DOI:
10.1039/C9NA00756C
(Minireview)
Nanoscale Adv., 2020,
2, 991-1006
Graphene nanocrystals in CO2 photoreduction with H2O for fuel production
Received
3rd December 2019
, Accepted 15th January 2020
First published on 16th January 2020
Abstract
Graphene nanocrystals can utilize solar light and are valuable in cases where electricity is lacking due to their chemical stability during the photocatalytic process, low cost and non-toxicity. However, because of the large band gap, ultraviolet light irradiation can barely excite graphene, which limits its application in the environment. CO2 photoreduction through the visible light-responsive photocatalytic performance of graphene nanocrystals has recently been the focus of research in nanoscience due to the ability to convert pollutants into CO2 and H2O for environmental applications such as energy, environmental purification and wastewater treatment. This paper highlights the present improvements in CO2 photoreduction with H2O through the visible light-responsive photocatalytic performance of graphene nanocrystals via the development of structural modification strategies, solar harvesting, methods of synthesis and solar light catalytic mechanisms.
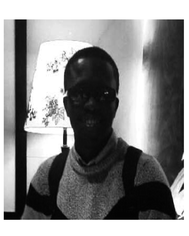 Williams Kweku Darkwah | Williams Kweku Darkwah is an MSc. student in the Environmental Engineering Department at the College of Environment, Hohai University, Nanjing, China under the supervision of Professor Yanhui Ao. He received his BSc. in Biochemistry from the University of Cape Coast, Cape Coast, Ghana. He was a recipient of the SLAS 2019 Prestigious Tony B Academic Award from the Society of Laboratory Automation and Screening, USA. His research interests are mainly focused on the photocatalysis-based remediation technology using nanomaterials. |
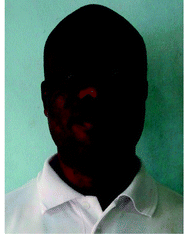 Godfred Kwesi Teye | Godfred Kwesi Teye is a PhD Candidate in the College of Environment, Hohai University, Nanjing City, China, under the supervision of Associate Professor Jingyu Huang and Professor Yi Li. He obtained his MSc in Sustainable Development and Climate Change and BSc in Environmental and Natural Resource Management from the University of Ghana. He also holds a Specialist certificate in Waste, Water and Environmental Management from the FIW Research Institute of Water and Waste Management, Aachen University, Germany as well as a Diploma and Certificate in Environmental Health and Sanitation from the School of Hygiene, Accra, Ghana. He also holds a Professional Certificate of Monitoring and Evaluation from the Ghana Institute of Management and Public Administration (GIMPA). His research area is mainly focused on transition metal-modified magnetite nanostructured photocatalysis, particularly for the degradation of pharmaceutical residues in water. |
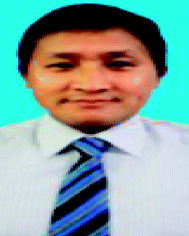 Yanhui Ao | Yanhui Ao, PhD, is working as a Professor in Hohai University, Nanjing, China. He received his PhD from Southeast University, Nanjing, China. During 2015–2016, he worked as a visiting scholar at Nanyang Technological University, Singapore. He was honoured by the Ministry of Education, China as one of the National Excellent Young Scientists. He has published more than 150 academic papers and also has more than 7 patents. His research interests are mainly focused on new photocatalysis-based remediation technologies using nanomaterials, water resource protection, behaviour of manufactured nanomaterials in the environment, and environment friendly materials. |
Introduction
Recently, activities such as transportation, industrial processes, and heating, among other main uses, have led to the consumption of large quantities of energy. This present situation is mainly centered on the substantial utilization of fossil fuels, including oil and natural gas, not only for transportation but also for the production of electricity and heat. Considering the limited resources of fossil fuels, it is clear that at the high current consumption rate, these resources will become depleted, making the present energy source scheme unsustainable.
Besides sustainability, a second driving force to develop alternative energy sources is climate change specifically atmospheric pollution due to greenhouse gases. CO2 accumulation has become a global crisis as it is the major product of fossil fuel combustion. It has been predicted that by 2050, the CO2 concentration in the atmosphere will reach over 500 ppm, which is nearly double the concentration present prior to the industrial revolution.1
CO2 is reduced with H2O to form useful products such as carbon monoxide (CO), formate, methanol, ethanol, or hydrocarbons. These molecules are converted or used directly as sustainable alternatives to fossil fuels for energy storage.139 CO2 photoreduction, particularly with H2O, is one promising solution compared to other methods, such as chemical, electrochemical and biological methods.140
Solar energy can be readily gathered, transformed and kept in the form of heat, which can either be distributed to residences or further converted into electricity as well as into other forms of energy.6 The most innovative investigated technologies concerning solar photon capture may be those via photocatalysis, as described by Edmond Becquerel in 1839.6
Fujishima and Honda revealed the exceptional knowledge about the photochemical splitting of water into hydrogen and oxygen in the presence of TiO2 in 1972; since then, research interest has been focused on heterogeneous photocatalysis.4–6 The increase in the rate of a photoreaction in the presence of a catalyst is described as photocatalysis. Photocatalytic reactions are best known to be carried out in media such as gas phases, pure organic liquid phases or aqueous solutions. Also, most photocatalytic reduction reactions involving photons and a catalyst are often identified as the best in controlling organic wastewater, solar energy utilization, and environmental treatment applications.5,6
Graphitic nanoparticles have become the prime candidates for CO2 reduction with H2O photocatalysis.7 Graphitic nanoparticles are visible light-responsive materials with outstanding band gaps, and the energy levels of CB and VB are optimally located with respect to the normal hydrogen electrode.7 In addition, these materials have the ability to resist heat as well as strongly acidic and strongly alkaline solutions.
Due to the outstanding properties of these nanoparticles, the use of these promising materials in water splitting, CO2 photoreduction with H2O, organic contaminant purification, catalytic organic synthesis, and fuel cells is more efficient and effective.7
This paper, however, highlights the capability and efficacy for the prospective applications of CO2 photoreduction with H2O through the visible light-responsive photocatalytic performance of graphene nanocrystals for energy and environmental treatment in order to design future implementations to solve the environmental problems related to fuel production. For this reason, these materials are recognized as attractive candidates for environmental uses such as purified fuel production and waste-water treatment.
Graphene
Graphene stands to be a promising 2D-carbon lattice in the field of material research due to its excellent chemical and physical properties.39 A large surface area, unique thermal and chemical stabilities, excellent mechanical strength, and superior electrical conductivity make graphene a potential component in various fields such as solar cells.
In recent years, graphene materials with novel compositions and well-defined structures are of particular interest due to their composition-dependent electronic, optical and catalytic properties.23,24 They have been widely used as efficient heterogeneous or homogeneous catalysts for a broad range of inorganic and organic reactions.25,26
Graphene is formed by the one-atom-thick planar sheets of sp2-bonded carbon atoms that are densely packed in a honeycomb crystal lattice.27,33 The derivatives of graphene contain a large number of oxygen-containing functional groups, such as –COOH and –OH.28,29 Graphene is one of the best base materials to solid-load AgNPs. Graphene can fix other metals in their laminar structure so that these metals or nanomaterials can exhibit good dispersion and it additionally plays a stabilizing and protective role.30–32
The use of graphene in phase change materials is more promising than other nanoparticles for enhancing the thermophysical properties of phase change materials.2,3,34,96,113,115–128 Graphene has the potential to outperform metal nanoparticles, carbon nanotubes, and other carbon allotropes as a filler in thermal management materials.35 The addition of 4% graphene to 1-octadecanol and erythritol (Fig. 1) leads to 140% increase in thermal conductivity. These improvements are markedly superior to that for other nanofillers such as multiwall carbon nanotubes and silver nanowires.36,37
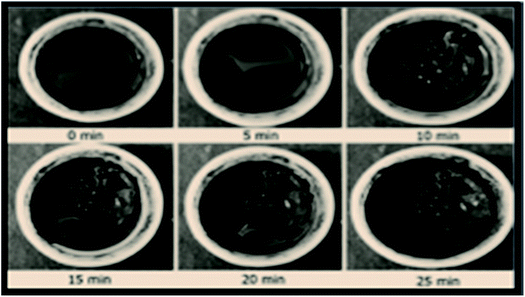 |
| Fig. 1 Solidification of erythritol with 1% graphitic nanoparticles. Reproduced with permission from Mayilvelnathan et al., 2019. Copyright 2019, Elsevier. | |
Composites of graphene
Graphene acts as a good electron acceptor and possesses transport properties. Metal/metal oxide–graphene composites absorb light on illumination and cause the photoexcitation of electrons. The excited electrons move from the valence band to the conduction band by electronic excitation between graphene and metal oxide. This process initiates the electron–hole pair charge separation between the metal oxide and graphene.43,96,101,112,148 Graphene is a potential electron acceptor and possesses a two-dimensional p-conjugation structure, which effectively suppresses the recombination of photogenerated e−–h+ pairs.49
Jiang et al. synthesized a novel ternary SnO2/Cu/graphene composite by a one-pot selective reduction method, in which N2H4·H2O was used as a reductant to realize a selective reduction fabrication process because its reduction strength falls in between these two components.40 The process abandons complicated mechanical mixing (such as high-energy ball milling) and the possibility of destroying the integrity of the composite structure; it can also realize the uniform distribution of active substances and conductive components. In the as-prepared ternary graphene composite by Jiang and co-workers, the electrochemically active SnO2 and inactive metallic Cu nanoparticles anchor tightly on flexible conductive graphene sheets and are located in close proximity to each other. Cu nanoparticles can promote the charge transfer kinetics of insulating SnO2 at the interfaces, compress the volume stress as lithium ion insertion/deinsertion, and obstruct the aggregation of metallic Sn and LixSn alloys. Thus, improved reversibility of the conversion reaction from Sn/Li2O to SnO2 is found in the SnO2/Cu/GNS composite (see Fig. 2). In summary, the results obtained from this study demonstrate that Cu nanoparticles are promising in promoting the reversibility of the conversion reaction and stabilizing the reversible capacity of SnO2–graphene-based composites.40
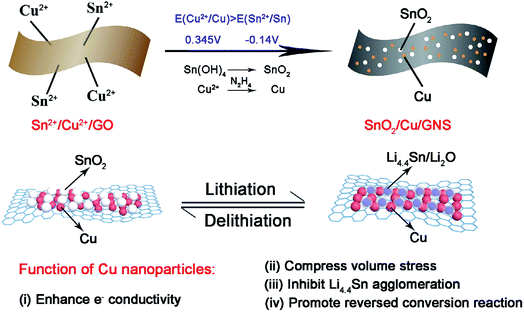 |
| Fig. 2 Schematic illustration of the selective reduction fabrication of SnO2/Cu/GNS composite; structure evolution and functions of Cu nanoparticles during lithiation and delithiation processes. Reproduced with permission from Jiang et al., 2019. Copyright 2019, Elsevier. | |
Carbon allotropes such as 0D carbon dots, 1D carbon nanotubes and 2D graphene have attracted significant interest in many fields including catalysis, new device structures and solar energy harvesting.41 Particularly, the discovery of graphene has raised a wave of new research on a range of 2D materials, e.g., phosphorene, transition metal dichalcogenides and hexagonal boron nitrides. Recently, graphdiyne (GDY) has emerged as a unique 2D carbon material and it consists of aromatic rings made by sp and sp2 carbons and carbon–carbon triple bonds linked by an acetylenic linkage.
Motivated by the multiple merits of GDY, Xu and co-workers reported a GDY-decorated TiO2 heterojunction prepared by the electrostatic-driven self-assembly of TiO2 nanofibers and GDY nanosheets for light capturing CO2 reduction. This work reveals that GYD can function as a highly effective co-catalyst for solar energy harvesting and may be used in other catalytic processes.41
The poor incorporation between graphene sheets and other nanoparticles has become a problem in the application of graphene-based composites.46–48 Graphene oxide was functionalized with triethylene tetramine (TETA) and then, reduced graphene oxide/Ag nanoparticle composites (rGO/Ag NPs) were prepared using TETA as the reductant via the hydrothermal method by Cheng et al. in 2019. rGO can be separated well by TETA and Ag NPs, and Ag NPs are dispersed on the surface of rGO and/or among the rGO sheets. Hence, the as-prepared rGO/Ag NP composites can be used as sensitive sensors for detecting traces of heavy metal ions except for certain deviations.
Han and co-workers modified a graphene foam electrode with Ag2+ NPs for detecting Hg using cyclic voltammetry (CV), and the limitation and sensitivity were 0.11 μM and 8.0 μA μM−1, respectively.43 In addition, the composites containing graphene and Ag NPs can be explored for use as glucose and quercetin sensors.44,45
Synthesis of graphene
The interesting etiological and electronic nature of graphene makes it possible to develop a method to deposit layers of graphitic nanoparticles in a controlled manner; hence, graphene can be obtained. Considerably, the benchmark particle for comparison is bulk graphene. Graphitic materials are mostly bulk resources with small surface areas when they are prepared or synthesized by the direct condensation of organic precursors.
The mineralization of mesoporous structures and the increase in the specific surface area help in fine-tuning the physicochemical properties, which then increases the photocatalytic performance of graphene.12 The nano-casting/replication of mesoporous silica matrices is the first method used to prepare graphite materials, such as carbon nitride (g-C3N4), which are famous for their cohort of the corresponding carbon nanostructures.10,11 Great efforts were then devoted to discover more innovative schemes for graphene modification, which was inspired by the hard template method. Liu and Cohen then discovered the soft template technique,8 and other graphene modification schemes such as acidic solution impregnation, ultrasonic dispersion, and chemical functionalization8,9 were also discovered. These methods described above are good signs of the principle of modifying the surface chemical properties and texture of graphene alone with its electronic potentials.
Thermal treatments such as physical vapor deposition (PVD),13 chemical vapor deposition,14 solvo-thermal method15 and solid-state reaction15 are used for their approachable processing steps and these serve as the basic techniques for graphene synthesis. Thermal oxidation exfoliation, ultra-sonic exfoliation and chemical exfoliation are well-known as the major exfoliation methods used for preparing graphitic nanomaterials.
The synthesis of AgRh bimetallic nanoparticles (AgRh BNPs) stabilized by graphene quantum dots (GQDs) and their exceptional catalytic activity in the reduction of 4-nitrophenol, 2,4-dinitrophenol and 4-nitrobenzene diazonium tetrafluoroborate and generation of hydroxyl radicals was studied. The fabrication of the AgRh BNP nanocomposites was achieved by mixing GQDs and sodium borohydride, followed by the addition of simple commercial Ag and Rh salts at 0 °C in water.16 AgRh BNPs exhibited exceptional catalytic functions due to the positive synergistic effect between the Ag and Rh atoms on GQDs,21,22 and their catalytic activity was better than those of both monometallic counterparts. This graphene exhibited outstanding generation of hydroxyl radicals17–20 and further oxidized MB to MB-OH via some positive synergistic effects between the Ag and Rh atoms on GQDs than those of other BNPs and pure Ag or Rh NPs (Fig. 3).
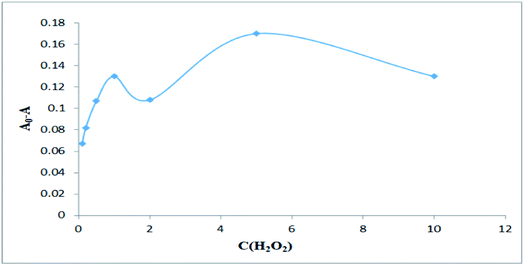 |
| Fig. 3 Effect of H2O2 on the generation of hydroxyl radicals from samples containing 3 mL of 1.5 × 10−4 mmol 4, 3.375 × 10−5 mmol methylene blue and H2O2 at different concentrations. Reproduced with permission from Li et al., 2019. Copyright 2019, Royal Society of Chemistry. | |
Ioni and co-workers presented a new approach for the preparation of graphene with noble metal nanoparticles on its surface using supercritical 2-propanol as a reducing agent for Pd or Pt graphene oxide nanocomposites. The prepared nanocomposites were characterized by X-ray diffraction analyses (XRD) and transmission electron microscopy (TEM). XRD reveals the face-centered cubic structure of Pd and Pt in the nanocomposites, and TEM images show the good spatial distribution of metal nanoparticles on layered graphene sheets.39
Photocatalysis
Studies define photocatalysis as one of the utmost environmental cleansing routines due to its potential to degrade refractory organic particles thoroughly by the use of sunlight or any visible light and its benefits of inferior energy utilization, no ancillary contamination, user-friendliness, etc. Graphene, as one of the best earliest established photocatalysts, has been well studied by scientists.111,112,114,115 Also, researchers have developed many other promising catalysts, such as nanocrystal particles, since the advancement of photocatalysis.116–121
Notably, there are many classes of reactions involved in the photocatalysis phenomenon by the use of a catalyst initiated by light (see Fig. 5), such as the breakdown of organic complexes into biologically reactive water and carbon dioxide, leading to the attractive features of surfaces enclosed with a photocatalyst as these protect against the coatings of fouling matter.
Studies have shown that nanocrystal graphene has more high-quality photocatalytic potential than many promising nanoparticles under light irradiation.118,134 The widespread application of nanocrystal graphene has been hampered due to its low separation efficiency of photo-excited electrons and holes. There have been many schemes to overcome these defects, such as forming a heterojunction structure via coupling with other semiconductors,120,121 doping with non-metals121,122 and depositing noble metals.122–124 These mechanisms can bring about high photocatalytic functioning. It is generally known that photocatalytic reactions usually take place on the surface of photocatalysts (Fig. 4). Subsequently, the surface environments of photocatalysts are fundamental to advance the photocatalytic activity for the calcination of organic pollutants. Inorganic oxyanions, for instance, phosphates, oxides, and metals have been used to modify photocatalysts and improve their performance.
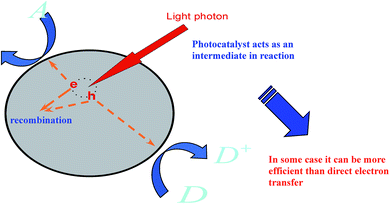 |
| Fig. 4 Scheme of redox reactions driven by light in a photocatalyst. | |
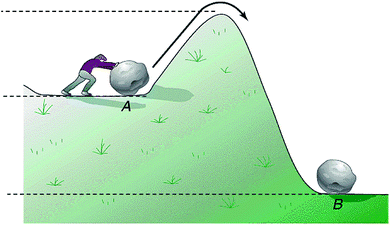 |
| Fig. 5 Activation of energy of photons by the catalyst in the presence of light. | |
The photocatalytic properties of graphene embedded in paints or concrete or positioned at the trivial of the particle can impact the high quality of these sustainable products.135–138 Photocatalysis is designed to harvest visible light by photocatalysts to drive chemical conversion.38,42
Mechanisms of photocatalysis
Gas and liquid phase photocatalysis
Numerous studies have revealed the efficacy of photocatalysis in gas and liquid phase remediation. Mostly, the catalytic route can be categorized into five distinct stages (Fig. 6): allocation of the reactants in the gas or liquid phase to the surface, adsorption of at least one of the reactants, reaction in the adsorbed phase, desorption of the product(s), and removal of the products from the interface region.
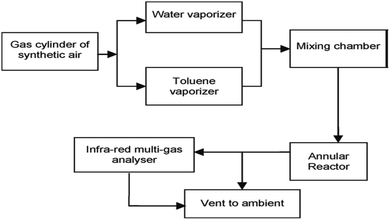 |
| Fig. 6 Flow diagram of photocatalytic reaction system set-up. Reproduced with permission from Adormaa et al., 2018. Copyright 2018, Royal Society of Chemistry. | |
The third step is where photocatalytic nature of the graphene plays major role, despite, all the steps are usually found in heterogeneous processes. The nanocrystal graphene is excited with a photon carrying energy equal or in excess of its band gap, producing an electron–hole pair related to the photoinduced electron transfer, and the absorption of light stimulates one electron into the conduction band. Graphene may transfer its electron to any adsorbed electron acceptor (thereby promoting its reduction), while the hole (or the electron vacancy) may accept an electron from an adsorbed donor (promoting its oxidation).
Basic principle of photocatalytic CO2 reduction
The basic principle of CO2 reduction with H2O photocatalysis occurs when the energy of the photons is enough to promote the electrons (see Fig. 5) in the valence band to jump to the conduction band. This occurs in three steps: (a) photon absorption and electron–hole pair generation,
(b) charge separation and migration to surface reaction sites or to recombination sites, and
(c) surface chemical reactions at the active sites containing donor oxidants at the valence-band holes and acceptor reductants at the electron center (Fig. 7). Numerous defects associated with these photocatalytic principles have been identified by researchers. During photocatalysis, cation radicals126 can be produced by injecting charges from an excited molecule into the conduction band of graphene (Fig. 4 and 5).
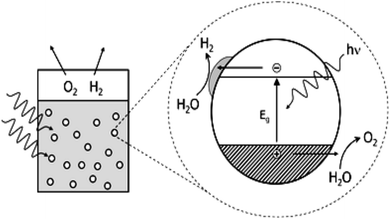 |
| Fig. 7 Photoredox catalysis by a photocatalyst. The oxidation steps are portrayed on the right; the reduction steps are shown on the left. | |
Irradiation is usually the initial process of CO2 reduction with H2O photocatalysis; thus, the excitation of electrons by photons at the ground state is the prerequisite. Periodically, the photoexcitation of electrons at the ground state also occurs in most of the materials adsorbed on the surface of graphene,127,128 for instance, the reaction occurring in dye-sensitized solar cells.129,131 Different pathways are mainly experienced by the charge carriers. Most individual graphene materials are primarily used for water splitting and oxidation/reduction (Fig. 8) in both suspension and electrode systems.132,133
 |
| Fig. 8 Mechanism of the photoreduction of CO2 with H2O to a methoxyl radical on TiO2 in the presence of water during the photocatalytic reaction. Reproduced with permission Adormaa et al., 2018. Copyright 2018, Royal Society of Chemistry. | |
CO2 reduction with H2O
Recently, the increased population as well as industrialization has been detrimental to the environment including the atmosphere.74,130 Recent increase in CO2 has remained to be a major issue on this planet.75,76 CO2 produced with H2O from burning fuels from the domestic to industrial level has contributed significantly to the atmospheric air pollution, resulting in the current global warming the world is experiencing.77–79,82 There has been an introduction of alternative advanced strategies to cut down the production of CO2. The SDG 7 has been identified for clean and renewable energy as one of the best mechanisms to reduce the production of CO2 with H2O in the atmosphere.80,81 However, due to the increasing demand of fuel and production on the industrial scale, the contribution of CO2 is still high. Technologies have been developed to reduce the amount of produced CO2. Among others, the photocatalytic reaction is one of the best technologies for CO2 reduction (Fig. 9).83
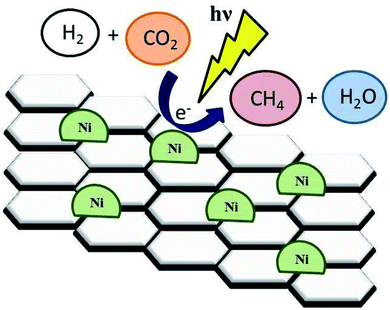 |
| Fig. 9 Illustration of the photoreduction of CO2 with H2O to CO. Reproduced with permission from Mateo et al., 2017. Copyright 2017, Elsevier. | |
Zhou and co-workers described the facile in situ synthesis of a graphene (g-C3N4)–N-TiO2 heterojunction as a competent photocatalyst for the selective photoreduction of CO2 with H2O to CO. The composites of graphene, i.e., carbon nitride and nitrogen-doped titanium dioxide composites (g-C3N4–N-TiO2) were in situ synthesized by the thermal treatment of well-mixed urea and Ti(OH)4 in an alumina crucible with a cover at different mass ratios. These results prove that graphene has high photocatalytic CO2 reduction with H2O as compared to its precursors.84
Photo-assisted CO2 reduction with H2O by H2 has been repeatedly described using fabrics covering noble or analytical metals, such as Pd, Ru and In.86–91 It was found that H2O formed in the photocatalytic reaction of NiO/Ni nanoparticles (NPs) supported on defective graphene via photo-assisted CO2 reduction with H2 has a negative influence on the photocatalytic activity and studies further confirmed that H2O desorption is one of the reasons why the system requires heating.85 Therefore, it is anticipated that a promising function of temperature in the system can be to promote H2O desorption from the photocatalyst surface, providing an opportunity for H2 and CO2 activation.72,85
Strategies for the photoreduction of CO2 with H2O
CO2 is the most oxidized form of carbon together with carbonate minerals with a formal oxidation state of +4. As a result, the conversion of CO2 into a more energetic product involves the transfer of electrons to carbon, reducing its oxidation state. The family of reactions by which CO2 is converted to a more reduced product is most often called CO2 reduction. These reactions are also often referred to as CO2 hydrogenation for thermally driven processes involving the reaction with hydrogen (H2) or CO2 fixation in natural photosynthesis and bioinspired catalysis.141
The ultimate source of electrons and protons for the reduction of CO2 must be water (H2O) as the combustion of a hydrogenated carbon product releases H2O. CO2 reduction/hydrogenation/fixation thus follows the following overall formula:
| xCO2 + yH2O → product + zO2, CO2 recycling reaction | (1) |
There are pathways in the traditional chemical industry, all thermally activated, that can be used for CO2 hydrogenation using H2 as the reductant.142,143 CO2 can be reduced to carbon monoxide (CO) by the reverse water gas shift reaction:
| CO2 + H2 → CO + H2O, reverse water–gas shift | (2) |
If excess H2 is used and the water is condensed out, the product gas is a mixture of H2 and CO, which is called synthesis gas or syngas. Syngas can be used as the precursor to methane on a Ni catalyst,144 multi-carbon hydrocarbons on an Fe or Co catalyst,145 or methanol on a Cu/ZnO catalyst;146,147 these reactions are called methanation, the Fischer–Tropsch reaction, and methanol synthesis, respectively.
| CO + 3H2 → CH4 + H2O, methanation | (3) |
| nCO + (2n + 1)H2 → CnH2n+2 + nH2O, Fischer–Tropsch | (4) |
The role of CO is to react with the water released by CO2 hydrogenation and generate more CO2 molecules via the water–gas shift reaction (reverse of reaction (2)). The net reaction is as follows:
| CO + 2H2 → CH3OH, water–gas shift plus methanol synthesis | (5) |
All of these processes run at high pressures and temperatures; for example, ∼100 bar and 250 °C are typical conditions for methanol synthesis, which is the mildest of the three reactions.142
In order for CO2 hydrogenation by the above-mentioned reactions to be a renewable process, hydrogen must come from water splitting using renewable energy: (4) in methanol synthesis, some CO molecules are required in the syngas stream because CO2 is actually the immediate reactant,
| 2H2O → O2 + 2H2, water splitting | (6) |
such that the combined reaction is in the form of reaction
(1) mentioned above. Water splitting would be driven photochemically using electrical energy from renewable sources such as wind and solar
120 according to the following half-reactions:
| 2H+ + 2e− → H2, H2 evolution reaction (HER) at the cathode | (7) |
| 2H2O → O2 + 4H+ + 4e−, O2 evolution reaction at the anode | (8) |
An alternative to CO production by the reverse water–gas shift reaction (reaction (2)) is the photoreduction of CO2 with H2O to CO:
| CO2 + 2H+ + 2e− → CO + H2O, photoreduction for CO production | (9) |
Photocatalytic reduction of CO2 to methanol (methanol oxidation reaction)
The photocatalytic conversion of CO2 with H2O into fuels such as methanol and formic acid by graphene and graphene oxides based on light irradiation at a specific coverage could decrease the amount of CO2 in the environment and meet the increasing demands for energy.93–95 Honda et al. stated for the first time that the photocatalytic reduction of CO2 with H2O into valuable chemicals can be achieved using various semiconductor-based catalytic materials.69 The conversion of CO2 by photocatalysis and artificial photosynthesis as well as electrochemical, chemical and biological approaches can not only alleviate the increasing levels of CO2 in the environment but also provide alternative fuels and valuable chemicals.97–100
Gusain and co-workers prepared reduced graphene oxide (rGO)–copper oxide nanocomposites via the covalent grafting of CuO nanorods on the rGO skeleton. This study explored the potential of the rGO–CuO nanocomposites for the reduction of CO2 into methanol under visible light irradiation. The pristine CuO nanorods demonstrated very low photocatalytic activity due to the fast recombination of charge carriers and yielded 175 μmol g−1 methanol although rGO–Cu2O and rGO–CuO revealed considerably better photocatalytic activities and yielded five (862 μmol g−1) and seven (1228 μmol g−1) times as much methanol, respectively. The superior photocatalytic activity of CuO in the rGO–CuO nanocomposites was credited to the slow recombination of charge carriers and the efficient transfer of photogenerated electrons through the rGO skeleton. This study further excludes the use of a scavenging donor.92
Recently, it was revealed that an rGO coating significantly increases the activity of Cu2O for CO2 photoreduction to CO, which is nearly six times higher than that for the optimized Cu2O.102 This was attributed to the slower electron–hole recombination, efficient charge transfer and protective function of rGO. Herein, CuO (Cu2+) nanorods grafted on rGO showed better photocatalytic activities than the rGO–Cu2O (Cu1+) nanocomposite.
Also, a facile combined method, sonothermal–hydrothermal method, was used to construct titanium dioxide (TiO2) nanoparticles on the surface of reduced graphene oxide (rGO) to form nanocomposites. There was improved photocatalytic CO2 reduction to methanol by the composites under UVA and visible irradiation, which suggested the modification in the band gap of the composite and the promotion of the separation of photogenerated carriers, yielding a methanol production rate of 2.33 mmol g−1 h−1 (ref. 103) (Fig. 10). From the study, it can be deduced that the photoreaction process occurs through the traditional mechanism of photogenerated electron transfer to rGO, while visible light CO2 reduction proceeds as a result of the charge transfer photoexcitation that directly produces electrons in rGO and holes in TiO2.
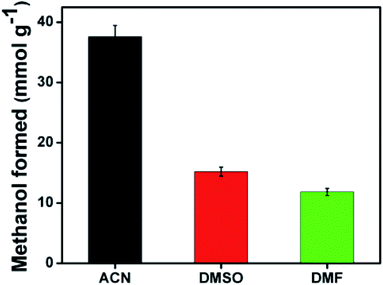 |
| Fig. 10 Influence of the reaction medium for the production of methanol from CO2 reduction under visible light by 5.0rGO–TiO2 for a period of 16 h. Reproduced with permission from Mungse et al., 2015. Copyright 2015, Royal Society of Chemistry. | |
Among the various applications of graphene, such as photocatalytic CO2 reduction,104,110 water splitting,105 dye and organic pollutant degradation,106,107 lithium-ion batteries,108 and antibacterial activity,109 photocatalytic CO2 reduction to valuable hydrocarbons via solar irradiation can serve as a solution to the total dependence on fossil fuels with its concomitant global warming.
CO2 methanation
CO2 methanation is an exothermic reaction involving CO2 as a substrate, which can be easily implemented in a thermal process to achieve maximum conversions and rates.67–71 Photo-assisted reactions are considered advantageous since they can be implemented in a range of temperatures, in which the thermal process does not happen or results in only low CO2 conversions because of the low reaction rates.72,73 In exothermic reactions, in order to shift the equilibrium towards the products, low reaction temperatures but with suitable rates are more appropriate, which can be provided by the photo-assisted process. CO2 methanation is one of the few exothermic reactions involving Ni/SiO2–Al2O3 as a catalyst at temperatures above 400 °C to reach high conversions and rates. Therefore, it is well-defined that H2O is a robust poison of photo-methanation and it is proposed that the role of temperature is to desorb this generated H2O acting as a poison from the catalyst surface.
It is obvious from Fig. 11 that the specific initial rate of CH4 formation is contingent on the irradiation power, suggesting that under the current conditions, CO2 methanation is a photo-assisted process.67
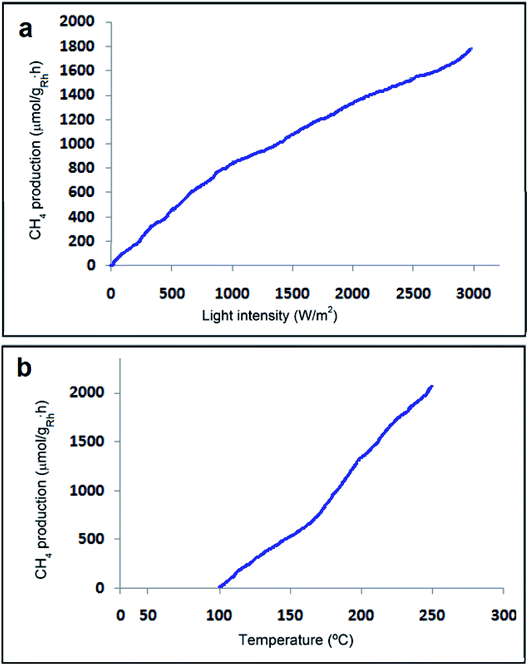 |
| Fig. 11 Specific CH4 formation rate of Rh (18)–G photocatalyst as a function of the light intensity (a) and temperature (b). The entire catalyst amount utilized in the light intensity experiment was 25 mg and 20 mg in the temperature experiment. Light intensity tests were carried out at a constant temperature of 175 °C, while the light intensity in the temperature dependency experiments was 2541 W m−2. PH2 = 1.00 bar, PCO2 = 0.25 bar in all cases. Reproduced with permission from Karach et al., 2018. Copyright 2018, Elsevier. | |
Recently, developing the photocatalytic activity of predictable semiconductors has attracted many researchers' attention and it has been stated that graphene as a useful additive can be provided to promote the photocatalytic activity. From the experiments of Karach et al., 2018, it has been established that Rh2O3/Rh NPs maintained on rGO can be an excellent photocatalyst for the methanation of CO2 at temperatures around 175 °C, achieving a specific CH4 formation rate of 814.38 μmol gRh−1 h−1, which is approximately double that of the estimated rate for Rh NPs supported on high-surface-area silica-alumina with a discernible quantum efficiency of 2.55%. It seemed that the photoinduced electron transfer from excited Rh2O3/Rh NPs to graphene sheets is responsible for the positive impact of graphene.
Photoelectrochemical CO2 reduction
Photoelectrochemical (PEC) CO2 reduction with H2O has been an innovation for artificial photosynthesis, which provides an effective route to relieve the environmental issues and energy crisis. Therefore, the development of effective catalytic materials with low energy consumption, controllable product selectivity and high quantum efficiency is an urgent concern for the PEC CO2 reduction with H2O.51–53
Graphene has been given considerable attention in many prospects of material science, such as solar cells,54,55 supercapacitors,56–58 and fuel cells.59,60 Due to the large specific surface area, chemical durability and excellent charge carrier mobility, graphene has become one of the most ideal carrier materials and electron collectors to promote the separation and transfer of photoinduced charge carriers.61,62 Also, graphene has been brought into focus in their optoelectronic applications due to the wide absorption range in the visible light region and long carrier diffusion length.63–65
The proposed possible photoelectrochemical mechanism of a graphene hybrid to achieve CO2 photoreduction with H2O is shown in Fig. 12. Graphene hybrids are the subject for photoelectrochemical CO2 reduction, from which the photoelectrons can be derived under solar-simulated light illumination. Graphene can be treated as a semiconductor due to the oxygen-containing groups on its surface.66 The sp2 carbon atoms function like a conduction band, while the sp3 carbon atoms serve as the valence band. After introducing a small amount of graphene into the hybrid, in addition to slightly enhanced light absorption, graphene mainly functions as an electron transfer medium to separate photogenerated electron–hole pairs, thus improving photocurrent.
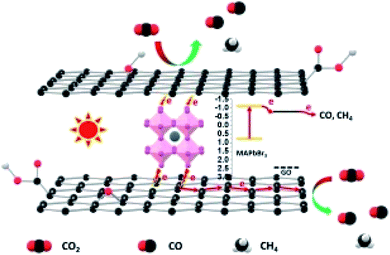 |
| Fig. 12 Possible mechanistic pathway of photoelectrochemical CO2 reduction. Reproduced with permission from Wang et al., 2018. Copyright 2018, Elsevier. | |
Wang and co-workers proposed that the in situ synthesized GO/MAPbBr3 hybrid was more beneficial to photoelectrochemical CO2 reduction with H2O than bare MAPbBr3. The yield of CO for the MAPbBr3 photoelectrochemical CO2 reduction was 0.268 μmol cm−2 h−1. After the hybridization of MAPbBr3 and GO, a significant improvement in the photoelectrochemical activity was obtained.50
Future prospects and conclusion
From the discussion, the prospective investigations of CO2 photoreduction with H2O through the visible light reactive photocatalytic performance of graphene nanocrystals may consider both designing and producing more effectual nanostructures, for example, hollow spheres with a high surface area or nanoplates with a highly active surface and the degradation performance and mechanism of additional categories of pollutants, particularly for non-dyed pollutants, better CO2 reduction with H2O and hydrogen production.
Also, future prospects should consider developing nanocrystals that are responsive to morphology monitoring, evaluating the photocatalytic practicality and efficacy of traditional synthesis and preparative strategies of graphene nanocrystal compounds and then discovering the functions of distinct graphene nanoparticles in the context of viable fuel production and its effective application in solar energy utilization.
The photocatalytic reduction of CO2 using H2O is a perfect approach for clean fuel production, but it remains challenging to design an extremely well-organized photocatalytic system by tracking the charge flow in a facile manner. Distinctive investigations have demonstrated the high photocatalytic activity of graphene over pollutant carbon dioxide reduction. Among others, graphene in recent times has presented photocatalytic activity towards organic pollutants, hydrogen production and carbon dioxide reduction.
Graphene nanocrystals are mostly used as co-catalysts to help improve the separation and transfer efficacy of electron–hole pairs to enhance the photocatalytic performances of the catalysts. Chen et al. (Qin et al. 2018; Wang et al., 2009) indicated that graphene nanocrystals coupled with nanoparticles, for instance, n-type graphitic carbon nitride, can usually form a p–n junction to promote charge separation and transfer for improved photocatalytic activity.
Graphene nanocrystals are very important in the management of the environment, especially those which are used in the photocatalytic processes. This was confirmed by (Deng et al. 2018; Lan et al., 2015) in their research, where they indicated that noble metals incorporated with graphene catalysts have significant application prospects in the chemical production processes and environmental protection fields.
Lastly, this review on CO2 photoreduction with H2O through the visible light-responsive photocatalytic performance of graphene nanocrystals highlights the current state of knowledge on the photocatalytic activity of these nanocrystals from different aspects of their structural alterations, synthesis, and catalytic capabilities. This mini-review offers a thoughtful perspective of the diverse means of solar harvesting with graphene nanocrystals for better visible light-responsive CO2 reduction activities. In light of this, further investigations are also needed in order to construct a complete picture of the exceptional properties of CO2 photoreduction with H2O through the visible light-responsive photocatalytic performance of graphene nanocrystals.
Conflicts of interest
The authors declares that they have no conflict of interest.
Acknowledgements
This work was financially supported by grants from National Science Funds for Creative Research Groups of China (No. 51421006), Natural Science Foundation of China (51679063), the Key Program of National Natural Science Foundation of China (No. 41430751), the National Key Plan for Research and Development of China (2016YFC0502203), Fundamental Research Funds (No. 2016B43814), and PAPD.
References
- M. Yuan, M. Kummer and S. Minteer, Strategies for Bioelectrochemical CO2 Reduction, Chem.–Eur. J., 2019 DOI:10.1002/chem.201902880.
-
M. Fox, Photocatalytic Oxidation of Organic Substances, in Photocatalysis and Environment: Trends and Applications, ed. Kluwer, New York Academic Publishers, 1988, pp. 445–467. Search PubMed.
- U. I. Gaya and A. H. Abdullah, Heterogeneous Photocatalytic Degradation of Organic Contaminants over Titanium Dioxide: A Review of Fundamentals, Progress and Problems, J. Photochem. Photobiol., C, 2008, 9, 1–12 CrossRef CAS.
- M. Umar and H. A. Aziz, Photocatalytic Degradation of Organic Pollutants in Water, Org. Pollut.: Monit., Risk Treat., 2006, 8, 195–208, DOI:10.5772/53699.
-
A. Fujishima and K. Honda, Electrochemical Photolysis of Water at a Semiconductor, 1972, pp. 3489–3491 Search PubMed.
- M. Boroski, A. C. Rodrigues, J. C. Garcia, L. S. Sampaio, J. Nozaki and N. Hioka, Combined Electrocoagulation and TiO2 Photo- assisted Treatment Applied to Wastewater Effluents from Pharmaceutical and Cosmetic Industries, J. Hazard. Mater., 2009, 162, 448–454 CrossRef CAS PubMed.
- X. Wang, K. Maeda, A. Thomas, K. Takanabe, G. Xin, J. M. Carlsson, K. Domen and M. Antonietti, A metal-free polymeric photocatalyst for hydrogen production from water under visible light, Nat. Mater., 2008, 8, 76 CrossRef PubMed.
- A. Y. Liu and M. L. Cohen, Prediction of new low compressibility solids, Science, 1989, 245(4920), 841–842 CrossRef CAS PubMed.
- M. Y. Chen, D. Li, X. Lin, V. P. Dravid, Y. W. Chung, M. S. Wong and W. D. Sproul, Analytical electron-microscopy and Raman-spectroscopy studies of carbon nitride thin-films, J. Vac. Sci. Technol., A, 1993, 11(3), 521–524 CrossRef CAS.
- Q. Yu, S. Guo, X. Li and M. Zhang, One-step fabrication and high photocatalytic activity of porous graphitic carbon nitride–graphene oxide hybrid by direct polymerization of cyanamide without templates, Russ. J. Phys. Chem. A, 2014, 88(10), 1643–1649 CrossRef CAS.
- Q. Yu, X. Li and M. Zhang, One-step fabrication and high photocatalytic activity of porous graphitic carbon nitride synthesized via direct polymerisation of dicyandiamide without templates, Micro Nano Lett., 2014, 9(1), 1–5 CrossRef.
- X. Li, J. Zhang, L. Shen, Y. Ma, W. Lei, Q. Cui and G. Zou, Preparation and characterization of graphitic carbon nitride through pyrolysis of melamine, Appl. Phys. A: Mater. Sci. Process., 2009, 94(2), 387–392 CrossRef CAS.
- R. Gago, I. Jimenez, D. Caceres, F. Agullo-Rueda, T. Sajavaara, J. M. Albella, A. Climent-Font, I. Vergara, J. Raisanen and E. Rauhala, Hardening mechanisms in graphitic carbon nitride films grown with N2/Ar ion assistance, Chem. Mater., 2001, 13(1), 129–135 CrossRef CAS.
- Y. Wang, F. Wang, Y. Zuo, X. Zhang and L. F. Cui, Simple synthesis of ordered cubic mesoporous graphitic carbon nitride by chemical vapor deposition method using melamine, Mater. Lett., 2014, 136, 271–273 CrossRef CAS.
- D. Lu, P. Fang, W. Wu, J. Ding, L. Jiang, X. Zhao, C. Li, M. Yang, Y. Li and D. Wang, Solvothermal-assisted synthesis of self-assembling TiO2 nanorods on large graphitic carbon nitride sheets with their anti-recombination in the photocatalytic removal of Cr(VI) and Rhodamine B under visible light irradiation, Nanoscale, 2017, 9(9), 3231–3245 RSC.
- N. Li, W. Chen, J. Shen, S. Chen and X. Liu, Synthesis of Graphene Quantum Dots Stabilized Bimetallic AgRh Nanoparticles and Their Applications, Inorg. Chim. Acta, 2019 DOI:10.1016/j.ica.2019.119031.
- J. Shi, C. Chan, Y. Pang, W. Ye, F. Tian, J. Lyu, Y. Zhang and M. Yang, A fluorescence resonance energy transfer (FRET) biosensor based on graphene quantum dots (GQDs) and gold nanoparticles (AuNPs) for the detection of mecA gene sequence of staphylococcus aureus, Biosens. Bioelectron., 2015, 67, 595–600 CrossRef CAS PubMed.
- X. Niu, Y. Zhong, R. Chen, F. Wang, Y. Liu and D. Luo, A “turn-on” fluorescence sensor for Pb2+ detection based on graphene quantum dots and gold nanoparticles, Sens. Actuators, B, 2018, 255(2), 1577–1581 CrossRef CAS.
- X. Liu and D. Astruc, Atomically precise copper nanoclusters and their applications, Coord. Chem. Rev., 2018, 359, 112–126 CrossRef CAS.
- X. Liu and D. Astruc, From galvanic to anti-galvanic synthesis of bimetallic nanoparticles and applications in catalysis, sensing, and materials science, Adv. Mater., 2017, 29(16), 1605305 CrossRef PubMed.
- J. Ju and W. Chen,
In situ growth of surfactant-free gold nanoparticles on nitrogen doped graphene quantum dots for electrochemical detection of hydrogen peroxide in biological environments, Anal. Chem., 2015, 87(3), 1903–1910 CrossRef CAS PubMed.
- R. M. Mohamed and E. S. Aazam, Novel Ag/YVO4 nanoparticles prepared by a hydrothermal method for photocatalytic degradation of methylene-blue dye, J. Ind. Eng. Chem., 2014, 20(6), 4377–4381 CrossRef CAS.
- V. R. Stamenkovic, B. Fowler, B. S. Mun, G. Wang, P. N. Ross, C. A. Lucas and N. M. Markovic, Improved oxygen reduction activity on Pt3Ni(111) via increased surface site availability, Science, 2007, 315(5811), 493–497 CrossRef CAS PubMed.
- F. Tao, M. E. Grass, Y. Zhang, D. R. Butcher, J. R. Renzas, Z. Liu, J. Y. Chung, B. S. Mun, M. Salmeron and G. A. Somorjai, Reaction-driven restructuring of Rh–Pd and Pt–Pd core-shell nanoparticles, Science, 2008, 322(5903), 932–934 CrossRef CAS PubMed.
- X. Liu, D. Wang and Y. Li, Synthesis and catalytic properties of bimetallic nanomaterials with various architectures, Nano Today, 2012, 7(5), 448–466 CrossRef CAS.
- M. B. Gawande, A. Goswami, T. Asefa, H. Guo, A. V. Biradar, D. Peng, R. Zboril and R. S. Varma, Core–shell nanoparticles: synthesis and applications in catalysis and electrocatalysis, Chem. Soc. Rev., 2015, 44, 7540–7559 RSC.
- A. K. Geim, Graphene: status and prospects, Science, 2009, 324, 1530–1534 CrossRef CAS PubMed.
- T. Kumeria, M. Bariana, T. Altalhi, M. Kurkuri, C. T. Gibson, W. Yang and D. Losic, Graphene oxide decorated diatom silica particles as new nano-hybrids: towards smart natural drug microcarriers, J. Mater. Chem. B, 2013, 1, 6302–6311 RSC.
- Z. Zang, X. Zeng, M. Wang, W. Hu, C. Liu and X. Tang, Tunable photoluminescence of water-soluble AgInZnS–graphene oxide (GO) nanocomposites and their application in vivo bioimaging, Sens. Actuators, B, 2017, 252, 1179–1186 CrossRef CAS.
- K. Chandraker, R. Nagwanshi, S. K. Jadhav, K. K. Ghosh and M. L. Satnami, Antibacterial properties of amino acid functionalized silver nanoparticles decorated on graphene oxide sheets, Spectrochim. Acta, Part A, 2017, 181, 47–54 CrossRef CAS PubMed.
- X. Wang, P. Huang, L. Feng, M. He, S. Guo, G. Shen and D. Cui, Green controllable synthesis of silver nanomaterials on graphene oxide sheets via spontaneous reduction, RSC Adv., 2012, 2, 3816–3822 RSC.
- J. Chen, L. Sun, Y. Cheng, Z. Lu, K. Shao, T. Li, C. Hu and H. Han, Graphene Oxide-Silver Nanocomposite: Novel agricultural antifungal agent against Fusarium graminearum for crop disease prevention, ACS Appl. Mater. Interfaces, 2016, 8(36) DOI:10.1021/acsami.6b05730.
- L. Gao, L. Wang and L. Yang,
et al., Preparation, characterization and antibacterial activity of silver nanoparticle/graphene oxide/diatomite composite, Appl. Surf. Sci., 2019 DOI:10.1016/j.apsusc.2019.04.153.
- T.-P. Teng, B.-G. Lin and Y.-Y. Yeh, Characterization of heat storage by nanocomposite-enhanced phase change materials, Adv. Mater. Res., 2011, 287–290(1), 1448–1455 CAS.
- D. H. Choi, J. Lee, H. Hong and Y. T. Kang, Thermal conductivity and heat transfer performance enhancement of phase change materials (PCM) containing carbon additives for heat storage application, Int. J. Refrig., 2014, 42, 112–120 CrossRef CAS.
- J. D. Renteria, D. L. Nika and A. A. Balandin, Graphene Thermal Properties: Applications in Thermal Management and Energy Storage, Appl. Sci., 2014, 4(4), 525–547 CrossRef.
- F. Yavari, H. R. Fard, K. Pashayi, M. A. Rafiee, A. Zamiri, Z. Yu, R. Ozisik, T. Borca-Tasciuc and N. Koratkar, Enhanced thermal conductivity in a nanostructured phase change composite due to low concentration graphene additives, J. Phys. Chem. C, 2011, 115(17), 8753–8758 CrossRef CAS.
- V. Mayilvelnathan and A. Valan Arasu, Characterisation and thermophysical properties of graphene nanoparticles dispersed erythritol PCM for medium temperature thermal energy storage applications, Thermochim. Acta, 2019 DOI:10.1016/j.tca.2019.03.03.
- Y. Ioni, E. Buslaeva and S. Gubin, Synthesis of graphene with noble metals nanoparticles on its surface, Mater. Today: Proc., 2016, 3, S209–S213 Search PubMed.
- Y. Jiang, Y. Wan, W. Jiang, H. Tao, W. Li, S. Huang, Z. Chen and B. Zhao, Stabilizing the reversible capacity of SnO2/graphene composites by Cu T nanoparticle, Chem. Eng. J., 2019, 367, 45–54 CrossRef CAS.
- F. Xu, K. Meng, B. Zhu, H. Liu, J. Xu and J. Yu, Graphdiyne: A New Photocatalytic CO2 Reduction Cocatalyst, Adv. Funct. Mater., 2019, 1904256, DOI:10.1002/adfm.201904256.
- Y. Cheng, H. Li, C. Fang, L. Ai, J. Chen, J. Su, Q. Zhang and Q. Fu, Facile synthesis of reduced graphene oxide/silver nanoparticles composites and their application for detecting heavy metal ions, J. Alloys Compd., 2019 DOI:10.1016/j.jallcom.2019.01.320.
- T. Han, J. L. Jin, C. X. Wang, Y. Y. Sun, Y. H. Zhang and Y. Q. Liu, Ag Nanoparticles-Modified 3D Graphene Foam for Binder-Free Electrodes of Electrochemical Sensors, Nanomaterials, 2017, 7, 40 CrossRef PubMed.
- S. M. Naghib, E. Parnian, H. Keshvari, E. Omidinia and M. E. Malek, Synthesis, characterization and electrochemical evaluation of polyvinylalchol/graphene oxide/silver nanocomposites for glucose biosensing application, Int. J. Electrochem. Sci., 2018, 13, 1013–1026 CrossRef CAS.
- Z. F. Yao, X. Yang, X. B. Liu, Y. Q. Yang, Y. J. Hu and Z. J. Zhao, Electrochemical quercetin sensor based on a nanocomposite consisting of magnetized reduced graphene oxide, silver nanoparticles and a molecularly imprinted polymer on a screen-printed electrode, Microchim. Acta, 2018, 185, 70 CrossRef PubMed.
- J. Mohammadnejad, F. Yazdian, M. Omidi, A. D. Rostami, B. Rasekh and A. Fathinia, Graphene oxide/silver nanohybrid: optimization, antibacterial activity and its impregnation on bacterial cellulose as a potential wound dressing based on GO–Ag nanocomposite-coated BC, Eng. Life Sci., 2018, 18, 298–330 CrossRef CAS.
- E. S. Orth, J. E. S. Fonsaca, S. H. Domingues, H. Mehl, M. M. Oliveira and A. J. G. Zarbin, Targeted thiolation of graphene oxide and its utilization as precursor for graphene/silver nanoparticles composites, Carbon, 2013, 61, 543–550 CrossRef CAS.
- Z. Q. Zhao, X. Chen, Q. Yang, J. H. Liu and X. J. Huang, Selective adsorption toward toxic metal ions results in
selective response: electrochemical studies on a polypyrrole/reduced graphene oxide nanocomposite, Chem. Commun., 2012, 48, 2180–2182 RSC.
- M. R. Gandhi, S. Vasudevan, A. Shibayama and M. Yamada, Graphene and Graphene-Based Composites: A Rising Star in Water Purification – A Comprehensive Overview, ChemistrySelect, 2016, 1, 4358–4385 CrossRef CAS.
- Q. Wang, L. Tao, X. Jiang, M. Wang and Y. Shen, Graphene Oxide Wrapped CH3NH3PbBr3 Perovskite Quantum dots Hybrid for Photoelectrochemical CO2 Reduction in Organic Solvents, Appl. Surf. Sci., 2018 DOI:10.1016/j.apsusc.2018.09.215.
- L. Yuan and Y.-J. Xu, Photocatalytic conversion of CO2 into value-added and renewable fuels, Appl. Surf. Sci., 2015, 342, 154–167 CrossRef CAS.
- M. Iqbal, Y. Wang, H. Hu, M. He, A. Hassan Shah, L. Lin, P. Li, K. Shao, A. Reda Woldu and T. He, Cu2O-tipped ZnO nanorods with enhanced photoelectrochemical performance for CO2 photoreduction, Appl. Surf. Sci., 2018, 443, 209–216 CrossRef CAS.
- A. Navaee and A. Salimi, Specific anion effects on copper surface through electrochemical treatment: Enhanced photoelectrochemical CO2 reduction activity of derived nanostructures induced by chaotropic anions, Appl. Surf. Sci., 2018, 440, 897–906 CrossRef CAS.
- A. Giuri, S. Masi, S. Colella, A. Listorti, A. Rizzo, A. Kovtun, S. Dell'Elce, A. Liscio and C. Esposito Corcione, Rheological and physical characterization of PEDOT: PSS/graphene oxide nanocomposites for perovskite solar cells, Polym. Eng. Sci., 2017, 57, 546–552 CrossRef CAS.
- A. Agresti, S. Pescetelli, L. Cinà, D. Konios, G. Kakavelakis, E. Kymakis and A. D. Carlo, Efficiency and Stability Enhancement in Perovskite Solar Cells by Inserting Lithium-Neutralized Graphene Oxide as Electron Transporting Layer, Adv. Funct. Mater., 2016, 26, 2686–2694 CrossRef CAS.
- Y. Xu, L. Wang, P. Cao, C. Cai, Y. Fu and X. Ma, Mesoporous composite nickel cobalt oxide/graphene oxide synthesized via a template-assistant co-precipitation route as electrode material for supercapacitors, J. Power Sources, 2016, 306, 742–752 CrossRef CAS.
- Y. Gao, Y. Wan, B. Wei and Z. Xia, Capacitive Enhancement Mechanisms and Design Principles of High-Performance Graphene Oxide-Based All-Solid-State Supercapacitors, Adv. Funct. Mater., 2018, 28, 1706721 CrossRef.
- Y. Xu, X. Li, G. Hu, T. Wu, Y. Luo, L. Sun, T. Tang, J. Wen, H. Wang and M. Li, Graphene oxide quantum dot-derived nitrogen-enriched hybrid graphene nanosheets by simple photochemical doping for high-performance supercapacitors, Appl. Surf. Sci., 2017, 422, 847–855 CrossRef CAS.
- D. He, H. Tang, Z. Kou, M. Pan, X. Sun, J. Zhang and S. Mu, Engineered Graphene Materials: Synthesis and Applications for Polymer Electrolyte Membrane Fuel Cells, Adv. Mater., 2017, 29, 1601741 CrossRef PubMed.
- J. Shui, M. Wang, F. Du and L. Dai, N-doped carbon nanomaterials are durable catalysts for oxygen reduction reaction in acidic fuel cells, Sci. Adv., 2015, 1, 1400129 CrossRef PubMed.
- D. Xu, B. Cheng, S. Cao and J. Yu, Enhanced photocatalytic activity and stability of Z-scheme Ag2CrO4–GO composite photocatalysts for organic pollutant degradation, Appl. Catal., B, 2015, 164, 380–388 CrossRef CAS.
- X. Li, J. Yu, S. Wageh, A. A. Al-Ghamdi and J. Xie, Graphene in Photocatalysis: A Review, Small, 2016, 12, 6640–6696 CrossRef CAS PubMed.
- S. Kazim, M. K. Nazeeruddin, M. Grätzel and S. Ahmad, Perovskite as Light Harvester: A Game Changer in Photovoltaics, Angew. Chem., Int. Ed., 2014, 53, 2812–2824 CrossRef CAS PubMed.
- M. He, X. Pang, X. Liu, B. Jiang, Y. He, H. Snaith and Z. Lin, Monodisperse Dual-Functional Upconversion Nanoparticles Enabled Near-Infrared Organolead Halide Perovskite Solar Cells, Angew. Chem., Int. Ed., 2016, 55, 4280–4284 CrossRef CAS PubMed.
- J. Hwang, R. R. Rao, L. Giordano, Y. Katayama, Y. Yu and Y. Shao-Horn, Perovskites in catalysis and electrocatalysis, Science, 2017, 358, 751–756 CrossRef CAS PubMed.
- G. Eda and M. Chhowalla, Chemically derived graphene oxide: towards large-area thin-film electronics and optoelectronics, Adv. Mater., 2010, 22, 2392–2415 CrossRef CAS PubMed.
- N. Karach, M. Hosseini, Z. Parsaee and R. Razavi, Novel high performance reduced graphene oxide based nanocatalyst decorated with Rh2O3/Rh NPs for CO2 photoreduction, J. Photochem. Photobiol., A, 2018 DOI:10.1016/j.jphotochem.2018.06.024.
- G. Du, S. Lim, Y. Yang, C. Wang, L. Pfefferle and G. L. Haller, Methanation of carbon dioxide on Ni-incorporated MCM-41 catalysts: The influence of catalyst pretreatment and study of steady-state reaction, J. Catal., 2007, 249, 370–379 CrossRef CAS.
- T. Inui, M. Funabiki, M. Suehiro and T. Sezume, Methanation of CO2 and CO on supported nickel-based composite catalysts, J. Chem. Soc., Faraday Trans. 1, 1979, 75, 787–802 RSC.
- Y. x. Pan, C. j. Liu and Q. Ge, Effect of surface hydroxyls on selective CO2 hydrogenation over Ni4/gamma-Al2O3: A density functional theory study, J. Catal., 2010, 272, 227–234 CrossRef CAS.
- S. Abello, C. Berrueco and D. Montane, High-loaded nickel–alumina catalyst for direct CO2 hydrogenation into synthetic natural gas (SNG), Fuel, 2013, 113, 598–609 CrossRef CAS.
- P. Frontera, A. Macario, M. Ferraro and P. Antonucci, Supported Catalysts for CO2 Methanation: A Review, Catalysts, 2017, 7, 59 CrossRef.
- H. Yang, X. Cui, X. Dai, Y. Deng and F. Shi, Carbon-catalysed reductive hydrogen atom transfer reactions, Nat. Commun., 2015, 6, 1–11 Search PubMed.
-
S. E. Manahan, The endangered Global Atmosphere, in Environmental Chemistry, Lewis Publisher, Boca Raton, Landon, New York, Washington D.C, 7th edn, 2000 Search PubMed.
- S. Chakravarty, A. Chikkatur, H. de Coninck, S. Pacala, R. Socolow and M. Tavoni, Sharing global CO2 emission reductions among one billion high emitters, Proc. Natl. Acad. Sci. U. S. A., 2009, 106(29), 11884–11888 CrossRef CAS PubMed.
- J. Rogelj,
et al., Energy system transformations for limiting end-of-century warming to below 1.5 °C, Nat. Clim. Change, 2015, 5(6), 519–527 CrossRef.
- L. A. Duguma, P. A. Minang and M. van Noordwijk, “Climate Change Mitigation and Adaptation in the Land Use Sector: From Complementarity to Synergy, Environ. Manage., 2014, 54, 420–432 CrossRef PubMed.
- Y. Xu and V. Ramanathan, Well below 2 °C: Mitigation strategies for avoiding dangerous to catastrophic climate changes, Proc. Natl. Acad. Sci. U. S. A., 2017, 114(39), 201618481 CrossRef PubMed.
- B. Solano Rodriguez, P. Drummond and P. Ekins, Decarbonizing the EU energy system by 2050: an important role for BECCS, Clim. Pol., 2017, 17, S93–S110 CrossRef.
- J. Fuglestvedt,
et al., Implications of possible interpretations of ‘greenhouse gas balance’ in the Paris Agreement, Philos. Trans. R. Soc., A, 2018, 376(2119), 20160445 CrossRef PubMed.
- V. Duscha, A. Denishchenkova and J. Wachsmuth, Achievability of the Paris Agreement targets in the EU: demand-side reduction potentials in a carbon budget perspective, Clim. Pol., 2018, 3062, 1–14 Search PubMed.
- W. Yu, D. Xu and T. Peng, Enhanced photocatalytic activity of g-C3N4 for selective CO2 reduction to CH3OH via facile coupling of ZnO: A direct Z-scheme mechanism, J. Mater. Chem. A, 2015, 3(39), 19936–19947 RSC.
- Y. He,
et al., Z-scheme SnO2−x/g-C3N4 composite as an efficient photocatalyst for dye degradation and photocatalytic CO2 reduction, Sol. Energy Mater. Sol. Cells, 2015, 137, 175–184 CrossRef CAS.
- S. Zhou,
et al., Facile in situ synthesis of graphitic carbon nitride (g-C3N4)–N-TiO2 heterojunction as an efficient photocatalyst for the selective photoreduction of CO2 to CO, Appl. Catal., B, 2014, 158–159, 20–29 CrossRef CAS.
- D. Mateo, J. Albero and H. García, Graphene supported NiO/Ni nanoparticles as efficient photocatalyst for gas phase CO2 reduction with hydrogen, Appl. Catal., B, 2017 DOI:j.apcatb.2017.10.071.
- M. Li, P. Li, K. Chang, T. Wang, L. Liu, Q. Kang, S. Ouyang and J. Ye, Highly efficient and stable photocatalytic reduction of CO2 to CH4 over Ru loaded NaTaO3, Chem. Commun., 2015, 51, 7645–7648 RSC.
- X. Meng, T. Wang, L. Liu, S. Ouyang, P. Li, H. Hu, T. Kako, H. Iwai, A. Tanaka and J. Ye, Photothermal Conversion of CO2 into CH4 with H-2 over Group VIII Nanocatalysts: An Alternative Approach for Solar Fuel Production, Angew. Chem., Int. Ed., 2014, 53, 11478–11482 CrossRef CAS PubMed.
- J. Ren, S. Ouyang, H. Xu, X. Meng, T. Wang, D. Wang and J. Ye, Targeting Activation of CO2 and H-2 over Ru-Loaded Ultrathin Layered Double Hydroxides to Achieve Efficient Photothermal CO2 Methanation in Flow-Type System, Adv. Energy Mater., 2017, 7 DOI:10.1002/aenm.201770022.
- K. K. Ghuman, L. B. Hoch, P. Szymanski, J. Y. Y. Loh, N. P. Kherani, M. A. E-Sayed, G. A. Ozin and C. V. Singh, Photoexcited Surface Frustrated Lewis Pairs for Heterogeneous Photocatalytic CO2 Reduction, J. Am. Chem. Soc., 2016, 138, 1206–1214 CrossRef CAS PubMed.
- J. Jia, P. G. O'Brien, L. He, Q. Qiao, T. Fei, L. M. Reyes, T. E. Burrow, Y. Dong, K. Liao, M. Varela, S. J. Pennycook, M. Hmadeh, A. S. Helmy, N. P. Kherani, D. D. Perovic and G. A. Ozin, Visible and Near-Infrared Photothermal Catalyzed Hydrogenation of Gaseous CO2 over Nanostructured Pd@Nb2O5, Adv. Sci., 2016, 3, 1600189 CrossRef PubMed.
- P. G. O'Brien, A. Sandhel, T. E. Wood, A. A. Jelle, L. B. Hoch, D. D. Perovic, C. A. Mims and G. A. Ozin, Photomethanation of Gaseous CO2 over Ru/Silicon Nanowire Catalysts with Visible and Near-Infrared Photons, Adv. Sci., 2014, 1, 10.1002/advs.201400001 Search PubMed.
- R. Gusain, P. Kumar, O. P. Sharma, S. L. Jain and O. P. Khatri, Reduced graphene oxide-CuO nanocomposites for photocatalytic conversion of CO2 into methanol under visible light irradiation, Appl. Catal., B, 2015 DOI:j.apcatb.2015.08.012.
- K. Li, X. An, K. H. Parka, M. Khraisheh and J. Tang, A critical review of CO2 photoconversion: Catalysts and reactors, Catal. Today, 2014, 224, 3–12 CrossRef CAS.
- H. C. Hsu, I. Shown, H. Y. Wei, Y. C. Chang, H. Y. Du, Y. G. Lin, C. A. Tseng, C. H. Wang, L. C. Chen, Y. C. Lin and K. H. Chen, Graphene oxide as a promising photocatalyst for CO2 to methanol conversion, Nanoscale, 2013, 5, 262–268 RSC.
- F. Sastre, A. V. Puga, L. Liu, A. Corma and H. Garcia, Complete Photocatalytic Reduction of CO2 to Methane by H2 under Solar Light Irradiation, J. Am. Chem. Soc., 2014, 136, 6798–6801 CrossRef CAS PubMed.
- T. Inoue, A. Fujishima, S. Konishi and K. Honda, Photoelectrocatalytic reduction of carbon dioxide in aqueous suspensions of semiconductor
powders, Nature, 1979, 277, 637–638 CrossRef CAS.
- M. Mikkelsen, M. Jorgensen and F. C. Krebs, The teraton challenge. A review of fixation and transformation of carbon dioxide, Energy Environ. Sci., 2010, 3, 43–81 RSC.
- C. Costentin, M. Robert and J. M. Saveant, Catalysis of the electrochemical reduction of carbon dioxide, Chem. Soc. Rev., 2013, 42, 2423–2436 RSC.
- A. T. Najafabadi, CO2 chemical conversion to useful products: An engineering insight to the latest advances toward sustainability, Int. J. Energy Res., 2013, 37, 485–499 CrossRef.
- V. P. Indrakanti, J. D. Kubicki and H. H. Schobert, Photoinduced activation of CO2 on Ti-based heterogeneous catalysts: Current state, chemical physics-based insights and outlook, Energy Environ. Sci., 2009, 2, 745–758 RSC.
- S. Wang and X. Wang, Photocatalytic CO2 reduction by CdS Promoted with a Zeolitic Imidazolate Framework, Appl. Catal., B, 2015, 162, 494–500 CrossRef CAS.
- H. P. Mungse, R. Singh, H. Sugimura, N. Kumar and O. P. Khatri, Molecular Pillar Supported Graphene Oxide Framework: Conformational Heterogeneity and Tunable d-spacing, Phys. Chem. Chem. Phys., 2015 10.1039/c5cp02313k.
- J. O. Olowoyo, M. Kumar, B. Singh, V. O. Oninla, J. O. Babalola, H. é. Valdés, A. V. Vorontsov and U. Kumar, Self-assembled reduced graphene oxide-TiO2 nanocomposites: Synthesis, DFTB+ calculations, and enhanced photocatalytic reduction of CO2 to methanol, Carbon, 2019 DOI:10.1016/j.carbon.2019.03.019.
- W. Tu, Y. Zhou, Q. Liu, Z. Tian and J. Gao,
et al., Robust hollow spheres consisting of alternating titania nanosheets and graphene nanosheets with high photocatalytic activity for CO2 conversion into renewable fuels, Adv. Funct. Mater., 2012, 22, 1215–1221 CrossRef CAS.
- P. F. Wang, S. H. Zhan, Y. G. Xia, S. L. Ma, Q. X. Zhou and Y. Li, The fundamental role and mechanism of reduced graphene oxide in rGO/Pt-TiO2 nanocomposite for high-performance photocatalytic water splitting, Appl. Catal., B, 2017, 207, 335–346 CrossRef CAS.
- C. Wang, D. L. Meng, J. H. Sun, J. Memon, Y. Huang and J. X. Geng, Graphene wrapped TiO2 based catalysts with enhanced photocatalytic activity, Adv. Mater. Interfaces, 2014, 1, 1300150 CrossRef.
- X. Y. Wang, J. Wang, X. L. Dong, F. Zhang and L. G. Ma,
et al., Synthesis and catalytic performance of hierarchical TiO2 hollow sphere/reduced graphene oxide hybrid nanostructures, J. Alloys Compd., 2016, 656, 181–188 CrossRef CAS.
- M. M. Zhen, X. H. Zhu, X. Zhang, Z. Zhou and L. Liu, Reduced graphene oxide-supported TiO2 fiber bundles with mesostructures as anode materials for lithium-ion batteries, Chem.–Eur. J., 2015, 21, 14454–14459 CrossRef CAS PubMed.
- O. Akhavan and E. Ghaderi, Photocatalytic reduction of graphene oxide nanosheets on TiO2 thin film for photoinactivation of bacteria in solar light irradiation, J. Phys. Chem. C, 2009, 113, 20214–20220 CrossRef CAS.
- L. L. Tan, W. J. Ong, S. P. Chai and A. R. Mohamed, Noble metal modified reduced graphene oxide/TiO2 ternary nanostructures for efficient visible-light-driven photoreduction of carbon dioxide into methane, Appl. Catal., B, 2015, 166, 251–259 CrossRef.
- H. Tong, S. X. Ouyang, Y. P. Bi, N. Umezawa, M. Oshikiri and J. H. Ye, Nano-photo- catalytic materials: possibilities and challenges, Adv. Mater., 2012, 24(2), 229–251 CrossRef CAS PubMed.
- J. Schneider, M. Matsuoka, M. Takeuchi, J. L. Zhang, Y. Horiuchi, M. Anpo and D. W. Bahnemann, Understanding TiO2 photocatalysis: mechanisms and materials, Chem. Rev., 2014, 114(19), 9919–9986 CrossRef CAS PubMed.
- W. K. Darkwah and Y. Ao, Mini Review on the structural and properties (photocatalysis), preparation techniques of graphite Carbon Nitride (g-C3N4) Nano-Based particle, Nanoscale Res. Lett., 2018, 13, 388, DOI:10.1186/s11671-018-2702-3.
- Y. Ma, X. L. Wang, Y. S. Jia, X. B. Chen, H. X. Han and C. Li, Titanium dioxide-based nanomaterials for photocatalytic fuel generations, Chem. Rev., 2014, 114(19), 9987–10043 CrossRef CAS PubMed.
- S. W. Cao, J. X. Low, J. G. Yu and M. Jaroniec, Polymeric photocatalysts based on graphitic carbon nitride, Adv. Mater., 2015, 27(13), 2150–2176 CrossRef CAS PubMed.
- X. Yang, H. Li, W. Zhang, M. X. Sun, L. Q. Li, N. Xu, J. D. Wu and J. Sun, High visible photoelectrochemical activity of Ag nanoparticle-sandwiched CdS/Ag/ZnO nanorods, ACS Appl. Mater. Interfaces, 2017, 9(1), 658–667 CrossRef CAS PubMed.
- R. J. Hou, Y. Gao, H. J. Zhu, G. X. Yang, W. H. Liu, Y. N. Huo, Z. L. Xie and H. X. Li, Coupling system of Ag/BiOBr photocatalysis and direct contact membrane distillation for complete purification of N-containing dye wastewater, Chem. Eng. J., 2017, 317, 386–393 CrossRef CAS.
- C. S. Pan and Y. F. Zhu, New type of BiPO4 oxy-acid salt photocatalyst with high photocatalytic activity on degradation of dye, Environ. Sci. Technol., 2010, 44(14), 5570–5574 CrossRef CAS PubMed.
- S. Obregón, Y. F. Zhang and G. Colón, Cascade charge separation mechanism by ternary heterostructured BiPO4/TiO2/g-C3N4 photocatalyst, Appl. Catal., B, 2016, 184, 96–103 CrossRef.
- G. Q. Tan, L. N. She, T. Liu, C. Xu, H. J. Ren and A. Xia, Ultrasonic chemical synthesis of hybrid mpg-C3N4/BiPO4 heterostructured photocatalysts with improved visible light photocatalytic activity, Appl. Catal., B, 2017, 207, 120–133 CrossRef CAS.
- Y. F. Liu, Y. H. Lv, Y. Y. Zhu, D. Liu, R. L. Zong and Y. F. Zhu, Fluorine mediated photo- catalytic activity of BiPO4, Appl. Catal., B, 2014, 147(14), 851–857 CrossRef CAS.
- P. Zhang, H. H. Yu, J. J. Li, H. Zhao, B. L. Zhu, W. P. Huang and S. M. Zhang, Au/BiPO4 nanorod catalysts: synthesis, characterization and their catalytic performance for CO oxidation, RSC Adv., 2016, 6(19), 15304–15312 RSC.
- S. Y. Chen, R. Yan, X. L. Zhang, K. Hu, Z. J. Li, M. Humayun, Y. Qu and L. Q. Jing, Photogenerated electron modulation to dominantly induce efficient 2,4-di- chlorophenol degradation on BiOBr nanoplates with different phosphate modification, Appl. Catal., B, 2017, 209, 320–328 CrossRef CAS.
- Z. J. Li, Y. Qu, K. Hu, M. Humayun, S. Y. Chen and L. Q. Jing, Improved photoelectrocatalytic activities of BiOCl with high stability for water oxidation and MO degradation by coupling rGO and modifying phosphate groups to prolong carrier lifetime, Appl. Catal., B, 2017, 203, 355–362 CrossRef CAS.
- L. Q. Jing, J. Zhou, J. R. Durrant, J. W. Tang, D. N. Liu and H. G. Fu, Dynamics of photogenerated charges in the phosphate modified TiO2 and the enhanced activity for photoelectrochemical water splitting, Energy Environ. Sci., 2012, 5(4), 6552–6558 RSC.
- C. Nasr,
et al., Environmental photochemistry on semiconductor surfaces. Visible light induced degradation of a textile diazo dye, naphthol blue black, on TiO2 nanoparticles, J. Phys. Chem., 1996, 100, 8436–8442 CrossRef CAS.
- B. B. Adormaa, W. K. Darkwah and Y. Ao, Oxygen vacancies of the TiO2 nano-based composite photocatalysts in visible light responsive photocatalysis, RSC Adv., 2018, 8, 33551 RSC.
- Y. Nosaka and A. Y. Nosaka, Generation and Detection of Reactive Oxygen Species in Photocatalysis, Chem. Rev., 2017, 117, 11302–11336 CrossRef CAS.
- D. Hollmann, M. Karnahl, S. Tschierlei, K. Kailasam, M. Schneider, J. Radnik, K. Grabow, U. Bentrup, H. Junge and M. Beller,
et al., Structure-Activity Relationships in Bulk Polymeric and Sol–Gel-Derived Carbon Nitrides during Photocatalytic Hydrogen Production, Chem. Mater., 2014, 26, 1727–1733 CrossRef CAS.
- W. K. Darkwah and K. A. Oswald, Photocatalytic Applications of Heterostructure Graphitic Carbon Nitride: Pollutant Degradation, Hydrogen Gas Production (water splitting), and CO2 Reduction, Nanoscale Res. Lett., 2019 DOI:10.1186/s11671-019-3070-3.
- T. Li, L. Zhao, Y. He, J. Cai, M. Luo and J. Lin, Synthesis of g-C3N4/SmVO4 composite photocatalyst with improved visible light photocatalytic activities in RhB degradation, Appl. Catal., B, 2013, 129, 255–263 CrossRef CAS.
- M. R. Gholipour, C.-T. Dinh, F. Bélandb and T.-O. Do, Nanocomposite heterojunctions as sunlight-driven photocatalysts for hydrogen production from water splitting, Nanoscale, 2015, 7, 8187–8208 RSC.
- I. Nakamura, N. Negishi, S. Kutsuna, T. Ihara, S. Sugihara and K. Takeuchi, Role of oxygen vacancy in the plasma-treated TiO photocatalyst with visible light activity for NO removal, J. Mol. Catal. A: Chem., 2000, 161, 205–212 CrossRef CAS.
- W. K. Darkwah, B. B. Adormaa, M. K. C. Sandrine and Y. Ao, Modification Strategies for Enhancing Visible Light Responsive Photocatalytic activity of BiPO4 Nano base composite Photocatalyst, Catal. Sci. Technol., 2019 10.1039/c8cy02039f.
- X. Wang, K. Maeda, A. Thomas, K. Takanabe, G. Xin, J. M. Carlsson, K. Domen and M. Antonietti, A metal-free polymeric photocatalyst for hydrogen production from water under visible light, Nat. Mater., 2009, 8, 76–80 CrossRef CAS PubMed.
- Y. Lan, X. Li, G. Li and Y. Luo, Sol-gel method to prepare graphene/Fe2O3 aerogel and its catalytic application for the thermal decomposition of ammonium perchlorate, J. Nanopart. Res., 2015, 17, 395 CrossRef.
- Z. Qin, M. Wang, R. Li and Y. Chen, Novel Cu3P/g-C3N4 p–n Heterojunction Photocatalysts for Solar Hydrogen Generation, Sci. China Mater., 2018, 61(6), 861–868 CrossRef CAS.
- X. Deng, L. Zhu, H. Zhang, A. Kroner, J. Zheng, N. Zhang, J. He and B. Hui, Ruthenium Stabilized on Transition Metal-on-Transition Metal Oxide Nanoparticles for Naphthalene Hydrogenation, Int. J. Hydrogen Energy, 2018, 1–9 Search PubMed.
- S. Nitopi, E. Bertheussen, S. B. Scott, X. Liu and A. K. Engstfeld,
et al., Progress and Perspectives of Electrochemical CO2 Reduction on Copper in Aqueous Electrolyte, Chem. Rev., 2019, 119, 7610–7672 CrossRef CAS PubMed.
- N. Shehzad, M. Tahir, K. Johari, T. Murugesan and M. Hussain, A critical review on TiO2 based photocatalytic CO2 reduction system: Strategies to improve efficiency, J. CO2 Util., 2018, 26, 98–122 CrossRef CAS.
- A. M. Appel, J. E. Bercaw, A. B. Bocarsly, H. Dobbek, D. L. DuBois, M. Dupuis, J. G. Ferry, E. Fujita, R. Hille and P. J. A. Kenis,
et al., Frontiers, Opportunities, and Challenges in Biochemical and Chemical Catalysis of CO2 Fixation, Chem. Rev., 2013, 113, 6621–6658 CrossRef CAS PubMed.
-
M. Eckert, G. Fleischmann, R. Jira, H. M. Bolt and K. Golka, Ullmann's Encyclopedia of Industrial Chemistry, Wiley-VCH Verlag GmbH & Co. KGaA, Weinheim, Germany, 2000 Search PubMed.
-
I. Chorkendorff and J. W. Niemantsverdriet, Concepts of Modern Catalysis and Kinetics, Wiley-VCH Verlag GmbH & Co. KGaA, Weinheim, FRG, 2003 Search PubMed.
- S. Rönsch, J. Schneider, S. Matthischke, M. Schlülter, M. Götz, J. Lefebvre, P. Prabhakaran and S. Bajohr, Review on Methanation – From Fundamentals to Current Projects, Fuel, 2016, 166, 276–296 CrossRef.
- J. Yang, W. Ma, D. Chen, A. Holmen and B. H. Davis, Fischer–Tropsch Synthesis: A Review of the Effect of CO Conversion on Methane Selectivity, Appl. Catal., A, 2014, 470, 250–260 CrossRef CAS.
- S. G. Jadhav, P. D. Vaidya, B. M. Bhanage and J. B. Joshi, Catalytic Carbon Dioxide Hydrogenation to Methanol: A Review of Recent Studies, Chem. Eng. Res. Des., 2014, 92, 2557–2567 CrossRef CAS.
-
G. A. Olah, A. Goeppert and G. K. S. Prakash, Beyond Oil and Gas: The Methanol Economy, 2nd edn, 2009 Search PubMed.
- Z. W. Seh, J. Kibsgaard, C. F. Dickens, I. Chorkendorff, J. K. Nørskov and T. F. Jaramillo, Combining Theory and Experiment in Electrocatalysis: Insights into Materials Design, Science, 2017, 355, eaad4998 CrossRef PubMed.
|
This journal is © The Royal Society of Chemistry 2020 |