
| Scheme 1 Proposed mechanism of superoxide formation and adsorption onto the dynamic ATiO3 surface during the hydrothermal synthesis. | |
In Scheme 1, we depict ATiO3 surface that is stabilized by oleate ligands under hydrothermal conditions (pH 12 and 200 °C). At this high temperature, we expect the surface ligands to rapidly exchange between bound and free. This dynamics surface would allow for
to bind to the surface in method-I and become stabilized upon cooling to room temperature. In the anaerobic cases, method-II and method-III, there is no direct way to produce
in solution and therefore rule out the possibility of
adsorption on NC surface.
To confirm the presence of lactate and pyruvate anions, we performed mass spectrometry analysis of reaction supernatants from the various syntheses without purification. Fig. 4 shows a zoomed in region of the negative ion mode ESI-MS spectra collected from all three methods. The dominant peak at m/z 89 is attributed to lactate ions which are formed in all three methods consistent with our hypothesis. Despite being weak, the presence of a minority peak at m/z 87 is detected in each of the samples and corresponds to pyruvate ions. When normalized to the lactate signals for the three methods, we detect about a three-fold higher relative intensity of pyruvate anion signal from the supernatant from method-I. While not absolutely quantitative, the ESI-MS results suggest this conversion yield is <1%. Despite this limitation, the observation is consistent with the proposed mechanism that at least some fraction of the lactate ions undergoes the conversion shown in Scheme 1. The lower O2 content in method-III precludes the
formation which in turn can be supported by observing relatively a lower pyruvate concentration. However, the absence of any
defects together with much lower pyruvate content in method-II questions the role of hydrazine in controlling the
formation during hydrothermal synthesis.
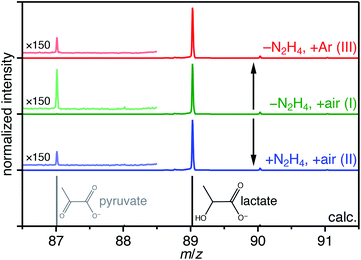 |
| Fig. 4 Negative-ion mode ESI-MS of supernatants from the various reactions in the region of the lactate ([C3H5O3]˙−) and pyruvate ([C3H3O3]˙−) anions. The spectra are normalized to the major peak from the lactate anion at m/z 89. | |
Based on results above, hydrazine appears to control the formation of
defects by limiting the available O2 in the synthesis. Hydrazine has been used to remove O2 in industrial-scale reactors for decades.53,54 The reaction between hydrazine and O2 produces N2 and 2H2O, which provides the inert atmosphere during synthesis as shown in Scheme 1.34 Post-synthetic attempts to control the concentration of defects on the surface of the SrTiO3 NCs prepared by method-I were also performed. When an excess of hydrazine hydrate is added to an aqueous mixture of defect-rich NCs and heated under aerobic hydrothermal conditions, no change in the appearance of the NCs was observed suggesting no change in surface defects (data not shown).
To test the generality of this approach, we extended the study to BaTiO3 NCs using a similar hydrothermal-based synthesis with TALH and a literature solvothermal method (see ESI†). The room temperature EPR spectra of BaTiO3 NCs prepared from all methods is shown in Fig. 5. Similar to the results for SrTiO3, the appearance of a
resonance at g = 2.003 is observed in BaTiO3 samples prepared using TALH and air. The formation of these
defects can be inhibited by adding hydrazine to remove O2 from the reaction that is consistent with our proposed mechanism.
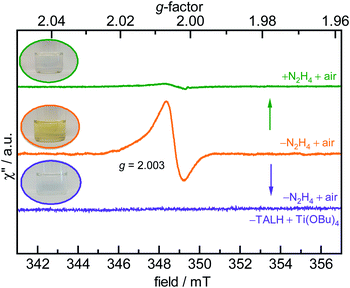 |
| Fig. 5 Room temperature EPR spectra of BaTiO3 NCs prepared without hydrazine (orange/middle), with hydrazine (top/green) and from lactate-free precursor (bottom/purple). All samples were prepared in air. The color photographs of colloidal solution in hexanes for NCs prepared from all three methods are shown in insets. | |
To confirm the critical role of lactate ions in the formation of these surface
defects, we synthesized BaTiO3 NCs from a lactate-free titanium precursor, Ti(OBu)4, using similar hydrothermal conditions as used above with TALH.31 The resulting lactate-free BaTiO3 NCs are transparent below the band gap and do not display any detectable EPR signal as shown in Fig. 5 and in the ESI.† This result is entirely consistent with the critical role that lactate ions play in the formation of
defects during hydrothermal synthesis of ATiO3 and likely also TiO2 NCs.
Conclusions
In conclusion, we have presented a systemic study of the surface defects formed during the hydrothermal synthesis of colloidal SrTiO3 and BaTiO3 NCs. We found that an electron transfer step presumably from lactate anions (from the Ti4+ precursor) to dissolved O2 may be the key step that leads to the formation of the surface-adsorbed
ions under these reaction conditions (high pH and high temperature). These surface
defects are EPR active and impart a broad feature throughout the visible region that produces a reddish-brown color to the NCs. We also provided evidence that the removal of dissolved O2 by either (1) addition of hydrazine, (2) purging with argon, or (3) using lactate-free Ti(IV) precursors inhibit
formation. These results shed light on the role of additives in the chemical synthesis of colloidal ATiO3 NCs and we are currently investigating the generality of this approach to control the formation of O2-related surface defects in other colloidal metal oxide NCs. Modification of these surface defects through post-synthetic treatments are currently underway.
Conflicts of interest
The authors declare that this research was conducted in the absence of any commercial or financial relationships that could be constructed as a potential conflict of interest.
Acknowledgements
This work was supported by the U.S. National Science Foundation (DMR-1747593). The acquisition of the powder X-ray diffractometer used in this work was supported by the U.S. National Science Foundation Major Research Instrumentation program (CHE-1726578). R. J. N. acknowledges support through the NSF REU Program (CHE-1659266). We acknowledge Prof. Dhandapani Venkataraman at the University of Massachusetts Amherst for use of the FTIR spectrophotometer. We also thank Blaise Arden and Jillian Denhardt for ESI-MS measurements, and Dr William Harrigan for fruitful discussions in the early stages of this project.
Notes and references
- J. Son, P. Moetakef, B. Jalan, O. Bierwagen, N. J. Wright, R. Engel-Herbert and S. Stemmer, Epitaxial SrTiO3 films with electron mobilities exceeding 30
000 cm2 V−1 s−1, Nat. Mater., 2010, 9, 482 CrossRef CAS PubMed.
- K. Müller and H. Burkard, SrTiO3: An intrinsic quantum paraelectric below 4 K, Phys. Rev. B, 1979, 19(7), 3593 CrossRef.
- Q. Wang, T. Hisatomi, Q. Jia, H. Tokudome, M. Zhong, C. Wang, Z. Pan, T. Takata, M. Nakabayashi, N. Shibata, Y. Li, I. D. Sharp, A. Kudo, T. Yamada and K. Domen, Scalable water splitting on particulate photocatalyst sheets with a solar-to-hydrogen energy conversion efficiency exceeding 1%, Nat. Mater., 2016, 15(6), 611 CrossRef CAS PubMed.
- S. Shoji, A. Yamaguchi, E. Sakai and M. Miyauchi, Strontium titanate based artificial leaf loaded with reduction and oxidation cocatalysts for selective CO2 reduction using water as an electron donor, ACS Appl. Mater. Interfaces, 2017, 9(24), 20613 CrossRef CAS PubMed.
- T. K. Townsend, N. D. Browning and F. E. Osterloh, Nanoscale strontium titanate photocatalysts for overall water splitting, ACS Nano, 2012, 6(8), 7420 CrossRef CAS PubMed.
- H. Kato and A. Kudo, Visible-light-response and photocatalytic activities of TiO2 and SrTiO3 photocatalysts codoped with antimony and chromium, J. Phys. Chem. B, 2002, 106(19), 5029 CrossRef CAS.
- T. Ishii, H. Kato and A. Kudo, H2 evolution from an aqueous methanol solution on SrTiO3 photocatalysts codoped with chromium and tantalum ions under visible light irradiation, J. Photochem. Photobiol., A, 2004, 163(1–2), 181 CrossRef CAS.
- R. Niishiro, H. Kato and A. Kudo, Nickel and either tantalum or niobium-codoped TiO2 and SrTiO3 photocatalysts with visible-light response for H2 or O2 evolution from aqueous solutions, Phys. Chem. Chem. Phys., 2005, 7(10), 2241 RSC.
- H. Kato, Y. Sasaki, N. Shirakura and A. Kudo, Synthesis of highly active rhodium-doped SrTiO3 powders in Z-scheme systems for visible-light-driven photocatalytic overall water splitting, J. Mater. Chem. A, 2013, 1(39), 12327 RSC.
- N. Sarin, M. Mishra, G. Gupta, I. P. Parkin and V. Luthra, Elucidating iron doping induced n- to p- characteristics of strontium titanate based ethanol sensors, Curr. Appl. Phys., 2018, 18(2), 246 CrossRef.
- C. Baeumer, C. Schmitz, A. H. Ramadan, H. Du, K. Skaja, V. Feyer, P. Muller, B. Arndt, C. L. Jia, J. Mayer, R. A. De Souza, C. Michael Schneider, R. Waser and R. Dittmann, Spectromicroscopic insights for rational design of redox-based memristive devices, Nat. Commun., 2015, 6, 8610 CrossRef CAS PubMed.
- J. Wang, S. Choudhary, W. L. Harrigan, A. J. Crosby, K. R. Kittilstved and S. S. Nonnenmann, Transferable memristive nanoribbons comprising solution-processed strontium titanate nanocubes, ACS Appl. Mater. Interfaces, 2017, 9(12), 10847 CrossRef CAS PubMed.
- C. W. Beier, M. A. Cuevas and R. L. Brutchey, Low-temperature synthesis of solid-solution BaxSr1−xTiO3 nanocrystals, J. Mater. Chem., 2010, 20(24), 5074 RSC.
- Q. Ma and K. Kato, Nucleation and growth mechanism of barium titanate nanoblocks in hydrothermal process using aqueous titanium compound, Cryst. Growth Des., 2017, 17(5), 2507 CrossRef CAS.
- F. Dang, K.-I. Mimura, K. Kato, H. Imai, S. Wada, H. Haneda and M. Kuwabara, Growth of monodispersed SrTiO3 nanocubes by thermohydrolysis method, CrystEngComm, 2011, 13(11), 3878 RSC.
- G. Canu and V. Buscaglia, Hydrothermal synthesis of strontium titanate:
thermodynamic considerations, morphology control and crystallisation mechanisms, CrystEngComm, 2017, 19(28), 3867 RSC.
- K. Fujinami, K. Katagiri, J. Kamiya, T. Hamanaka and K. Koumoto, Sub-10 nm strontium titanate nanocubes highly dispersed in non-polar organic solvents, Nanoscale, 2010, 2(10), 2080 RSC.
- K. Park, J. S. Son, S. I. Woo, K. Shin, M.-W. Oh, S.-D. Park and T. Hyeon, Colloidal synthesis and thermoelectric properties of La-doped SrTiO3 nanoparticles, J. Mater. Chem. A, 2014, 2(12), 4217 RSC.
- S. O'Brien, L. Brus and C. B. Murray, Synthesis of monodisperse nanoparticles of barium titanate: toward a generalized strategy of oxide nanoparticle synthesis, J. Am. Chem. Soc., 2001, 123(48), 12085 CrossRef.
- F. A. Rabuffetti and R. L. Brutchey, Complex perovskite oxide nanocrystals: low-temperature synthesis and crystal structure, Dalton Trans., 2014, 43(39), 14499 RSC.
- D. Kan, T. Terashima, R. Kanda, A. Masuno, K. Tanaka, S. Chu, H. Kan, A. Ishizumi, Y. Kanemitsu, Y. Shimakawa and M. Takano, Blue-light emission at room temperature from Ar+-irradiated SrTiO3, Nat. Mater., 2005, 4(11), 816 CrossRef CAS.
- H. Tan, Z. Zhao, W. B. Zhu, E. N. Coker, B. Li, M. Zheng, W. Yu, H. Fan and Z. Sun, Oxygen vacancy enhanced photocatalytic activity of pervoskite SrTiO3, ACS Appl. Mater. Interfaces, 2014, 6(21), 19184 CrossRef CAS PubMed.
- D. Wang, L. Zhao, H. Ma, H. Zhang and L. H. Guo, Quantitative analysis of reactive oxygen species photogenerated on metal oxide nanoparticles and their bacteria toxicity: The role of superoxide radicals, Environ. Sci. Technol., 2017, 51(17), 10137 CrossRef CAS PubMed.
- Y. Li, W. Zhang, J. Niu and Y. Chen, Mechanism of photogenerated reactive oxygen species and correlation with the antibacterial properties of engineered metal-oxide nanoparticles, ACS Nano, 2012, 6(6), 5164 CrossRef CAS PubMed.
- M. Hayyan, M. A. Hashim and I. M. AlNashef, Superoxide ion: Generation and chemical implications, Chem. Rev., 2016, 116(5), 3029 CrossRef CAS.
- J. Yu, J. Chen, C. Li, X. Wang, B. Zhang and H. Ding, ESR signal of superoxide radical anion adsorbed on TiO2 generated at room temperature, J. Phys. Chem. B, 2004, 108(9), 2781 CrossRef CAS.
- S. Mumtaz, L. S. Wang, S. Z. Hussain, M. Abdullah, Z. Huma, Z. Iqbal, B. Creran, V. M. Rotello and I. Hussain, Dopamine coated Fe3O4 nanoparticles as enzyme mimics for the sensitive detection of bacteria, Chem. Commun., 2017, 53(91), 12306 RSC.
- D. Ma, A. Liu, S. Li, C. Lu and C. Chen, TiO2 photocatalysis for C–C bond formation, Catal. Sci. Technol., 2018, 8(8), 2030 RSC.
- W. L. Harrigan, S. E. Michaud, K. A. Lehuta and K. R. Kittilstved, Tunable electronic structure and surface defects in chromium-doped colloidal SrTiO3−δ nanocrystals, Chem. Mater., 2016, 28(2), 430 CrossRef CAS.
- Z. Sun, L. Zhang, F. Dang, Y. Liu, Z. Fei, Q. Shao, H. Lin, J. Guo, L. Xiang, N. Yerra and Z. Guo, Experimental and simulation-based understanding of morphology controlled barium titanate nanoparticles under co-adsorption of surfactants, CrystEngComm, 2017, 19(24), 3288 RSC.
- S. Adireddy, C. Lin, B. Cao, W. Zhou and G. Caruntu, Solution-Based Growth of Monodisperse Cube-Like BaTiO3 Colloidal
Nanocrystals, Chem. Mater., 2010, 22(6), 1946 CrossRef CAS.
- R. H. Mitchell, A. R. Chakhmouradian and P. M. Woodward, Crystal chemistry of perovskite-type compounds in the tausonite-loparite series, (Sr1−2xNaxLax)TiO3, Phys. Chem. Miner., 2000, 27(8), 583 CrossRef CAS.
- P. A. Giguère and I. D. Liu, On the infrared spectrum of hydrazine, J. Chem. Phys., 1952, 20(1), 136 CrossRef.
- E. J. Bowen and A. W. Birley, The vapour phase reaction between hydrazine and oxygen, Trans. Faraday Soc., 1951, 47, 580 RSC.
- K. van Benthem, C. Elsässer and R. H. French, Bulk electronic structure of SrTiO3: experiment and theory, J. Appl. Phys., 2001, 90(12), 6156 CrossRef CAS.
- K. A. Lehuta and K. R. Kittilstved, Reversible control of the chromium valence in chemically reduced Cr-doped SrTiO3 bulk powders, Dalton Trans., 2016, 45(24), 10034 RSC.
- F. Zuo, L. Wang, T. Wu, Z. Zhang, D. Borchardt and P. Feng, Self-doped Ti3+ enhanced photocatalyst for hydrogen production under visible light, J. Am. Chem. Soc., 2010, 132(34), 11856 CrossRef CAS PubMed.
- C. Mitra, C. Lin, J. Robertson and A. A. Demkov, Electronic structure of oxygen vacancies in SrTiO3 and LaAlO3, Phys. Rev. B: Condens. Matter Mater. Phys., 2012, 86(15), 155105 CrossRef.
- W. D. Rice, P. Ambwani, M. Bombeck, J. D. Thompson, G. Haugstad, C. Leighton and S. A. Crooker, Persistent optically induced magnetism in oxygen-deficient strontium titanate, Nat. Mater., 2014, 13(5), 481 CrossRef CAS PubMed.
- A. Henglein, Colloidal TiO2 catalyzed photo- and radiation chemical processes in aqueous solution, Ber. Bunsenges. Phys. Chem., 1982, 86(3), 241 CrossRef CAS.
- W. L. Harrigan and K. R. Kittilstved, Reversible modulation of the Cr3+ spin dynamics in colloidal SrTiO3 nanocrystals, J. Phys. Chem. C, 2018, 122(46), 26652 CrossRef CAS.
- E. Carter, A. F. Carley and D. M. Murphy, Evidence for O2− radical stabilization at surface oxygen vacancies on polycrystalline TiO2, J. Phys. Chem. C, 2007, 111(28), 10630 CrossRef CAS.
- B. Pallavi D, B. Deu S and G. Gavisiddappa S, Visible light active superoxide modified nanocrystalline anatase titania, J. Nanoeng. Nanomanuf., 2015, 5, 216 CrossRef.
- Z. Wei, D. Liu, W. Wei, X. Chen, Q. Han, W. Yao, X. Ma and Y. Zhu, Ultrathin TiO2(B) Nanosheets as the Inductive Agent for Transferring H2O2 into Superoxide Radicals, ACS Appl. Mater. Interfaces, 2017, 9(18), 15533 CrossRef CAS PubMed.
- A. L. Attwood, D. M. Murphy, J. L. Edwards, T. A. Egerton and R. W. Harrison, An EPR study of thermally and photochemically generated oxygen radicals on hydrated and dehydrated titania surfaces, Res. Chem. Intermed., 2003, 29(5), 449 CrossRef CAS.
- J. Green, E. Carter and D. M. Murphy, Interaction of molecular oxygen with oxygen vacancies on reduced TiO2: site specific blocking by probe molecules, Chem. Phys. Lett., 2009, 477(4–6), 340 CrossRef CAS.
- K. Komaguchi, T. Maruoka, H. Nakano, I. Imae, Y. Ooyama and Y. Harima, Electron-transfer reaction of oxygen species on TiO2 nanoparticles induced by sub-band-gap illumination, J. Phys. Chem. C, 2010, 114(2), 1240 CrossRef CAS.
- K. Komaguchi, T. Maruoka, H. Nakano, I. Imae, Y. Ooyama and Y. Harima, ESR study on the reversible electron transfer from O22− to Ti4+ on TiO2 nanoparticles induced by visible-light illumination, J. Phys. Chem. C, 2009, 113(4), 1160 CrossRef CAS.
- H. Möckel, M. Giersig and F. Willig, Formation of uniform size anatase nanocrystals from bis(ammonium lactato)titanium dihydroxide by thermohydrolysis, J. Mater. Chem., 1999, 9, 3051 RSC.
- G. A. Seisenbaeva, G. Daniel, J. M. Nedelec and V. G. Kessler, Solution equilibrium behind the room-temperature synthesis of nanocrystalline titanium dioxide, Nanoscale, 2013, 5(8), 3330 RSC.
- S. M. Bertolino, L. A. Melgaco, R. G. Sa and V. A. Leao, Comparing lactate and glycerol as a single-electron donor for sulfate reduction in fluidized bed reactors, Biodegradation, 2014, 25(5), 719 CrossRef CAS PubMed.
- V. Vandieken, N. Finke and B. Thamdrup, Hydrogen, acetate, and lactate as electron donors for microbial manganese reduction in a manganese-rich coastal marine sediment, FEMS Microbiol. Ecol., 2014, 87(3), 733 CrossRef CAS PubMed.
- S. Tsubakizaki, H. Gotou, N. Ishihara, M. Takada, K. Mawatari and R. Kai, Alternatives to hydrazine in water treatment at thermal power plants, Mitsubishi Heavy Ind. Tech. Rev., 2009, 46(2), 43 Search PubMed.
- B. Scrivenand and T. R. Winter, Chemical oxygen scavengers - Use of hydrazine and tannins for boiler water treatment, Anti-Corros. Methods Mater., 1978, 25(10), 10 CrossRef CAS.
Footnote |
† Electronic supplementary information (ESI) available. See DOI: 10.1039/d0na00106f |
|
This journal is © The Royal Society of Chemistry 2020 |