DOI:
10.1039/C9LC00949C
(Paper)
Lab Chip, 2020,
20, 155-167
PDMS filter structures for size-dependent larval sorting and on-chip egg extraction of C. elegans†
Received
23rd September 2019
, Accepted 22nd November 2019
First published on 28th November 2019
Abstract
C. elegans-based assays require age-synchronized populations prior to experimentation to achieve standardized sets of worm populations, due to which age-induced heterogeneous phenotyping effects can be avoided. There have been several approaches to synchronize populations of C. elegans at certain larval stages; however, many of these methods are tedious, complex and have low throughput. In this work, we demonstrate a polydimethylsiloxane (PDMS) microfluidic filtering device for high-throughput, efficient, and extremely rapid sorting of mixed larval populations of C. elegans. Our device consists of three plasma-activated and bonded PDMS parts and permits sorting of mixed populations of two consecutive larval stages in a matter of minutes. After sorting, we also retain the remaining larval stage of the initially mixed worm population on the chip, thereby enabling collection of the two sorted larval populations from the device. We demonstrated that the target larvae could be collected from a mixed worm population by cascading these devices. Our approach is based on only passive hydrodynamics filter structures, resulting in a user-friendly and reusable tool. In addition, we employed the equivalent of a standard bleaching procedure that is practiced in standard worm culture on agar plates for embryo harvesting on our chip, and we demonstrated rapid egg extraction and subsequent harvesting of a synchronized L1 larvae population.
Introduction
Caenorhabditis elegans is a commonly used multicellular model organism, the use of which is motivated by its short life cycle, hermaphrodite behavior, close human homologue genome match, transparency and easy culturing conditions with no ethical limitations.1–5 These nematodes have been widely studied as neurodegenerative disease models for toxicity testing of compounds and in drug discovery, because worm assays can provide valuable information in a rather short time compared to rodents.6–9 Typically, C. elegans pass through four larval stages (L1–L4) and reach adult stage in 2 to 3 days.10 Subsequently, adult nematodes lay embryos – which hatch in 10–12 hours – and the developmental cycle reinitiates with the development of larvae progeny. Frequently, age-synchronized populations at a designated larval stage are required to initiate an experimental sequence with a homogeneous set of worms. Any lagging or leading worms in the experimental set could potentially cause outliers in the resultant data. Synchronization methods to obtain age-synchronized worm populations to overcome this problem have been proposed, but these are often laborious and require the operator's manual work.11 Such methods consist of the operator's regular involvement by transporting a few adult worms in between Petri dishes to isolate and culture released progenies. Thus, there has been an increasing interest in the development of more automated technologies and protocols to resolve many of the tedious steps in manual manipulation.
COPAS Biosort is a commercially available automated sorting system that provides high-throughput sorting based on recognition of fluorescent protein expression patterns and other optical signatures.12,13 While this system can also be applied to C. elegans for age-synchronization of mixed worm populations at moderate accuracy, it is still an expensive and bulky solution. Lab-on-a-chip (LoC) devices have emerged as powerful bioanalytical assisting tools for handling bio-organisms and are renowned for their potentially low-cost fabrication protocols.14–16 Several LoC platforms have been reported to enable tackling many of the challenges that were encountered during standard handling, manipulation and culture protocols of C. elegans.17–19 While many of the reported microfluidic platforms allow automation in biological assays in terms of phenotyping, imaging and screening of C. elegans,20,21 dedicated devices for age-synchronization and sorting applications have been developed too.22–24 By means of electrotaxis, capitalizing on the nematode movement towards the cathodic side of an electrical field, and PDMS microfluidics, some researchers accomplished sorting of C. elegans larval stages. Some researchers employed age-based sorting using the electrotactic response of the nematode with a selectivity of 90%.25 While this method was automated and simple to use, it was working at low throughput. Another study displayed the size-dependent sorting application within a few minutes by taking advantage of the worms' electrotactic skills.26 PDMS–agarose hybrid platforms that allowed application of an electrical field were also illustrated for larvae sorting and for isolation of abnormally developing mutants.27 Such a platform leveraged the angular movement of nematodes in combination with the natural orientation of worms under electrotactic stimuli and isolated multiple larval stages. PDMS microchannels were also utilized as a feature in sorting applications based on electrotaxis.28 However, long-time application of an electrical field to the nematode might have adverse effects;25,26 therefore, alternative non-electrical approaches have been demonstrated for sorting applications. A continuous-flow-based microfluidic platform for sorting of C. elegans has been reported too.29 This microfluidic approach, based on optical fiber detection and laminar flow switching, allowed sorting of fluorescence-labeled and wild-type worms at rather low-throughput. Another automated motility-based high-throughput chip was reported.30 While this device was utilized to isolate mutants and can find potential use in genetic studies, it was also working at low-throughput and limited to its application range. A microfluidic device for high-speed sorting and immobilization of C. elegans was further modified to dispense screened worms to culture chambers for high-resolution time-lapse imaging.31 Integrated PDMS pressure valves have also been reported as impeccable add-on features for size-dependent sorting of C. elegans.32 In combination with pressure valves, researchers revealed a diode-like microfluidic chip for passive filtering of a mixed nematode population, ultimately obtaining a size-dependent isolation of larval stages in separate sorting chambers.33 These microfluidic chips have proven to be highly valuable for sorting applications, but their fabrication was complex and they did not allow egg harvesting directly on-chip. Another microfluidic platform reported early C. elegans larvae sorting via their encapsulation in droplets of a thermosensitive hydrogel.34 Size-based separation using an array of geometrically optimized pillars,35 maze-like designs36 that acted as sieves, or trapezoidal channels integrated in microfluidic spiral chips37 were also demonstrated for sorting. The versatile set of introduced designs allowed accurate sorting of larval stages, but challenges in fabrication, ease of operation, reusability, application range and throughput of these devices might hinder employment by non-experienced users in the longer run.
In this work, we present a microfluidic chip for rapid and high-throughput sorting of the nematode C. elegans. We defined an experimental protocol with reusable microfluidic chips for size-dependent sorting of various combinations of mixed larval populations. Our device is a hand-held tool that requires no external pressure control, electrical field, complicated tubing, or use of a syringe pump. Resembling a common syringe-based solution filtering approach, our microfluidic chip can be attached to the tip of a standard 1 mL syringe and several hundreds of mixed larvae can be sorted in a matter of minutes. As a further enabling feature of our microfluidic chip, we demonstrated the usage of this device in the application of egg harvesting as obtained from a standard bleaching protocol. We demonstrated that the aforementioned applications can be employed with a minimum of external components, avoiding bulky and expensive experimentation.
Materials and methods
Materials and chemicals
4 inch 550 μm thick Si wafers were obtained from the Center of Micro- and Nanotechnology of EPFL (Lausanne, Switzerland). PDMS Sylgard 184 was obtained from Dow Corning (Wiesbaden, Germany). MicroChem SU8-3050 1 L negative photoresist was acquired from Micro Resist Technology GmbH (Berlin, Germany). 1 mL borosilicate H-TLL-PE syringes were purchased from Innovative Labor Systeme (Stutzerbach, Germany). A Microline ethyl vinyl acetate tube with 0.51 mm inner and 1.52 mm outer diameters was obtained from Fisher Scientific (Wohlen, Switzerland). Trimethylchlorosilane (TMCS), sodium hydroxide and sodium hypochlorite solution 10–15% were acquired from Sigma-Aldrich (Buchs, Switzerland). L-Broth bacterial culture medium was obtained by adding 10 g of Bacto-tryptone, 5 g of Bacto-yeast, and 5 g of NaCl in 1 L of H2O. S-basal medium was prepared by adding 5.85 g of NaCl, 1 g of K2HPO4, 6 g of KH2PO4, and 1 mL of cholesterol (5 mg mL−1 in ethanol) in 1 L of H2O. S-medium was obtained by adding 0.5 mL of 1 M potassium citrate (pH 6), 0.5 mL of trace metal solution, 0.15 mL of 1 M CaCl2, and 0.15 mL of 1 M MgSO4 in 50 mL of S-basal medium. S-basal, L-Broth and S-medium were sterilized by autoclaving. The bleach solution was created by merging 0.33 mL of 4 M sodium hydroxide, 3.66 mL of DI water and 1 mL of 7–10% sodium hypochlorite solution.
Worm and bacteria preparation
A single colony of Escherichia coli strain OP50 was used from a streak plate and injected into L-Broth medium. The injected cultures were shaken at 37 °C overnight in an incubator-shaker. Nematode growth medium (NGM) plates were then seeded with this E. coli OP50 food source for C. elegans culturing at room temperature (22 °C) and at 16 °C. A synchronized population of around 1000–2000 L1 worms were distributed on a NGM plate after adjusting the density of the worms by counting the number of worms in a 2 μL suspension on a glass slide. When the population reached the desired larval stage, the NGM plate was washed with S-medium and the worms were suspended in an Eppendorf tube. Additionally, a standard bleaching procedure was employed on a gravid adult plate and the resultant embryo suspension was collected in an Eppendorf tube with filtered S-medium. The thus obtained embryo suspension hatched into L1 larvae in 12 hours, which were used to be cultured on NGM plates for future experiments. N2 wild-type strain was used and was provided by the Caenorhabditis Genetics Center (University of Minnesota).
Microfluidic device fabrication
Three dedicated molds were used to fabricate each PDMS part of the microfluidic device. We employed standard soft lithography techniques for fabrication of the three SU8 molds. Conventional photolithography was used to deposit an 80 μm thick layer of SU8 on 4 inch wafers. After developing three different designs on three different 4 inch wafers, the SU8 molds were treated with TMCS in a vacuum chamber for 15 minutes to avoid adhesion of PDMS during molding. A base-to-curing agent ratio of 10
:
1 PDMS mixture was dispensed on the molds and degassed for 30 minutes in a vacuum chamber which was followed by a 2 hour curing step at 80 °C. The molds were removed from the oven, the PDMS parts were peeled off – both sides of the PDMS parts were protected with a parafilm layer – and 1.5 mm inlets and outlets were pierced using a biopsy punch. Prior to plasma bonding, the parafilm layers were removed and the filtering layer was bonded first on the transmission layer. This was followed by another plasma bonding step to attach the collection layer to the previously bonded PDMS parts. The bonded microfluidic device was kept on a hotplate at 80 °C for at least 6 hours to enhance the bonding and later, the chip was mounted on the experimental setup, ready to be used. A schematic illustration of the fabrication protocol can be found in Fig. S1.†
Experimental preparation
The microfluidic device's input was connected to a borosilicate syringe and the output to an Eppendorf tube via Microline ethyl vinyl acetate tubing. Additional borosilicate syringes and Eppendorf tubes were reserved for the continuation of the experiment. The microfluidic device was fixed on the motorized stage of a microscopy control system (Visitron, Puchheim, Germany). The illumination source was set at white light for bright-field microscopy and videos were recorded during experimentation. After an experiment, Eppendorf tubes that were used to collect worm populations from the input and the output of the microfluidic device were shortly centrifuged and the excess liquid was removed, allowing a dense amount of worms to be characterized for the data analysis. The pressure measurement of the medium flow inside the microfluidic chip was performed using pressure sensors (Qmix P – Pressure Measurement Module, Cetoni GmbH).
Diameter measurements with image analysis
Sample worms obtained from the NGM agar plates (for characterization before the sorting experiment) and centrifuged Eppendorf tubes (for characterization after performing the sorting) were dispensed on a glass slide mounted on the microscope stage to be analyzed for diameter measurement. After acquiring images of the worms through a 20× (0.4 NA) objective, the diameter values of the worms were measured using open-source ImageJ software. A line perpendicular to the worm's spline around its vulva was drawn and the length of this line was computed. Further data classification was employed to associate the larval stages of the worms corresponding to the measured diameter values.
Experimental
Characterization of the initial mixed larval populations for use in the sorting experiments
Most of the prior C. elegans developmental studies used either L1 or L4 larval stages38–40 and omitted data collection from other synchronized larval stages. Our approach consisted in sorting of two consecutive larval stages (i.e. L1 and L2, L2 and L3, L3 and L4, L4 and young adult (YA), respectively) to validate the sorting principle of our microfluidic device. A challenge in such an experimental protocol is the preparation of well-characterized mixed larvae populations. The development and onset of larval stages of C. elegans strongly depend on temperature conditions during culture, a faster development being reported for higher temperatures.41,42 Based on these prior studies, we adopted an experimental approach that allows collection and testing of each of the C. elegans larval stages at a designated time point for use in the sorting experiment. We first obtained embryos by employing a standard bleaching procedure on a synchronized adult worm population that was cultured on a NGM agar plate.43,44 Following the hatching of such-harvested embryos, a synchronized L1 population was obtained (see “Materials and methods”). We distributed a certain amount of L1 worms on a fresh NGM plate and the exact time and temperature of culture was adjusted to obtain a synchronized population at a certain larval stage for use at a fixed experimental starting time X (see Table 1). We used an incubator to stabilize the temperature either at 16 °C or adopted room temperature (22 °C) culture conditions. The different larval stages can be distinguished from the average worm diameter data Davg as adapted from a prior study.32 The diameter measurements prior to and after each sorting experiment demonstrated that our synchronization approach was highly accurate (see Fig. S2–S6†).
Table 1 Experimental method to obtain L1, L2, L3, L4 and young adult (YA) C. elegans at a designated time point (hour X) from an initially characterized L1 larval population. For example, to have a population of synchronized YA, a synchronized L1 population needs to be cultured during 52 hours at 22 °C. The different larval stages can be distinguished from the average worm diameter data Davg
Targeted synchronized population at experimental starting time X |
YA |
L4 |
L3 |
L2 |
L1 |
L1 seeding time and culture temperature |
(X-52) hours (22 °C) |
(X-28) hours (22 °C) |
(X-20.5) hours (22 °C) |
(X-19) hours (16 °C) |
— |
D
avg [μm] |
47.9 |
29.5 |
22.1 |
17.0 |
11.7 |
Design of the microfluidic device
The main goal in the design and fabrication of the microfluidic sorting device was to create a user-friendly and simple device that could reach out to a broad user community. The main working principle of the sorting chip is based on allowing high-throughput and rapid sorting between multiple larval stages via passive hydrodynamic filter structures. In addition, the proposed device can be further exploited for egg extraction without any need of external hardware components. The microfluidic device is realized by combining three different PDMS parts that are labeled as the filtering, transmission and collection layers (Fig. 1a). While the filtering layer is utilized for collecting a worm population and sorting out the target worm population by means of passive hydrodynamics, the transmission and collection layers are used for the transfer and collection of the target worm population, respectively. Our device, which resembles a commercial syringe filter tip, can find potential use as a hand-held worm synchronization tool. The chip has a typical size of 36 × 36 × 9 mm; it can be held in an operator's hand while only requiring a borosilicate syringe and a desired length of tubing as additional items (Fig. 1b).
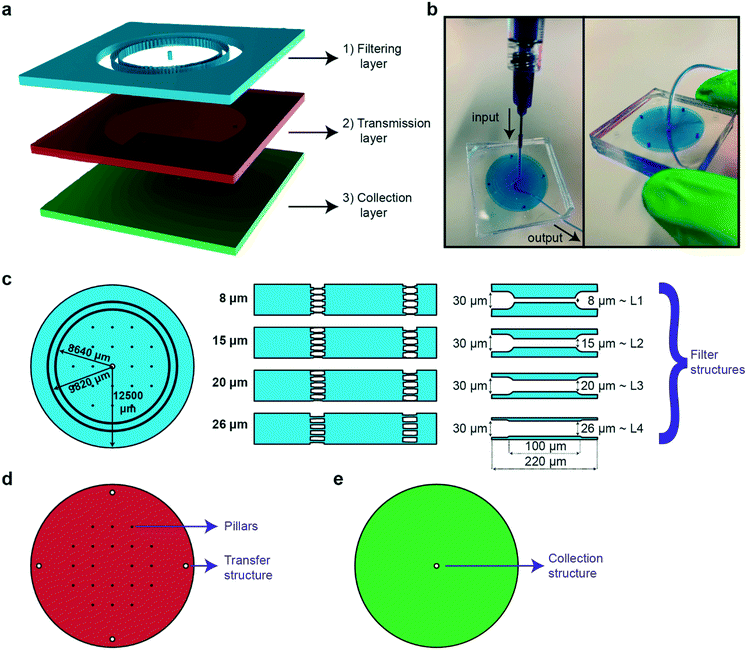 |
| Fig. 1 Details of the adjustable PDMS filter structures for size-dependent sorting and on-chip egg extraction of the nematode C. elegans. (a) Schematic illustration of the design. A worm population is sorted in the filtering layer, transferred via the transmission layer, and finally collected at the collection layer. (b) Photograph of the device filled with liquid dye solution. (c) Details of the filtering layer. Two circular filtering layers with a radius of 8640 and 9820 μm, respectively, are placed in series to ensure accurate sorting of the worm population. A hole is punched in the center for sample injection. The width of the filter structures on a circle are adjusted for the sorting of the target population, i.e. 8, 15, 20 and 26 μm filter widths and 80 μm height for the sorting of L1, L2, L3 and L4 target populations, respectively. (d) Details of the transmission layer. Pillars are used to avoid collapse of the PDMS parts during plasma bonding. Four hole transfer structures allow the transfer of a worm population down to the collection layer. (e) Details of the collection layer, which is simply a single hole collection structure, via which the sorted population is fetched. PDMS parts of the transmission and collection layers were demolded from 80 μm SU8-coated Si wafers. | |
The PDMS device has an outer radius of 12.5 mm and is designed to mimic the layout of a standard syringe filter (Fig. 1c). The filtering layer contains a primary and a secondary ring of sorting filters with a radius of 8640 and 9820 μm, respectively. The outer filter set acts as a fail-safe device in the event of PDMS-to-PDMS bonding issues – which might result in a local collapse of a filter set – or an accidental transfer of an undesired worm during an over inflation of filter structures; the inner filter ring is integrated as a second insurance to avoid any failure of the sorting principle. Integration of an additional filter set and pillars solved the aforementioned issues. The sorting of worm populations by means of passive hydrodynamics is supported by the integration of constriction filter structures in the filtering layer. The feature size of these filters can be tuned for the selective passage of the larval stage of interest.40 In fact, we constructed four different versions of the filtering layer with 8, 15, 20 and 26 μm wide filter structures that serve sorting of L1, L2, L3 and L4 larval stages, respectively. We observed that a filter width that is narrower than the targeted worms' diameter by 2–4 μm can accurately sort the population by compensating for minor PDMS inflations. Moreover, the natural elastic behavior of the nematodes facilitates crawling through filter structures that are narrower than their diameter. In parallel, such tight filters block the nematodes at older life stages. Once a worm population is sorted, it is transferred to the output of the device via the transmission layer (Fig. 1d). The 4 transfer structures are punched in the PDMS part, while the pillars are constructed to prevent collapse during both PDMS–PDMS plasma bonding and operation of the device. The collection layer contains a hole collection structure, which is punched in the PDMS part for extraction of the worm population (Fig. 1e). The chosen vertical buildup of the chip mimics a classical syringe porous membrane filter that is well known to the user. Additional feature sizes of these three layers can be found in Fig. S7.†
Sorting protocol
We optimized a standard protocol for repeatable and rapid sorting of various combinations of worm populations (Fig. 2). The microfluidic device was initially degassed by injecting 1 mL of filtered S-medium solution loaded in a 1 mL borosilicate syringe while the output of the device was clamped (Fig. 2a). We left the device in this configuration for a minimum of 6 hours or, in most cases, overnight. Such a step ensures a reliable sorting protocol as bubbles might hinder the sorting operation of the device. Prior to an experiment, the clamp was released and the excess S-medium was flushed from the output of the microfluidic device. Meanwhile, we designed the target sorting experiment by choosing two consecutive larval stages of interest. Population densities were adjusted to obtain 200–400 worms from each population by counting the worm density in 2 μL droplets from Eppendorf reservoirs, which were obtained by washing NGM agar plates with 1 mL of S-medium solution. After combining the worm populations and adjusting to a total volume of 1 mL, the suspension was loaded in a separate 1 mL borosilicate syringe. While we abided by a total injection volume of 1 mL to standardize our experimental approach, larger medium volumes can be injected too. The worm syringe was plugged instead of the S-medium syringe and the sorting experiment was initiated by employing a hand-held user-operated tool (Fig. 2b). Our experimental observations showed that the worm suspension injection could be done in a range of 30 to 60 seconds, without over-pressurizing the device, which might cause detachment of PDMS layers. Of note, other types of syringes can also be used for the fluidic protocol, while keeping in mind the average sorting flow rate of 17 to 33 μL s−1 (corresponding to 30 to 60 seconds of medium injection with a 1 mL syringe). We obtained pressure values of 0.9 and 1.3 bar for flow rates of 17 and 33 μL s−1, respectively. Our experimental observations revealed that a pressure above 1.3 bar exerted on the device can yield inaccurate sorting or even detachment of PDMS parts. The washed-off and sorted population was collected in an Eppendorf tube located at the output of the device. Once the syringe was emptied, it was replaced by another 1 mL borosilicate syringe that was filled with filtered S-medium solution (Fig. 2c). The main reason for such an approach was to remove any possible worm population remaining in the external tubing. The syringe was, once again, emptied in a time interval of 30 to 60 seconds. In order to collect the worm population residing in the filtering layer, the operation principle of the device was reverted (Fig. 2d). The input of the device was linked to an Eppendorf reservoir while connecting a 1 mL borosilicate syringe fully filled with S-medium solution at the output of the device. Another S-medium washing step was performed, in the opposite direction of the initial sorting part within the same sorting time as before, and the unsorted worm population was collected at the input. The bi-directional flow working principle of the microfluidic device enables rapid sorting and collection of worm populations with this experimental protocol. In a matter of 2 to 3 minutes, the entire sorting operation was performed. We note that we used the same microfluidic devices up to 3 to 5 times without a need of a new device fabrication. We fabricated new devices for additional sorting experiments to ensure reliable measurement results, but these experiments could have been also performed with the priorly fabricated microfluidic chips. We finally highlight the utility and user-friendliness of our device design for multiple sorting experiments and different larval stage synchronization applications.
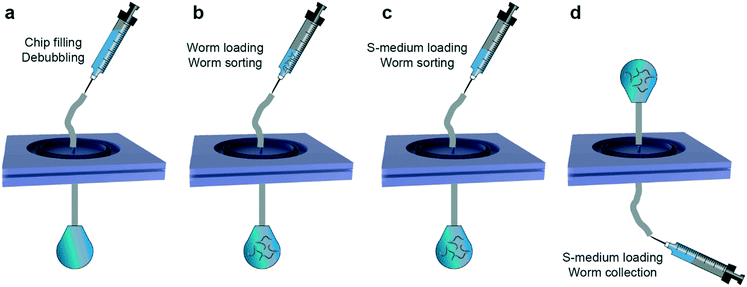 |
| Fig. 2 Details of the experimental protocol used during the sorting procedure. (a) In a first step, the PDMS device is degassed using over-pressure by injecting 1 mL of filtered S-medium solution while having the output of the device clamped. After an overnight degassing step, the clamp is released and the device is ready to be used. (b) A mixed worm population loaded in a 1 mL borosilicate syringe is injected in the device. (c) 1 mL of filtered S-medium solution is introduced to remove any remaining worm population in the tubing. The sorted population is collected at the output of the device. (d) 1 mL of filtered S-medium solution is injected from the output of the device to retain the worm population residing in the filtering layer. | |
Results and discussion
Results of the sorting experiments
We recorded real-time videos to characterize the sorting principle. We analyzed all the experiments from the recorded videos and we illustrated a series of sorting examples by extracting image frames from the video sets in Fig. 3. We characterized the travelling time of randomly picked target worms across the two filter sets to get the average sorting time per single worm. We initially tested the sorting of L1 populations out of L1 and L2 worm populations (Fig. 3a). In this particular example, we observed that an L1 worm successfully traveled across the two filter sets that contained filter structures with a width of 8 μm in 2.3 seconds. At this flow rate, larger worms – L2 worms in this specific experiment – were either blocked in the first or second filter sets. In a similar approach, we analyzed the sorting behavior of L2, L3, L4 and L1 worm populations out of L2 and L3, L3 and L4, L4 and YA, and L1 and YA worm populations, respectively (Fig. 3b–e). The widths of the filter structures were chosen as 15, 20, 26 and 8 μm with respect to the aforementioned experimental sequences. Our experimental observations revealed that if the residing population in the filtering layer was not large in body size, sorting can be realized very rapidly. In particular, we noticed that L2 and L3 worm populations can be sorted in a matter of 2–3 seconds per worm (Fig. 3b and c), mostly due to the large number of available filter structures a worm can pass through. On the other hand, when a YA worm was resting in the filtering layer, most of its body was covering the filter structures of the first filter set (Fig. 3d and e). As such, the sorting time was slightly increased to be in the range of 3 to 7 seconds, while the variations in sorting time did not have any correlation with the accuracy of the sorting principle.
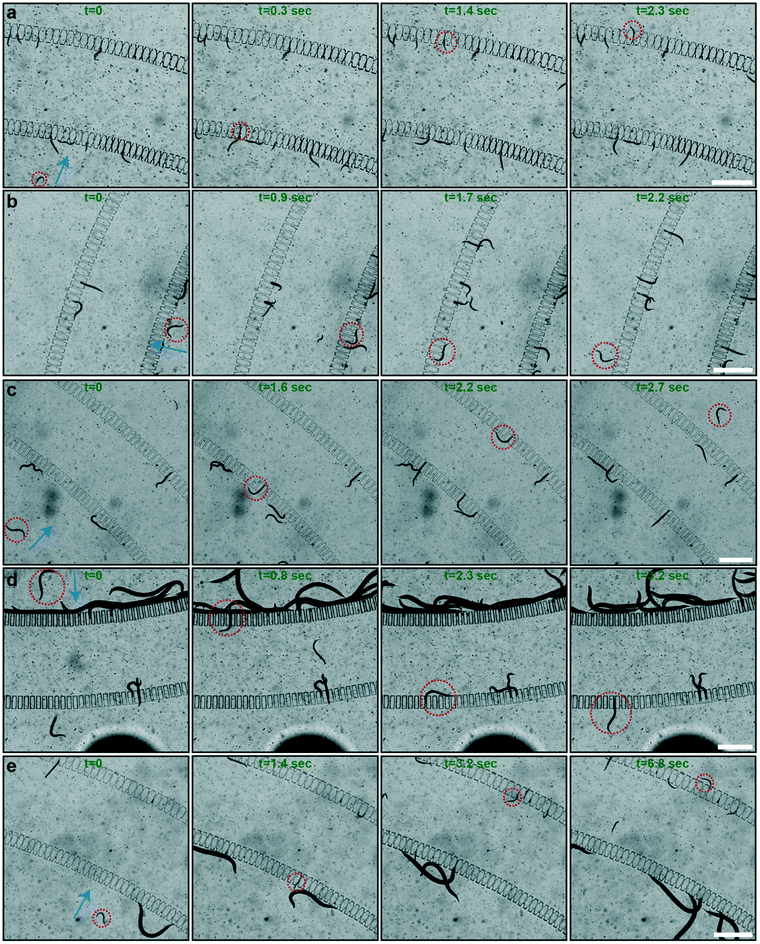 |
| Fig. 3 Real-time images illustrating the sorting procedure; the target worms of the sorting are highlighted by red circles. The flow directions during sorting are indicated by blue arrows. (a) The real-time sorting of an L1 worm in 2.3 seconds in a worm population of L1 and L2 worms. (b) The real-time sorting of an L2 worm in 2.2 seconds from a population of L2 and L3 worms. (c) The real-time sorting of an L3 worm in 2.7 seconds from a worm population of L3 and L4 worms. (d) The real-time sorting of an L4 worm in 3.2 seconds from a worm population of L4 and young adult (YA) worms. (e) The real-time sorting of an L1 worm in 6.8 seconds from a worm population of L1 and YA worms. Scale bars: 500 μm. | |
We displayed the different diameter values measured after the sorting experiments in Fig. S8.† We observed that for L1–L2, L4–YA and L1–YA worm sorting experiments, the diameter values before and after sorting experiments lie around the nominal onset diameter values of each larval stage (Fig. S8a, d and e†). This means that while the initial calibration of the worm plates was indeed accurate, after merging the two populations and sorting them with the microfluidic device, the sorted populations were again having the expected diameter and larval stage. However, some outliers were noted for L2–L3 and L3–L4 worm sorting experiments, such as worms that exhibit smaller diameter values at the input and larger diameter values at the output chamber of the microfluidic chip, respectively (Fig. S8b and c†). This was probably due to (i) local PDMS inflation during the sorting process or (ii) the crawling behavior of the nematodes. Of note, a dense worm population around the L1 onset diameter is collected at the output after L1–YA sorting experiments (Fig. S8e†). The latter also exemplifies our technique as an alternative method to rapidly isolate early L1 progenies from a NGM agar plate that they usually populate along with adult nematodes. While our methodology of using diameter-based statistics summarizes the working principle of the sorting protocol, a quantitative assessment of the sorting experiments requires further data analysis.
Population-based statistics of the sorted nematodes
Based on the worm diameter measurements, we can classify the developmental stages of randomly picked worms during their life cycle. To this purpose, we associated each larval stage with a diameter measurement range Drange around the average diameter value associated with that larval stage (Davg listed in Table 1), as shown in Fig. 4a. In particular, we checked the diameter D of the worm and we assigned it to a larval stage if it was in the range, Davg − Drange < D < Davg + Drange. We defined Drange to be 2.8, 2.5, 2.6, 4.8 and 6 μm for L1, L2, L3, L4 and YA worms, respectively. If a worm was not exactly in one of those intervals, but it was in a developmental stage situated in between the maximum diameter of the earlier stage and the minimum diameter of the later stage, as happened for the later larval stages, it was chosen as being in the ‘transition region’ in between these two larval stages (defining the L4–YA and YA to adult (YA–A) transition stages). We demonstrated the population-based sorting results of all our experiments in Fig. 4b–f, where we list both the distribution of larval stages within the initial mixed populations and the distribution of the sorted populations collected from both the input and the output from our filter chip. As already mentioned in the experimental section, we have prepared the different larvae populations by ‘synchronized’ culture on NGM agar plates. We underline the accuracy of the latter cultures, as we obtained 96%, 95%, 83% and 91% purity of the aimed L1, L2, L3 and L4 larval stages on their NGM plate, respectively (Fig. S9†). The local E. coli distribution, the population density, and the distribution of worms on an NGM plate evidently can cause deviations from the ideal target worm population of 100%, leading to worms with a slightly slower or faster development. Also our method allowed worms in YA and YA–A transition to be collected from an agar plate, with a population of 40% and 58%, respectively. In the preparation of a sorting experiment, we combined an approximately identical amount of worms from two consecutive larvae populations, and we loaded the mixed worm suspension in our device, the distribution of which is indicated by the left-hand side bars in Fig. 4b–f. L1–L2 worm sorting experiments revealed a 94% accuracy in the sorted L1 worms at the output of the device, while the input of the device had only 10% L1 stage larvae and 90% of combined L2 and L3 larvae (Fig. 4b). While the older stage larvae sorting (L3 larvae and a few L4 larvae) at the input in the L2–L3 sorting experiments demonstrated a sorting accuracy of 97%, the same operation allowed 27% of L3 larvae being transferred to the output (Fig. 4c). A similar trend was also noted for L3–L4 sorting experiments by permitting only 8% L4 larvae to be collected at the output (Fig. 4d). In this case, the input side of the filter device had only 10% L3 larvae after finalization of the sorting experiments. The sorting results of the L4–YA worms showed that the output of the device can sort young larvae (L3 and L4) at 100%, whereas the input side displayed a sorting characteristic of a combined 96% for YA and YA–A worms (Fig. 4e). The same type of behavior was also observed in the L1–YA sorting experiments (Fig. 4f). We note that, in this case, 5% of L2 larvae were also collected at the output of the sorting device, while they were not spotted initially in the collected samples from the agar culture plates. Summarizing, we demonstrated that the filter device can be conceived for accurate size-dependent sorting of all consecutive larval stages, an exception being the sorting of L2–L3 larvae at the output where 73% of L2 stage larvae could be collected (Fig. 4c).
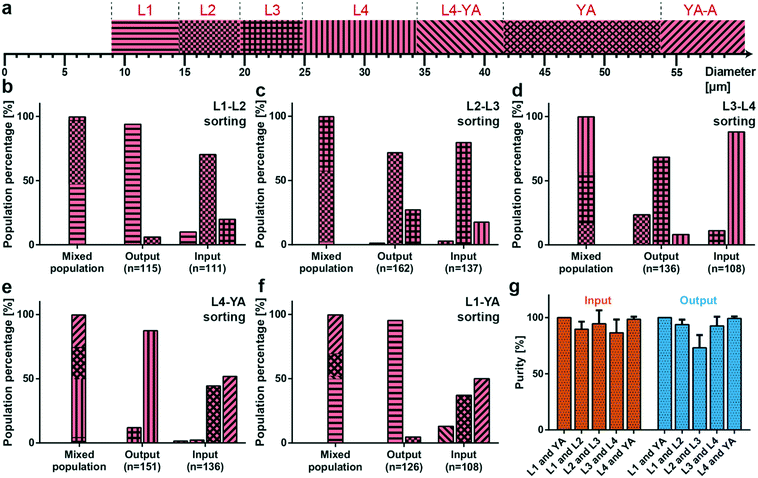 |
| Fig. 4 Results from the sorting experiments. (a) Assignment of the larval stage with respect to the diameter. The mean diameter Davg of each stage is taken from Table 1 and a larva/worm is classified in a stage (denoted as L1, L2, L3, L4, and YA) when its diameter D is in the range Davg − Drange < D < Davg + Drange, while Drange is 2.8, 2.5, 2.6, 4.8 and 6 μm for L1, L2, L3, L4 and YA worms, respectively. Also the intermediate stages, L4–YA and YA to adult stage (YA–A), are defined. (b–f) Distribution of worm populations, as determined from the worm diameter, before sorting (“Mixed population” results) and after all sorting experiments (“Output and Input” results) for mixed worm populations of (b) L1 and L2, (c) L2 and L3, (d) L3 and L4, (e) L4 and YA, and (f) L1 and YA, respectively. The total number of worms measured for a particular condition, the number n, is noted below the bar plots. (g) Sorting purity of the initially mixed populations (L1 and YA, L1 and L2, L2 and L3, L3 and L4, and L4 and YA) at the input and the output of the microfluidic chip. Ideally, the higher stage larvae are all collected at the input (100% purity), while the lower stage larvae are all obtained at the output (100% purity). Data are expressed as mean ± SD. Measurements are based on 6 experiments for each sorting experiment. | |
Purity of the sorting
A more subtle sorting characteristic was required to prove the efficacy of the microfluidic device. Therefore, we have chosen a ‘purity’ parameter to evaluate the device operation protocol.32,35,37 Purity can be defined as the number of target worms divided by the number of all the worms at a target location (i.e. the output or input of the chip). As an example, if there were a1% L1, a2% L2, and a3% L3 worms at the output and b1% L2, b2% L3, and b3% L4 worms at the input of the device for an L2–L3 sorting experiment, purity could be calculated as a2% and b2% for the output and the input, respectively. For our experiments, we defined purity, however, as 100% − a3% at the output (100% − b1% at the input) of the microfluidic device to take into account younger larvae that were eventually present in the mixed population at the output and older larvae at the input, respectively. We additionally displayed the ‘purity precision’ of the sorting – by choosing a2% and b2% for the output and the input, respectively – in Fig. S10.† We looked at the individual experiments, calculated the purity of each one and then combined the data together (Fig. 4g). We obtained a purity of 100%, 90%, 95%, 87% and 99% at the input and 100%, 94%, 73%, 93% and 99% at the output of the device for L1–YA, L1–L2, L2–L3, L3–L4 and L4–YA sorting experiments, respectively. Interestingly, a set of slightly higher purity values was found at the input compared to the output. This is understandable, as the older worm population can either reside at the input, upstream of the filter structures, or accidentally be transferred to the output. This behavior might lead to a drop in purity for the larval population that is expected to be collected at the output, whereas the input purity will remain unaffected. The high-purity values obtained at the input of the device indicate that the input side of the microfluidic device is the one that can be optimally tuned for sorting applications. We noticed a significant drop of purity at the output of the L2–L3 sorting device, as already noted in the results priorly obtained through population-based statistics. However, the remaining purity values computed here indicate that the sorting purity of the proposed device is competitive compared to the previously proposed sorting results. Moreover, we can perform high-purity sorting while accomplishing such procedures in a significantly shorter period and in a less complicated manner than before. We characterized the sorting operation of the device at higher throughput by combining 800 worms from each L4 and YA worm population, thereby providing approximately 4 times more worms than the initial sorting experiments (Fig. S11†). Our results revealed that higher throughput sorting was enabled with our device too, while establishing purity of 93% and 99% at the input and at the output of the device, respectively.
Extraction of target larvae from a mixed worm population
After the initial characterization of the sorting principle of the proposed microfluidic devices, we focused on extraction of a target worm population out of a mix of all worm populations, which is typically observed on an NGM agar plate. As such, we cascaded two microfluidic chips with different filter structures via tubing and the metallic part of a syringe tip as a connector (Fig. 5). For example, an L3 population can be obtained by using first a microfluidic chip with 20 μm filter structures and collecting the worm population at the output (containing L3, L2 and L1 worms). In a second step, the thus filtered population can be loaded in a microfluidic chip with 15 μm filter structures (targeting L2 and L1 worms at the output) and one could collect the remaining L3 worm population residing at the input. In our study, we utilized microfluidic chips with double rings of 26 and 20 μm and 20 and 15 μm filter structures for extracting L4 and L3 larvae, respectively. We defined a new fluidic protocol to extract these worm populations (Fig. 5a–e). First, we filled the chips with 1 mL of S-medium using a 1 mL borosilicate syringe, while the output was clamped to remove any air bubbles inside the microfluidic devices by gas diffusion through PDMS (Fig. 5a). Thereafter, a mixed worm population (containing 200 worms from each of the L1, L2, L3, L4, and YA worm development stages) was injected in the device in a time interval of 30 to 60 seconds (Fig. 5b). This was followed by injecting 1 mL of S-medium to flush worms remaining in the tubing within a similar time interval (Fig. 5c). Then, we clamped all the tubing and disassembled the device (Fig. 5d). This step was crucial to ensure a faultless sealing of the device against air bubbles. 1 mL of S-medium was injected from the output side of each microfluidic device to characterize the worm distributions at the “Input”, “Output” and “Center” inlets of both devices (Fig. 5e). The target worm population was each time extracted from the center inlet. We first characterized the worm diameter distribution at all stages of the experiment (Fig. S12†). The worm distribution on agar plates before sorting was once again highly accurate. For both filter chips in the cascaded configuration, we noticed a variability at the input and the output. However, the center inlet of the 26–20 μm and 20–15 μm filter chips displayed highly selective sorting of the targeted L4 and L3 worm populations, respectively. We associated these data to larval stages to obtain population purity percentage statistics (Fig. 5f and g). Our results revealed a population-averaged percentage of 67% for L4 larvae (Fig. 5f) and 65% for L3 larvae (Fig. 5g) collected at the center inlet with the 26–20 μm and 20–15 μm filter chips, respectively. While we previously illustrated that 83% L3 larvae and 91% L4 larvae can be obtained through a standard NGM plate-based method, this alternative microfluidic technique provides rapid larvae synchronization within a period of 4–5 minutes only. Therefore, lengthy worm culture and worm population tracking can be eliminated with our device with only a slight drop in the population purity percentage of the target worms. Throughout this experimental procedure, we lost around 3% of the initial worm population in between the two filter rings of both microfluidic devices, which was deduced by examining the devices after the experiments with a 4× (0.1 NA) objective.
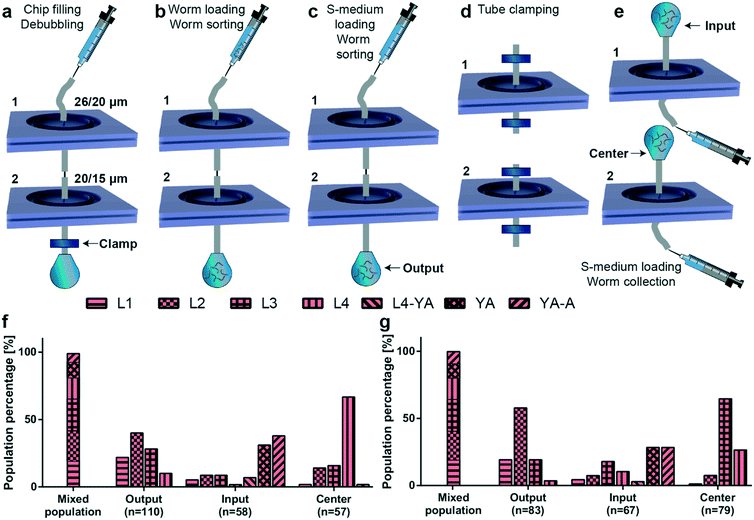 |
| Fig. 5 Details of the experimental protocol and results of the cascade sorting experiments. (a) In a first step, the cascaded PDMS devices are degassed by applying over-pressure by injecting 1 mL of filtered S-medium solution using a 1 mL borosilicate syringe, while having the output of the device clamped. (b) A mixed worm population loaded in a 1 mL borosilicate syringe is injected in the device after releasing the clamp. (c) 1 mL of filtered S-medium solution is introduced to remove any remaining worm population in the tubing. (d) All tube connections are clamped and the cascade is disassembled to collect target worm populations. (e) 1 mL of filtered S-medium solution is injected from the output of each PDMS device to retain the worm population residing in the filtering layers. (f and g) Distribution of worm populations, as determined from the worm diameter, before sorting (“Mixed population” results) and after all sorting experiments (“Output, Input and Center” results) for mixed worm populations with the cascaded PDMS devices containing top-to-bottom (f) 26 and 20 μm and (g) 20 and 15 μm filter structures, respectively. The total number of worms measured for a particular condition, the number n, is noted below the bar plots. Measurements are based on 4 experiments for each sorting experiment. | |
On-chip egg extraction
We can also exploit our device to perform additional separation or sorting experiments. In particular, we demonstrated on-chip egg extraction from gravid adults (Fig. 6) by employing a similar protocol to that in the sorting experiments. After having the microfluidic device filled with S-medium solution and degassed, we loaded a synchronized adult worm population in the device (Fig. 6a). In parallel, a standard bleaching solution was prepared (see “Materials and methods”), loaded in a 1 mL borosilicate syringe and plugged in the input of the microfluidic device. The bleaching solution was injected in the device and occasionally, manual pull–push steps by the syringe were required to break adult body fragments utilizing filter structures (Fig. 6b). Once all the body fragments were dissolved (typically in 10–15 minutes) and only embryos were left in the filtering layer, a 1 mL borosilicate syringe containing filtered S-medium was connected at the input and the device was washed in approximately 30 seconds. The S-medium washing step was repeated at least 3 times to ensure removal of bleaching reagent. In the last S-medium washing step, the output of the device was clamped and it was left in this configuration overnight (Fig. 6c). The day after – or at least 12 hours later – the output was gently unclamped to avoid instant S-medium flush in the device due to overpressure. We noticed a population of synchronized L1 larvae, unhatched embryo clusters and debris in the filtering layer (Fig. 6d). 1 mL of S-medium was injected from the input to collect L1 population at the output. This operation was also performed from the output to retain the remaining debris and embryos from the input. After characterizing the obtained samples under a bright-field microscope (Fig. 6e), we noticed that the output sample contained 100% L1 larvae, while the sample at the input contained unhatched embryos or aggregates of embryos and debris. The microfluidic filter device served two purposes in this study: (i) allowing the collection of a synchronized L1 worm population and (ii) removal of any dirt or unhatched embryos created during the bleaching procedure. Additionally, by injecting 1 mL of S-medium from the output as soon as the bleaching process was finalized, the device can be tuned for egg collection from the input. Our method allows rapid egg extraction and L1 larvae synchronization without a need of any external hardware. We believe that this feature is highly valuable for the C. elegans research community, as our chip easily replaces all the required hardware needed for a standard bleaching procedure. We also tried another methodology in an attempt to exclude even the traditional bleaching step from the protocol (Fig. S13†). This method constituted of a manual and local PDMS deflection to extract eggs from mother nematodes.45 We noticed that the method works too but is subject to future optimization opportunities, as it can enable massive egg extraction and L1 larvae synchronization without a need of both external hardware and a bleaching protocol.
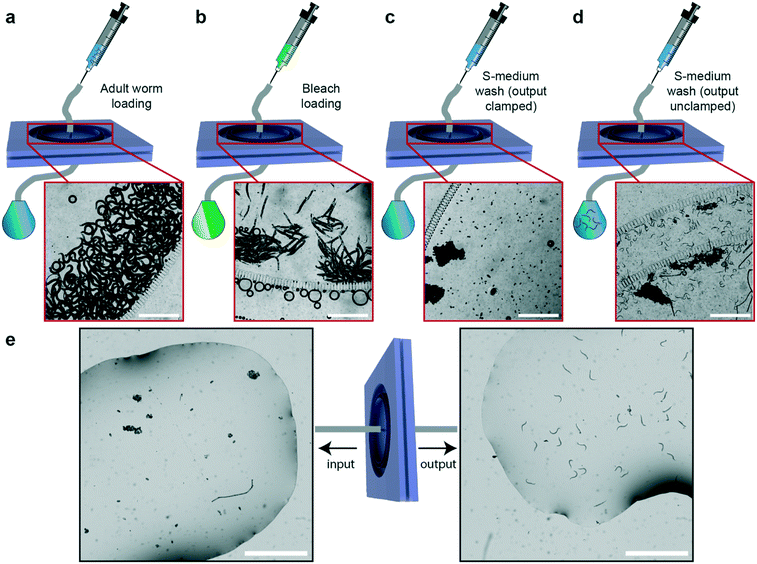 |
| Fig. 6 Schematic illustration and real-time images of the experimental protocol for on-chip egg extraction. (a) After chip filling and degassing steps, an adult worm population is loaded in the microfluidic device. (b) A standard bleaching solution is exposed to the adult worms present in the filtering layer. (c) Once the adult body fragments disappear, a fresh S-medium solution is injected to terminate the bleaching process. After three cycles of S-medium injection of 1 mL, for degassing of the chip, its output is clamped and S-medium solution is loaded from the input to leave embryos overnight while keeping the PDMS chip inflated. (d) After 12 hours, the clamp is released and the L1 population that hatched from the eggs is washed out of the microfluidic device. A similar procedure is also applied to the output of the device to retain unhatched embryo population. (e) Unhatched embryos and embryo aggregates are seen to be retained at the input of the device, while the output of the device produces a synchronized L1 population. Scale bars: 1000 μm. | |
Conclusions
We report a new microfluidic filter device for size-dependent sorting and rapid egg extraction of C. elegans. Worm assays require age-synchronized populations for longitudinal developmental and culture studies, and accurate age synchronization methods traditionally often require tedious manipulation methods. We tackled these issues by providing a hand-held tool that will be easy to operate by all C. elegans researchers. The proposed device consists of three plasma-activated and bonded PDMS parts, in which the adjustable filter structures permit selective sorting of several combinations of adjacent larval stages. We used 8, 15, 20 and 26 μm filter structures for target worm populations of L1, L2, L3 and L4 larvae, respectively. Our device accurately sorts any two consecutive developmental stages with a purity ranging from 73% to 100% at the output and from 87% to 100% at the input of the microfluidic chip. Our device showed the possibility of multiple reuse in the absence of major clogging issues with the filter structures. As the device was operating with a massive amount of worms, the exact numbers of larvae were not precisely calculated but rather approximated. We characterized the sample sizes by checking a few 2 μL droplets from the population reservoirs and we adjusted the worm populations used in the experiments accordingly. We tried to have almost equal amounts of worms, in the range of 200 to 400 worms, from two adjacent larval stages per experiment. The entire sorting operation took place in 2 to 3 minutes, displaying an average throughput of 160 to 240 worms per minute. We studied the sorting operation with moderate sample sizes to closely observe its functioning. However, the throughput of the device can be enhanced by increasing the sample size without a problem. We additionally illustrated an experimental approach to synchronize larvae populations at different stages on NGM agar plates prior to an experiment.
We illustrated two different double filter ring sorting devices of the proposed microfluidic chips for extraction of L3 and L4 worm populations out of a mixed population, respectively. We reported a worm population purity for these two stages of 65% and 67%, respectively, in approximately 4–5 minutes of operation. Eventually, different filter structures could be configured on a single filter chip too, thereby creating a more user-friendly configuration. In future, this type of approach could be further optimized with different filter combinations to refine further the sorting of all target population from a mixed population.
All size-dependent sorting experiments have been executed by exploiting wild-type nematodes and hence the parameters of the microfluidic design were adapted for such experimentation. However, it has been well known that dumpy animals with aberrant morphologies exist, as mutations can have abnormal body sizes.46 Our device will not work accurately with such nematodes, unless a dedicated filter design will have been performed. On the other hand, C. elegans mutants with reduced motility47 could still work fine with our microfluidic chip, although the device is prone to longer operation time due to the immobile nature of these mutants. Also one must keep in mind the average onset diameter values of mutant larvae while utilizing our microfluidic device for size-dependent sorting.
We exploited some of the microfluidic devices with a filter structure width of 8 μm for egg harvesting applications. Our technique allowed rapid extraction of C. elegans eggs without a need of any external hardware. By employing a standard bleaching procedure on-chip, we collected C. elegans embryos within 20–30 minutes. Such a method bypassed the requirement of expensive hardware, like centrifugation equipment, and all harvesting protocols could be implemented using a 1 mL borosilicate syringe, microfluidic chip, external tubing, Eppendorf reservoirs and a bleaching solution. Other syringe types with different volumes can also be mounted by simply adjusting the injection flow rate. 12 hours after embryo collection, we retained a synchronized population of L1 larvae at the output of the microfluidic device. In another effort, we used the mechanical deflection properties of PDMS to extract embryos from the mother nematodes. By exerting local PDMS deflection, we managed to mechanically compress some adult nematodes and we harvested their embryos. While this methodology eliminates usage of a toxic bleaching solution, further optimization, such as a uniform application of the pressure for PDMS deflection over the complete microfluidic chamber that hosts the mothers may be required to enable high-throughput egg extraction.
Overall, we illustrate here our novel platform for rapid larvae sorting and egg extraction of the nematode C. elegans at high throughput. Based on the purity results, highly selective larvae sorting has been performed and an alternative method for egg harvesting has been demonstrated. We believe that this microfluidic chip can reach out to a broad scientific community to tackle many labor-intensive C. elegans handling steps. The hand-held configuration of the device in particular provides massive simplification for applications, as it can be used just like a standard syringe filter. In the near future, with further design optimization, this device could find wide use in nematode synchronization and egg harvesting applications.
Author contributions
H. B. A. and F. A. designed the platform. H. B. A. designed the experiments. H. B. A. designed the chip and fabricated the wafers. H. B. A. and F. A. fabricated the microfluidic devices and performed the experiments. H. B. A. analyzed the data. H. B. A., F. A. and M. A. M. G. wrote the paper.
Conflicts of interest
The authors declare no conflict of interest.
Acknowledgements
Work in the MG laboratory was supported by the Ecole Polytechnique Fédérale de Lausanne and the EU Ideas Program (ERC-2012-AdG-320404). We thank the staff of the Center of Micro- and Nanotechnology of EPFL for assistance in microfabrication processes, Roger Krenger and Dr. Matteo Cornaglia for the fruitful discussions on C. elegans, and the Caenorhabditis Genetics Center, which is funded by the NIH Office of Research Infrastructure Programs (P40 OD010440), for providing worm strains.
References
- T. Kaletta and M. O. Hengartner, Nat. Rev. Drug Discovery, 2006, 5, 387–399 CrossRef CAS PubMed
.
- M. Markaki and N. Tavernarakis, Biotechnol. J., 2010, 5, 1261–1276 CrossRef CAS PubMed
.
- E. L. L. Sonnhammer and R. Durbin, Genomics, 1997, 46, 200–216 CrossRef CAS PubMed
.
- M. C. K. Leung, P. L. Williams, A. Benedetto, C. Au, K. J. Helmcke, M. Aschner and J. N. Meyer, Toxicol. Sci., 2008, 106, 5–28 CrossRef CAS PubMed
.
- S. Brenner, Genetics, 1974, 77, 71–94 CAS
.
- A. Desalermos, M. Muhammed, J. Glavis-Bloom and E. Mylonakis, Expert Opin. Drug Discovery, 2011, 6, 645–652 CrossRef CAS PubMed
.
- E. Braungart, M. Gerlach, P. Riederer, R. Baumeister and M. C. Hoener, Neurodegener. Dis., 2004, 1, 175–183 CrossRef CAS PubMed
.
- P. R. Hunt, J. Appl. Toxicol., 2017, 37, 50–59 CrossRef CAS PubMed
.
- C. Voisine, H. Varma, N. Walker, E. A. Bates, B. R. Stockwell and A. C. Hart, PLoS One, 2007, 2, e504 CrossRef
.
- V. Ambros, Curr. Opin. Genet. Dev., 2000, 10, 428–433 CrossRef CAS PubMed
.
- E. M. Jorgensen and S. E. Mango, Nat. Rev. Genet., 2002, 3, 356–369 CrossRef CAS PubMed
.
- R. Pulak, Methods Mol. Biol., 2006, 351, 275–286 Search PubMed
.
- M. Doitsidou, N. Flames, A. C. Lee, A. Boyanov and O. Hobert, Nat. Methods, 2008, 5, 869–872 CrossRef CAS
.
- V. Sivagnanam and M. A. M. Gijs, Chem. Rev., 2013, 113, 3214–3247 CrossRef CAS PubMed
.
- M. Muthaiyan Shanmugam and T. Subhra Santra, Molecules, 2016, 21, 1–16 CrossRef PubMed
.
- B. P. Gupta and P. Rezai, Micromachines, 2016, 7, 123 CrossRef PubMed
.
- M. F. Yanik, C. B. Rohde and C. Pardo-Martin, Annu. Rev. Biomed. Eng., 2011, 13, 185–217 CrossRef CAS PubMed
.
- S. E. Hulme, S. S. Shevkoplyas and A. Samuel, Nat. Methods, 2008, 5, 589–590 CrossRef CAS PubMed
.
- M. M. Crane, K. Chung, J. Stirman and H. Lu, Lab Chip, 2010, 10, 1509–1517 RSC
.
- L. P. O'Reilly, C. J. Luke, D. H. Perlmutter, G. A. Silverman and S. C. Pak, Adv. Drug Delivery Rev., 2014, 69–70, 247–253 CrossRef
.
- A. Ben-Yakar, N. Chronis and H. Lu, Curr. Opin. Neurobiol., 2009, 19, 561–567 CrossRef CAS PubMed
.
-
A. San-Miguel and H. Lu, Microfluidics as a tool for C. elegans research, WormBook, ed. The C. elegans Research Community, (September 24, 2013), DOI:10.1895/wormbook.1.162.1, http://www.wormbook.org
.
- N. A. Bakhtina and J. G. Korvink, RSC Adv., 2014, 4, 4691–4709 RSC
.
- K. Chung, M. M. Crane and H. Lu, Nat. Methods, 2008, 5, 637–643 CrossRef CAS PubMed
.
- P. Rezai, S. Salam, P. R. Selvaganapathy and B. P. Gupta, Lab Chip, 2012, 12, 1831–1840 RSC
.
- X. Manière, F. Lebois, I. Matic, B. Ladoux, J. M. Di Meglio and P. Hersen, PLoS One, 2011, 6, e16637 CrossRef PubMed
.
- X. Wang, R. Hu, A. Ge, L. Hu, S. Wang, X. Feng, W. Du and B. F. Liu, Lab Chip, 2015, 15, 2513–2521 RSC
.
- B. Han, D. Kim, U. H. Ko and J. H. Shin, Lab Chip, 2012, 12, 4128–4134 RSC
.
- Y. Yan, L. F. Ng, L. T. Ng, K. B. Choi, J. Gruber, A. A. Bettiol and N. V. Thakor, Lab Chip, 2014, 14, 4000–4006 RSC
.
- J. Yuan, J. Zhou, D. M. Raizen and H. H. Bau, Lab Chip, 2015, 15, 2790–2798 RSC
.
- C. B. Rohde, F. Zeng, R. Gonzalez-rubio, M. Angel and M. F. Yanik, Proc. Natl. Acad. Sci. U. S. A., 2007, 104, 13891–13895 CrossRef CAS PubMed
.
- L. Dong, M. Cornaglia, T. Lehnert and M. A. M. Gijs, Lab Chip, 2016, 16, 574–585 RSC
.
- L. Yang, T. Hong, Y. Zhang, J. G. S. Arriola, B. L. Nelms, R. Mu and D. Li, Biomed. Microdevices, 2017, 19, 1–11 CrossRef
.
- G. Aubry, M. Zhan and H. Lu, Lab Chip, 2015, 15, 1424–1431 RSC
.
- X. Ai, W. Zhuo, Q. Liang, P. T. McGrath and H. Lu, Lab Chip, 2014, 14, 1746–1752 RSC
.
- X. C. I. Solvas, F. M. Geier, A. M. Leroi, J. G. Bundy, J. B. Edel and A. J. DeMello, Chem. Commun., 2011, 47, 9801–9803 RSC
.
- S. Sofela, S. Sahloul, M. Rafeie, T. Kwon, J. Han, M. E. Warkiani and Y. A. Song, Lab Chip, 2018, 18, 679–687 RSC
.
- M. Cornaglia, T. Lehnert and M. A. M. Gijs, Lab Chip, 2017, 17, 3736–3759 RSC
.
- M. Cornaglia, G. Krishnamani, L. Mouchiroud, V. Sorrentino, T. Lehnert, J. Auwerx and M. A. M. Gijs, Mol. Neurodegener., 2016, 11, 1–13 CrossRef
.
- H. B. Atakan, M. Cornaglia, L. Mouchiroud, J. Auwerx and M. A. M. Gijs, Lab Chip, 2019, 19, 120–135 RSC
.
- L. Byerly, R. C. Cassada and R. L. Russell, Dev. Biol., 1976, 51, 23–33 CrossRef CAS PubMed
.
-
Z. F. Altun and D. H. Hall, Introduction, in WormAtlas, 2009, DOI:10.3908/wormatlas.1.1
.
-
H. B. Atakan, R. Xiang, M. Cornaglia, L. Mouchiroud, J. Auwerx and M. A. M. Gijs, in 20th International Conference on Solid-State Sensors, Actuators and Microsystems (TRANSDUCERS), 2019, pp. 2209–2212 Search PubMed
.
- H. B. Atakan, R. Xiang, M. Cornaglia, L. Mouchiroud, E. Katsyuba, J. Auwerx and M. A. M. Gijs, Sci. Rep., 2019, 9, 14340 CrossRef CAS PubMed
.
- L. Dong, R. Jankele, M. Cornaglia, T. Lehnert, P. Gönczy and M. A. M. Gijs, Adv. Sci., 2018, 5, 1700751 CrossRef PubMed
.
- C. Mörck and M. Pilon, BMC Dev. Biol., 2006, 6, 39 CrossRef
.
- J. F. Morley, H. R. Brignull, J. J. Weyers and R. I. Morimoto, Proc. Natl. Acad. Sci. U. S. A., 2002, 99, 10417–10422 CrossRef PubMed
.
Footnote |
† Electronic supplementary information (ESI) available. See DOI: 10.1039/c9lc00949c |
|
This journal is © The Royal Society of Chemistry 2020 |