DOI:
10.1039/D0GC01830A
(Paper)
Green Chem., 2020,
22, 6404-6414
Towards sustainable ethylene production with cyanobacterial artificial biofilms†
Received
29th May 2020
, Accepted 7th September 2020
First published on 7th September 2020
Abstract
Photosynthetic cyanobacteria hold a great potential for the direct conversion of solar energy and CO2 into ‘green’ ethylene. The present study aims to develop a thin-layer artificial biofilm technology for sustainable and long-term ethylene photoproduction, where recombinant Synechocystis sp. PCC 6803 cells holding ethylene forming enzyme (Efe) from Pseudomonas syringae are entrapped within the natural polymer matrix, thus forming the thin-layer biocatalytic structure. The production system was optimized by varying different parameters, such as radiance, inorganic carbon level, and periodicity of medium renewal. As a result, artificial films with entrapped cells of Synechocystis sp. PCC 6803 mutant produced ethylene for up to 38 days, yielding 822 mL m−2 ethylene at 1.54% light to ethylene conversion efficiency. These figures represent a 2-time enhancement in the duration of ethylene production, a 2.2-fold increase in the production yield, and a 3.5-fold improvement in the light to ethylene conversion efficiency as compared to the cell suspension. This study demonstrates that ethylene producing cyanobacteria entrapped in the polymeric matrix could truly act as photo-biocatalyst for the prolonged ethylene production by strongly limiting biomass accumulation and maintaining photosynthetic activity and cell fitness.
Introduction
The global challenges of the present day, including mitigation of climate change and scarcity of natural resources, emerge a transition from a linear fossil-based economy to a circular sustainable (bio)economy. There is an urgent need to develop sustainable fossil-free production platforms for fuels and chemicals. Photosynthetic microorganisms (e.g. cyanobacteria, microalgae) are considered as a third-generation sustainable feedstock for the chemical industry. They utilize solar energy to convert CO2 into biomass and energy-rich organic compounds. These microorganisms are also capable of holding novel synthetic production pathways that allow them to function as living cell factories for the production of targeted chemicals and fuels.1–3 Among cyanobacteria, Synechocystis sp. PCC 6803 (hereafter Synechocystis) is the most popular model organism with extensively characterized metabolic engineering tools.4–6
Ethylene is the main building block for the production of plastics, fibers, and other organic compounds, also it has a higher energy density (47 MJ kg−1) and carbon content (92 wt% mass fraction) that makes ethylene an attractive fuel source.7,8 Currently, ethylene is extensively produced from energy-intensive steam-cracking of fossil fuel-derived hydrocarbon feedstocks. This process generates significant greenhouse gas and also co-produces extensive amounts of toxic compounds. Hence, alternative routes to environmentally friendly and sustainable ‘green’ ethylene production are being studied. Ethylene is biologically synthesized by plants as a signal molecule regulating plant growth and development, as well as a stress response.9 Some plant pathogens such as Pseudomonas syringae synthesize ethylene by ethylene forming enzyme (Efe) via a single-step reaction utilizing a key substrate i.e., 2-oxoglutarate (2-OG).10 The extensively studied Efe from Pseudomonas syringae was heterologously expressed in the cyanobacterial species including Synechococcus elongatus PCC 794211–13 and Synechocystis.7,14–18 Although good progress has been reported with the proof-of-the concept ethylene producing recombinant cyanobacteria, the overall efficiency of the available ethylene production systems is still low. Techno-economic analysis of bioethylene production by the recombinant cyanobacterium identified ethylene productivity as the most critical variable for cost reduction.19
In this work, we firstly optimized various culture parameters such as radiance and HCO3− concentration in order to enhance the yield of ethylene production in Synechocystis efe mutant. In the next step, we applied thin-layer artificial biofilm technique for improving ethylene production by Synechocystis efe mutant. It is known that natural microbial biofilm formation provide a protected mode enabling cells to survive in harsh environments and share resources from the neighbouring cells.20–23 Microbial biofilms can lead to economic losses (e.g. in food industry, hospitals) but also can be successfully employed in biotechnology.24,25
Artificial biofilm development by entrapping the microalgal cells into thin-layer polymer matrices has been recently demonstrated to be an alternative approach that strongly limits the cell growth, engages efficient flux of photosynthetic reductants to the desired end-products in a controlled manner.26–29 Alginate is the most often used natural and thus, biodegradable polymer, which is composed of guluronic and mannuronic acid residues. At room temperature, formation of the water-insoluble alginate matrix can be achieved by direct interaction of sodium alginate with divalent ions, like Ca2+ and Ba2+ due to electrostatic interaction between the carboxylic groups on the guluronic acid residues and Ca2+ ions.30
This study demonstrates that application of a thin-layer biofilm technology to Synechocystis efe mutant represents a proof-of-concept for long-term (38 days) photosynthetic ethylene production with high (above 1.5%) light to ethylene conversion efficiency.
Materials and methods
Strains and growth conditions
Synechocystis wild-type was originally obtained from Prof. A. Kaplan (Hebrew University of Jerusalem, IL), and ethylene producing Synechocystis efe mutant (S5 strain) from Dr P. Kallio (University of Turku, FI). In this mutant, the ethylene-forming enzyme (encoded by efe from Pseudomonas syringae) was heterologously expressed and the highest translational efficiency of efe gene has been achieved by its modified ribosomal binding site from native cyanobacterial genes.17 The Synechocystis cells were cultivated in BG11 medium31 with the supplementation of 5 mM HEPES–NaOH (pH 7.5). The cells were grown photoautotrophically at 30 °C on a rotary shaker (120 rpm) in the 1% CO2 aerated chamber. The cells were illuminated with continuous fluorescence light of 35 μmol photons m−2 s−1 PAR (Photosynthetically Active Radiation). The mutant cells were maintained in the presence of 25 μg mL−1 spectinomycin and 10 μg mL−1 chloramphenicol. The experimental cultures were grown under the same growth conditions in 1 L flat Roux bottles containing 600 mL of BG11 medium. These cultures were continuously sparged with sterile filtered air with 1% CO2 (filter with pore-size 0.2 μm, Acro 37 TF, Gelman Sciences, USA). For all the experiments, the cells were grown without antibiotics and harvested in their exponential growth phase.
Ethylene production assay and ethylene quantification
2–3 mL of Synechocystis efe cultures were transferred into a gas-tight 23.5 mL vials and supplemented with final concentration of 1 mM of isopropyl-β-D-thiogalactopyranoside (IPTG) (100 mM stock solution) and different concentrations of NaHCO3 (1 M stock solution prepared in BG11 medium), functioning as an inducer and Ci (inorganic carbon) source, respectively. The tightly sealed vials were kept in a growth chamber at 30 °C under continuous illumination from top (35 μmol photons m−2 s−1 PAR, if otherwise not indicated) with fluorescence lamps (Philips TL-D 36 W/865).
The ethylene level was monitored every 24 h using gas chromatography (GC, Clarus 580, PerkinElmer, Inc.). GC was equipped with Carboxen 1010 PLOT 30 m × 0.53 mm capillary column and a flame ionization detector set at 150 °C with H2 and air flow fixed at 30 mL min−1 and 300 mL min−1, respectively. Argon (AGA, Finland) was used as a carrier gas at a flow rate of ∼9.8 mL min−1 (18 psi). 40 μl gas sample were taken from the head-space of vials containing Synechocystis efe cells using a gas-tight Hamilton syringe (Hamilton Company, USA) and injected into the GC. The quantity of ethylene was determined based on the calibration curve, which was obtained using different volumes of a commercial gas standard (1% v/v C2H4 in N2, AGA, Finland). The ethylene production yields were normalized either per a litre of culture for suspension cultures or per m2 of films for the immobilized cells. To allow the direct comparison between suspension and immobilized cultures, the ethylene production rate was estimated based on their initial total Chlorophyll (Chl) content.
Cell immobilization procedures
Synechocystis efe cells were pelleted by centrifugation at 5000g (JA-10 Fixed-Angle Rotor, Beckman Coulter) for 5 min. The cells were entrapped in thin Ca2+-alginate films as described previously28 with minor modifications. In the current research, the blotting paper was used as a support. Cell entrapment was carried out by using a formulation ratio of 1 g of wet cell biomass and 1 mL of 1% alginate (#71238, Sigma-Aldrich). The alginate matrix gelation was initiated by spraying 50 mM CaCl2 solution. The alginate films were cut into 3 × 1 cm strips, placed onto the foam sponge support, and transferred into 23.5 mL vials containing 3 mL of BG11 medium. To ensure efficient production of ethylene by Synechocystis efe cells, 200 mM NaHCO3, if not mentioned otherwise, and 1 mM IPTG was introduced to the cultures. The ethylene quantification was performed as described above.
Efficiency of PAR light energy conversion to ethylene
The efficiency of light energy conversion to ethylene (LECE) was calculated assuming its photosynthetic biosynthesis from H2O and CO2. In this approach, we assume that formation of ethylene proceeds in two stages:
resulting in the overall reaction: 2H2O + 2CO2 → C2H4 + 3O2. For calculation of LECE, the following equation was applied:
where ΔG° is the standard Gibbs free energy change of ethylene biosynthesis (1331.4 kJ mol−1 at 25 °C), Ret is the rate of ethylene photoproduction (mol h−1), ES is the energy of the incident light radiation (kJ m−2 h−1), t is the duration of the experiment (h) and A is the surface area of the films (m2). The irradiance was determined by the SpectraPen LM 500 (ICT International, Australia) in the PAR region. For estimation of LECE based on enthalpy of ethylene combustion (ΔcH° = −1411.2 kJ mol−1 at 25 °C, NIST Chemistry WebBook, http://webbook.nist.gov) as presented by Lips et al.,3 all efficiencies given in our research should be multiplied by 1.06. The calculated efficiency values are only estimates due to uncertainties in the real reaction stoichiometry. Therefore, these values could not be directly compared to other technologies such as solar cells or solar-to-fuels.
Photosynthetic activity
The photosynthetic efficiency of cyanobacterial suspensions and immobilized cells were measured using AquaPen-C AP-C 100 hand-held fluorometer (Photon Systems Instruments, Czech Republic). All measurements were performed in single-use polystyrene cuvettes (optical length 1 cm). According to the manufacturer's manual, a predefined QY protocol was applied with saturating pulses (3000 μmol photons m−2 s−1) on top of the actinic light (50 μmol photons m−2 s−1) to probe the effective yield of Photosystem II, Y(II), in light-adapted state.
Cell density and Chl a determination
The cell density of the suspension cultures was determined spectrophotometrically by measuring optical density at 750 nm (OD750). Prior to the measurements of Chl a content, the Synechocystis suspension cells were harvested and dark incubated with 90% methanol for 5 min. The films with entrapped cells were incubated in the dark with 90% methanol at 65 °C for 30 min.29 All samples were harvested at 5000 g for 5 min and Chl a was determined spectrophotometrically at 665 nm.32
Absorption spectroscopy
In situ absorption spectra (370–750 nm) of cell suspensions (OD750 = 0.8) were measured by the OLIS CLARiTY 17 UV/VIS/NIR spectrophotometer with the integrating cavity (On Line Instrument Systems, Inc.). The raw absorbance data of the samples were converted into absorbance values by using Fry's method.33 Prior to the measurements, the cells were released from Ca2+-alginate films by 50 mM EDTA (pH 7.0), and washed with BG11 medium.
Results
We used the Synechocystis efe mutant with heterologous expression of the ethylene-forming enzyme (encoded by efe from Pseudomonas syringae) enabling the conversion of intracellular 2-OG into ethylene via TCA cycle.17
Effect of different radiances on ethylene production
To study the effect of different radiances on ethylene yield, we subjected the ethylene producing Synechocystis efe mutant suspensions to moderate (35 μmol photons m−2 s−1) and relatively high- (100 μmol photons m−2 s−1) radiances. Prior to the light experiments, the efe cells were supplemented with an inducer (1 mM IPTG) and Ci source (20 mM NaHCO3). 2 mL culture was placed in a gas-tight vial and ethylene production was monitored every 24 h by gas chromatography.
Synechocystis efe cells, when placed in dark, produced a residual amount of ethylene (Fig. 1A) and showed a stable Chl content even after 72 h of incubation (Fig. 1B and C). Contrary to this, strong ethylene production was monitored during the first 24 h of illumination (Fig. 1A). Under high-light, the cultures produced slightly less amount of ethylene than under moderate light: 0.041 mmol L−1 and 0.053 mmol L−1, respectively. In our experiments, continuous light illumination was used to eliminate the possible effects of day-night cycles on ethylene production. At 48 h, the cumulative ethylene yields further increased to ∼0.059 mmol L−1 and 0.050 mmol L−1 under moderate and high-light, respectively. An increase in ethylene yield was accompanied by an increase in the Chl content. Under both radiances, the experimental cultures demonstrated ∼2.3 times higher Chl content by 24 h of incubation (Fig. 1B and C). No further ethylene production was observed under both light conditions after 48 h (data not shown). Simultaneously, cultures substantially decreased Chl content (Fig. 1C) and demonstrated a visual shift in the pigment composition (Fig. 1B). It is important to note that a relatively higher ethylene yield under moderate radiance was observed even if the yield was normalized to Chl amount (Fig. 1A and Fig. S1A, B†). Therefore, we decided to apply the moderate radiance (35 μmol photons m−2 s−1) for further optimization of ethylene production in the Synechocystis efe mutant. We also would like to emphasize that efe mutant produced a residual amount of ethylene (0.01 mmol L−1 at 48 h) even without IPTG inducer due to the leakage in the lac promoter (Fig. S2†).
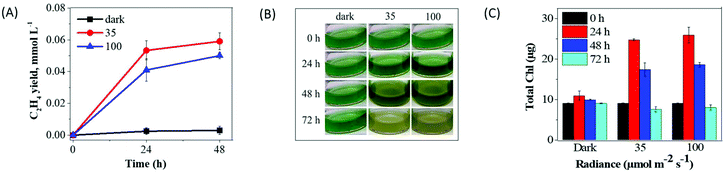 |
| Fig. 1 Effect of different radiances on ethylene production in Synechocystis efe mutant. (A) Cumulative ethylene yield in the dark and under 35 and 100 μmol photons m−2 s−1 radiances; (B) visual phenotypic changes of cells under ethylene production conditions; (C) total Chl content. Prior to the experiment, the cells were harvested in their exponential growth phase and Chl a concentration was adjusted to a final concentration of 4.5 μg mL−1 ( 2 mL cell suspension in 23.5 mL vial) with fresh BG11 medium (pH 7.5) supplemented with 20 mM NaHCO3 (as Ci source). Ethylene production was induced with 1 mM IPTG in the tightly sealed vials. Each experimental point represents 3 independent measurements from 3 vials (±SD). | |
Influence of different treatments on cell fitness in suspension cultures
In order to study the effects of the IPTG (an inducer), inorganic carbon (Ci) source and ethylene accumulation on cell fitness, Synechocystis efe mutant and its corresponding wild-type (hereafter, WT) cultures were incubated (i) in the absence of IPTG and NaHCO3 (negative control); (ii) in the presence of 20 mM NaHCO3; (iii) in the presence of 20 mM NaHCO3 and 1 mM IPTG; and (iv) in the presence of 20 mM NaHCO3 and exogenously added 1% ethylene to the headspace of the vials. Both Synechocystis WT and efe cells demonstrated strong growth inhibition in the sealed vials (Fig. 2A) without supplementation of external Ci source (the standard BG11 medium contains 2 mM Na2CO3 as Ci source). This was accompanied by a considerable decrease in OD750 and Chl amount monitored at 48 and 96 h (Fig. 2B–E). On the contrary, supplementation of cultures with only 20 mM NaHCO3 resulted in a higher growth of WT and efe mutant as deduced from the increased OD750 and Chl amount at 48 h. However, at 96 h Chl amount of both cultures declined strongly to a lower than the initial level. OD750 of both cultures (Fig. 2B and C) also decreased but to a lesser extent than Chl (Fig. 2D and E). It is important to note that the efe mutant demonstrated a stronger decrease in OD750 and Chl content compared to WT. The heterologous expression of efe in the Synechocystis cells did not alter the carotenoid profile when compared to WT cells under growth condition (Fig. S3†), thus excluding the influence of carotenoids on the bleaching phenotype of efe cells in the sealed vials under illumination. Collectively, the obtained data suggest that the Synechocystis cells become rapidly Ci limited and exogenously added Ci source is necessary for supporting cell fitness in sealed vials.
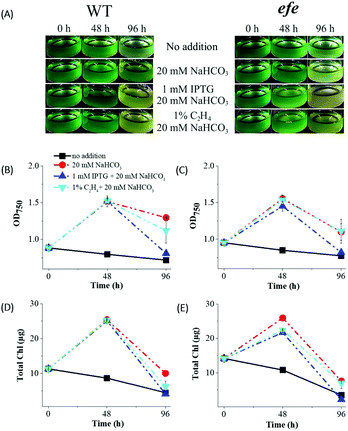 |
| Fig. 2 Effects of an inducer (1 mM IPTG), inorganic carbon (20 mM NaHCO3), and exogenously added ethylene on Synechocystis WT and efe cultures. (A and B) phenotypic change; (B and C) changes in OD750 and (D and E) Chl content under continuous illumination (35 μmol photons m−2 s−1). For the supply of 1% ethylene, the >99% ethylene was diluted in ambient air prior to the experiment. The error bars represents the SD of 3 independent measurements from 3 vials. | |
At 48 h, the cultures supplemented with 1 mM IPTG and 20 mM NaHCO3 demonstrated similar growth to the cultures supplemented with only 20 mM NaHCO3. However, by 96 h addition of IPTG led to a stronger decrease in the OD750 and Chl compared to the cultures with only NaHCO3. In order to clarify the IPTG effect on cultures, we have performed similar experiments (1 mM IPTG and 20 mM NaHCO3) in the flasks with efficient gas exchange (Fig. S4†). Contrary to the experiments performed in sealed vials, the cells demonstrated strong growth as indicated by increases in OD750 and Chl. The obtained results suggest that an inhibitory phenotype observed in the presence of IPTG during a long-term experiment (96 h) is due to cultivation in the closed system and Ci limitation.
In order to investigate the possible toxic effect of ethylene on the Synechocystis cells, we exogenously added 1% ethylene to the headspace of the tightly sealed vials containing efe mutant and its corresponding WT. The cultures supplemented with 1% ethylene and 20 mM NaHCO3 demonstrated similar OD and Chl pattern compared to the reference cultures containing 20 mM NaHCO3 only (Fig. 2). Even under a high dose of ethylene (>99% in the headspace), the cell growth was not inhibited during 96 h (Fig. S5†). The obtained results confirmed that ethylene accumulation is not toxic to the Synechocystis cells at least under the studied conditions, which is in agreement with previous data.7,13
Effects of HCO3− supplementation on cell fitness and ethylene production yield in Synechocystis efe suspensions
The results obtained above demonstrate that the cultures placed in the sealed vials easily become Ci deprived. Thus, supplementation of the cultures with an optimal Ci concentration would be important for the enhanced ethylene production and also for the survival of the cells in the closed system. NaHCO3 is an excellent inorganic carbon source for industrially important cyanobacteria and microalgae.34–36 Therefore, in the next series of experiments we have studied the effects of various NaHCO3 concentrations (without altering the level of IPTG inducer) on cell viability, photosynthetic activity, and ethylene productivity.
As shown in Fig. 3A, supplementation of the cultures with 100 mM NaHCO3 resulted in a ∼5.5-fold increase in cumulative ethylene yield (0.39 mmol L−1) compared to the cultures with 20 mM NaHCO3 (0.07 mmol L−1) during 72 h of production. The further increase in NaHCO3 concentration (200 mM) caused a further enhancement in ethylene productivity (0.46 mmol L−1). Nevertheless, 300 mM NaHCO3 led to a relatively lower ethylene yield (0.21 mmol L−1). The obtained results suggest that the increase in the NaHCO3 concentration to 100 or 200 mM has a positive impact on the enhancement of ethylene production. All cultures supplemented with different concentrations of NaHCO3 demonstrated photosynthetic yield Y(II) of 0.5 at 0 h, which is typical for healthy cyanobacterial cells. The photosynthetic performance of the cultures supplemented with 100 and 200 mM NaHCO3 did not change during the first 24 h. In contrast, the cultures incubated with the 20 mM and 300 mM NaHCO3 demonstrated a strong photosynthetic inhibition already at 24 h and thereafter (Fig. 3B). The efe cultures supplemented with 100 and 200 mM NaHCO3 demonstrated high biomass accumulation and an approximately 6-fold increase in the Chl content after 72 h of incubation (Fig. 3D). It is important to note that the efe cultures supplemented with a high concentration of NaHCO3 (100–300 mM) showed bleaching by 120 h of treatment (Fig. 3C), which was accompanied by a strong decrease in Chl content (Fig. 3D) and photosynthetic performance (Fig. 3B).
 |
| Fig. 3 Effects of NaHCO3 concentrations on cell fitness and ethylene production yield in Synechocystis efe mutant. (A) Cumulative ethylene yield; (B) photosynthetic activity; (C) growth phenotype and (D) Chl a content monitored at indicated time intervals. The cells were harvested and Chl a concentration was adjusted to a final concentration of 4.3 μg mL−1 (v = 3 mL) before supplementing with different concentrations of NaHCO3 (20–300 mM). Each experimental point represents 3 independent measurements from 3 vials (±SD). | |
Supplementation of the culture media with a high concentration of NaHCO3 could affect a cell metabolism via possible changes in pH. Regardless of different NaHCO3 concentrations, the pH of the initial culture increased from 7.5 to 7.9–8.0 immediately after the addition of NaHCO3 (Table S1†). After 24 h of incubation, pH further increased to 10–10.5 and continued to be alkaline until the end of the experiment (96 h). An increase in pH (7.5 to 10.5) did not seem to affect the photosynthetic performance of the ethylene producing Synechocystis efe cells (Fig. 3B, see 100 and 200 mM NaHCO3) after 24 h incubation. The obtained results suggest that optimally high concentration (100 and 200 mM) of NaHCO3 enhances ethylene production and prolongs cell viability (Fig. 3). These results also emphasize that a high concentration of NaHCO3 strongly increases the growth rate of the suspension cultures in closed vials. However, such rapid growth might have its flip side of causing nutrient starvation during the long-term production experiments (e.g. 120 h).
Ethylene production in the semi-continuous suspension cultures
Since inoculum size can influence the fitness of cells and also a production yield, we performed new experiments with dense efe cultures. During 3 days of ethylene production, the dense suspension cultures (initial total Chl ∼34 μg) demonstrated ∼1.2-fold higher ethylene yield (Fig. 4A) compared to the suspension cultures with low Chl (initial total Chl ∼12.9 μg) (Fig. 3A). However, ethylene production in dense cultures strongly declined after 3 days similarly to the low-density cultures and eventually strong bleaching was observed at the 5th day (Fig. S6B†).
 |
| Fig. 4 Effect of periodically replenished suspension cultures of Synechocystis efe mutant on the ethylene productivity. (A) The yield of ethylene (the culture is supplemented with 1 mM IPTG and Ci source 200 mM NaHCO3); (B) growth phenotypes; (C) Chl content and photosynthetic activity of the suspension cultures; (D) whole-cell absorption spectra of the samples. The spectra were recorded on an equal content of cells basis (OD750). After a strong decline in ethylene production (3–4 days), the suspension cells were periodically replenished with fresh BG11 medium (3 mL in 23.5 mL vial) containing inducer and Ci source. The total yield of ethylene productivity was normalized to the initial total Chl content. Each experimental point represents 3 independent measurements from 3 vials (±SD). The spectra were normalized to the Chl absorption measured at 438 nm. | |
The whole-cell absorption spectra recorded from efe cells at the 5th d of ethylene production revealed a strong decrease in the region of phycobiliproteins (around 625 nm) of phycobilisomes (PBS), a large cyanobacterial antenna complex. At the same time, only a small decrease in the carotenoids (∼480 nm) and Chl (∼685 nm) peak was observed (Fig. S6A†). The fast degradation of PBSs could be accounted for the severe drop in medium nutrients,35 mainly nitrogen in the rapidly grown suspension cultures supplemented with a high concentration of NaHCO3. Thus, such nutrients depletion might cause excessive bleaching, and complete inhibition of photosynthetic activity thus ultimately limiting ethylene production. Incubation of the efe mutant cells with 5× BG11 medium (5 times higher nutrient concentration) supplemented with 1 mM IPTG and 200 mM NaHCO3 delayed but not eliminated a bleaching phenotype (Fig. S6†). These results further supported that the nutrient limitation at least to some extent accounted for the degradation of photosynthetic pigments.
The results described above suggested that long-term ethylene production can be achieved by periodically replenishing cultures with fresh BG11 medium (supplemented with 1 mM IPTG and 200 mM NaHCO3). The refreshment was periodically performed every 3–4 days. As a result, replenished suspension cultures produced ethylene without exhibiting a strong bleaching phenotype for a longer period (more than 2 weeks) (Fig. 4A). In the replenished suspension culture, excessive cell bleaching has been noticed only after 17 days (Fig. 4B), which is accompanied by inhibition of photosynthetic activity (Fig. 4C) and a strong decrease in PBS (Fig. 4D) and ethylene production. The obtained results suggest that periodically replenished suspension cultures prolong ethylene production maximum of 2 weeks.
Ethylene production by efe mutant entrapped within Ca2+-alginate films
Next, we applied the immobilization technique to the ethylene producing Synechocystis efe mutant. It is known that immobilized microalgae can act as long-lived bio-catalysts by re-routing photosynthetic reductants to the production of desired products, instead of biomass accumulation.29,38 Therefore, we attempted to engineer thin-layer alginate films with entrapped Synechocystis efe cells as a long-term production strategy.
The efe cells entrapped within thin-layer alginate films were placed on top of a foam sponge in gas-tight vials. The medium was supplemented with various concentrations of NaHCO3 (from 20–600 mM) and ethylene production was monitored periodically under continuous moderate light (Fig. 5A). The efe cells entrapped in a thin-layer film supplemented with 20 mM NaHCO3 produced ethylene (∼1.23 mmol m−2) during 48 h, then stopped the production. The cells supplemented with 200 mM NaHCO3 demonstrated 9-fold higher ethylene production (∼10.95 mmol m−2) during 96 h compared to the cells incubated with 20 mM NaHCO3. It is important to note that suspension cultures demonstrated only a 5-fold increase in the presence of 200 mM NaHCO3 (Fig. 3A). Moreover, in the suspension cultures, a strong decline in ethylene production was observed already at 72 h (Fig. 3A), whereas immobilized cells continued producing ethylene for 144 h (Fig. 5A). Under 200 mM NaHCO3 loading, the immobilized cells were not prone to bleaching even at 144 h, only a slight bleaching phenotype was visible in the presence of low concentration (20 mM) of NaHCO3 (Fig. 5B).
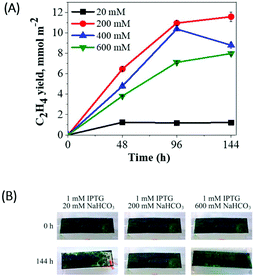 |
| Fig. 5 The ethylene production in the Synechocystis efe cells entrapped in Ca2+-alginate thin-layer films. (A) Cumulative ethylene yield; (B) the visual phenotype of the Synechocystis efe films. The films (3 cm2) contained ∼66 μg Chl. Each experimental point represents 3 independent measurements from 3 vials (±SD). | |
The further increase in NaHCO3 concentration (400 and 600 mM) resulted in a relatively low ethylene yield compared to 200 mM NaHCO3, this tendency was already encountered in the suspension cultures (Fig. 3A). The obtained results suggest that 200 mM NaHCO3 is optimal for the enhancement of ethylene productivity not only in the suspension (Fig. 3A) but also in immobilized cells (Fig. 5A). A longer period (up to 144 h) of ethylene production can be achieved by immobilization of the cells.
Effect of periodic replenishment cycles on ethylene productivity in thin films
In contrast to suspension cultures (3–4 d), alginate entrapped efe cells demonstrated a decline in ethylene production activity by the 5th day of the experiment (Fig. 6A). At this point, the medium in the vials was refreshed and supplemented with IPTG and NaHCO3, thus a new cycle was initiated. During the 24 h of incubation, the ethylene production rates in immobilized and suspended cells corresponded to 1.28 and 0.84 μmol C2H4 (mg Chl h)−1, respectively (Fig. S7†). The difference became even more pronounced by the 3rd day, where immobilized cells showed 7.7 times higher ethylene production rate (0.62 μmol C2H4 (mg Chl h)−1) than the suspension cells (0.08 μmol C2H4 (mg Chl h)−1). During the 1st cycle (1–5 d) of the production phase, the immobilized cells achieved approximately 84 μmol C2H4 (mg Chl)−1 yield (Fig. 6A), while the suspension cultures in batch mode (3 d) produced maximum 43 μmol C2H4 (mg Chl)−1 (Fig. 4A).
 |
| Fig. 6 Effect of periodic refreshment of media and gas phase on the ethylene photoproduction by immobilized cells. (A) Ethylene yield measured every 24 h after the induction with 1 mM IPTG; (B) the visual phenotypes of the Synechocystis efe films; (C) Chl content and photosynthetic activity; (D) whole-cell absorption spectra were monitored periodically. Every 5 d immobilized cells were periodically replenished with fresh BG11 medium supplemented with 1 mM IPTG and 200 mM NaHCO3. Each experimental point represents 3 independent measurements from 3 vials (±SD). The spectra were recorded on an equal content of cells basis (OD750) and normalized to the Chl absorption measured at 438 nm. | |
The Synechocystis efe cells entrapped in Ca2+-alginate film demonstrated prolonged production of ethylene under semi-continuous mode over 7 cycles (38 d) (Fig. 6A and Fig. S8A†) than the suspension cultures, which functioned maximum for 5 cycles (15 d) (Fig. 4A and Fig. S8B†). After the first cycle, the peak production of ethylene gradually decreased at each cycle in immobilized cells (1st cycle to 7th cycle: 84 to 35 μmol C2H4 (mg Chl)−1), also in the suspension cultures (1st cycle to 5th cycle: 39.4 to 18 μmol C2H4 (mg Chl)−1). At the end of the experiment, ∼2.2 fold higher yield of ethylene and 2 times longer periods of production was achieved from the immobilized cells (∼383 μmol C2H4 (mg Chl)−1) than the suspension culture system (∼174 μmol C2H4 (mg Chl)−1).
As presented in Fig. 6C, the entrapment of the efe cells did not completely inhibit its growth/Chl content (from ∼26 to ∼55 μg Chl at 30 d), but strongly restricted compared to the suspension cultures (from ∼33 to ∼133 μg Chl at 10 d) (Fig. 4C). Moreover, entrapped cells did not demonstrate bleaching in the 30 d of ethylene production phase and still were photosynthetically active (Fig. 6B and C). The whole-cell absorption spectra demonstrated a small decrease in the phycobilin absorbance peak at the 30 and 38 d of production (Fig. 6D) whereas complete PBS degradation was encountered in 18 d of suspension cultures (Fig. 4D). The results obtained indicated that entrapment of the cells in Ca2+-alginate films prolongs and enhances the photoproduction of ethylene and strongly improves cell fitness.
Light-to-ethylene conversion efficiency in Synechocystis efe cells
Besides extended productivity, the light to product conversion efficiency is one of the important factors for the industrial success of bio-based products (i.e., ethylene). Therefore, we evaluated the PAR LECE to ethylene in Synechocystis efe cultures.
In the first series of experiments, we entrapped Synechocystis efe cells within thin films and subjected them to different radiances: 2, 4, 7, and 46 W m−2 (9, 18, 30, and 205 μmol photons m−2 s−1, respectively). Among all applied radiances, the maximum yield of ethylene (9.14 mmol m−2) was obtained under 4 W m−2 and a minimum yield of ethylene (4.97 mmol m−2) was observed under high-light (46 W m−2) at 8 d (Fig. 7A). At this time period, the ethylene production had reached its stationary level in almost all samples. The maximum PAR LECE (based on the maximum rate) was observed under 2 W m−2 (1.54%), whereas lower efficiency 0.08% was obtained under 46 W m−2 (Fig. 7B). Similarly, the maximum PAR LECE for the overall ethylene production yield (0.64%) was obtained under 2 W m−2 whereas the minimum (0.04%) under 46 W m−2. As expected more efficient light utilization occurs under low light, where PAR LECE converges to the theoretical maximum.
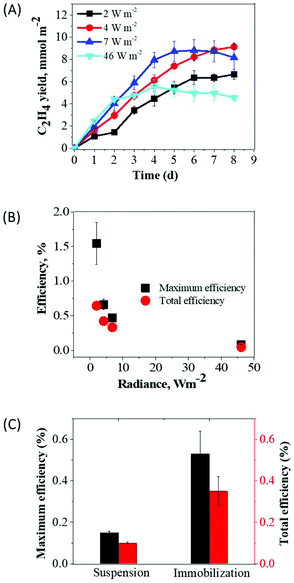 |
| Fig. 7 The light to ethylene conversion efficiencies by suspension and immobilized Synechocystis efe cultures. (A) The yield of ethylene by immobilized cultures under different radiances; (B) the light to ethylene conversion efficiencies (%) calculated for the maximum rate and for the total period of ethylene photoproduction in thin-films; the films (3 cm2) contained ∼43 μg Chl. The panel (C) compares light to ethylene conversion efficiencies in suspension and immobilized cultures of Synechocystis efe mutant under 35 μmol photons m−2 s−1. The efficiencies were calculated for the maximum production rate and for the total yield of ethylene during the 1st cycle (Fig. 4A and 6A). Each experimental point represents 3 independent measurements from 3 vials (±SD). | |
Next, we compared PAR LECE for Synechocystis efe cells in suspension cultures and in thin Ca2+ alginate films during the 1st production cycle at about 8 W m−2 radiance (Fig. 7C). The PAR LECE calculated from the maximum production rate (suspension 24–48 h and immobilization 0–24 h, Fig. 4A and 6A) was 0.15% in the suspension cultures, while immobilized cells demonstrated 0.53% efficiency. Similarly, PAR LECE calculated for the total period (suspension 72 h and immobilization 96 h) of ethylene production during the 1st cycle was more than 3-fold higher in the immobilized cells (0.35%) compared to the suspension cultures (0.10%). These results showed that a thin layer immobilization system, not only improved the yield of ethylene production but also substantially increased the light-to-product conversion efficiency.
Discussion
Optimal radiance and HCO3− concentration improve cell fitness and ethylene productivity
Light fuels photosynthetic processes. For this reason, the production of ethylene in Synechocystis efe cultures was dramatically enhanced by the light (Fig. 1A). Our study demonstrates that moderate radiance (30–35 μmol photons m−2 s−1, or 7–8 W m−2) is optimal for ethylene production in both suspensions and immobilized cultures under the studied conditions (Fig. 1A and 7A). A shift to either a low (down to 9 μmol photons m−2 s−1, or 2 W m−2) or to high (up to 205 μmol photons m−2 s−1, or 46 W m−2) radiances decreased the ethylene production yield (Fig. 1A and 7A). The small amounts of ethylene observed in dark samples most probably originated from stored carbon reserves accumulated in Synechocystis efe cells during photosynthesis. Various reports on the effect of different radiances and their quality on the ethylene production have been presented. Different from our findings, the enhancement of ethylene production in Synechocystis was demonstrated under 200 μmol photons m−2 s−1 (ref. 15) and 600 μmol photons m−2 s−1 of white light.7 The improved ethylene rate was also reported under 50 μmol photons m−2 s−1 of monochromatic red light with an additional 10 μmol photons m−2 s−1 of white light.39 Similar to our study, Xiong and co-authors40 showed that ethylene can be efficiently produced under moderate light (50 μmol photons m−2 s−1) via the TCA cycle of the genetically modified Synechocystis strain. They also demonstrated that under this light condition, Synechocystis cells converted around 10% of fixed CO2 to ethylene. Thus, utilization of various light for ethylene production seems to depend on the experimental setup: cell density, culture volume, employed bioreactor, and engineered strain.
In aquatic environments, CO2 diffusion is lower than in air and controlled via chemical equilibrium with bicarbonate (HCO3−) or carbonate (CO32−) depending on the medium pH. In plants and aquatic phototrophs, both CO2 and HCO3− can serve as main Ci sources for photosynthetic processes.41,42 However, the efficiency of HCO3− utilization is higher than CO2, mainly due to a higher solubility of bicarbonate salts in water (for example, NaHCO3 solubility is above 90 g L−1 at 25 °C, while CO2 is around 1.5 g L−1 at 25 °C). Hence, in this study NaHCO3 is applied as a Ci source for CO2 assimilation and ethylene production. Supplementation of the cultures with 20 mM NaHCO3 enabled and prolonged cell growth in the sealed vials during ethylene production (Fig. 2). Further increase of NaHCO3 up to 200 mM tremendously enhanced cell growth (Fig. 3D), stabilized photosynthetic activity (Fig. 3B), and improved ethylene production yields (Fig. 3A). This finding is in agreement with a previous study where supplementation of the Synechocystis mutant with other carbon source i.e., xylose enhanced ethylene production via the TCA cycle.43 Additionally, the positive effect of increased concentration of NaHCO3 (up to 180 mM) in microalgal cultures has already been reported not only on the cell growth and lipid content35,36 but also on ethanol and 1-butanol photoproduction yields.44,45 However, under very high amounts of bicarbonate the metabolic activity of cells is often inhibited by the substrate concentration or unbalanced osmotic pressure.46,47 Indeed, supplementation of 300–600 mM NaHCO3 in our study reduced cell growth (Fig. 3) and the ethylene production yield (Fig. 3 and 5).
Semi-continuous cultivation improves ethylene production yield
Under saturating concentrations (100–200 mM) of NaHCO3, Synechocystis efe suspension cultures demonstrate a strong growth (until 3rd day), followed by pigment bleaching and termination of ethylene production (5th day, Fig. 3). The bleaching phenotype might be linked to a strongly increased culture growth under high Ci concentration, which induces a nutrient limitation in the middle of the experiment with the following chlorosis. It is well known that macronutrient depletion (mainly nitrogen, sulphur or phosphorus) leads to rapid degradation of photosynthetic pigment-protein complexes. This is important for supplying cells with amino acid residues, which ensures their survival under nutrient limitation.37,48,49 Accordingly, deprivation of nutrients, particularly N, led to severe PBSs degradation (Fig. S6A†) and strong inhibition of photosynthetic activity in the suspension cultures observed at the 5th day (Fig. 3D). Application of 5-fold concentrated BG11 growth medium does not abolish a degradation of PBSs observed at 5th day but delays de-pigmentation (Fig. S6†) suggesting that nutrient limitation is not the only factor limiting the cell fitness, photosynthetic activity and ethylene production yield in the suspension cultures under the studied conditions. Under the semi-continuous mode, a periodic refreshment of the cells with growth medium (every 3rd day) by providing nutrients and eliminating possible toxic secondary metabolites from the spent medium strongly delays the inhibition process demonstrating only slight changes in the photosynthetic yield, PBS absorption peak at 10 days, and a sustained ethylene production for 16 days (Fig. 4). However, even periodic replacement of media does not sustain cell performance and photosynthetic activity after the 5th cycle demonstrating a complete inhibition of photosynthetic yield and ethylene production activity at the 18th day (Fig. 4).
2-OG derived from carbon fixation acts as a key substrate for both, the efe enzyme and N assimilation, and also serves as a signalling molecule during N-limitation.50,51 Supplementation of the cultures with high HCO3− concentrations affects cell metabolism via changes in intracellular C/N ratio through the accumulation of 2-OG. However, efficient utilization of 2-OG by recombinant ethylene production pathway would limit substrate supply for N-assimilation in Synechocystis efe cells disturbing intracellular C/N homeostasis. This in turn might weaken the metabolic plasticity required for cell survival as well as ethylene production in our experimental conditions. In response to environmental and metabolic stress, high density suspension cultures presumably undergo programmed cell death.52–54 We also could not exclude the involvement of massive production of reactive oxygen species (ROS) in this process.
Immobilization enhances and prolongs ethylene production by minimizing biomass accumulation
In contrast to suspension cultures, the immobilized Synechocystis efe cells produced ethylene over 7 cycles (38 days), resulting in a 2-time enhancement in the duration of ethylene production and a 2.2-fold increase in the production yield (Fig. 4 and 6). Application of similar biomass load (starting Chl content is 27–34 μg) and light irradiation allows a direct comparison of two different production systems. It is important to note that observed long-term ethylene production by the immobilized cells also addresses the genetic stability of efe cells (Fig. 6A). The entrapment of Synechocystis mutant in thin-layer Ca2+-alginate films strongly decreases energy and nutrient losses to biomass production28 by limiting, at least partially, cell growth (Fig. 6C). This reduces problems with nutrient limitations during the production cycles. Moreover, a protective polymer layer shielding cells and improving cell-to-cell communication via quorum sensing55 decreases competition between cells and enables them to achieve an optimal cellular fitness (Fig. 6C).28,56 By uncoupling cell growth from product synthesis, the artificial film biocatalyst allocates photosynthetic reducing power towards ethylene production (Fig. 6). As a result, the immobilized system achieves sustainable photoproduction of ethylene for up to 38 days yielding 822 mL ethylene per m2 of the film.
Besides prolongation of the production process, thin-layer immobilization approach also improves light utilization, and thus allows more efficient distribution of light energy to the product.38,57 Indeed, entrapment of Synechocystis efe cells within thin artificial biofilm strongly enhanced PAR LECE to ethylene (up to 3.5-fold) as compared to the suspension cultures (Fig. 7C). The highest PAR LECE of around 1.54% was achieved under the low radiance of 2 W m−2 (9 μmol photons m−2 s−1) when the cells showed the maximum production rate (Fig. 7B). To the best of our knowledge, only one report on PAR LECE showing a value of 3.58% has been presented. This value was calculated by Lips et al.3 from the previous study,39 which was performed with Synechocystis ethylene producing mutant cultivated in flat-panel chemostat photobioreactor setup. It is important to note that Zavřel et al. research39 was performed under monochromatic red LED light that engages the maximum photochemistry in Synechocystis cells and thus could not be directly compared to our study. Considering that our immobilized system achieves a higher PAR LECE for several days (8 d) at a low radiance of 9 μmol photons m−2 s−1, thus the efficiency of low-light utilization can be vastly improved by the thin-layer immobilization approach.
Our findings open new possibilities for further development of a novel technology platform that targets application of a direct production of ethylene from sunlight using cyanobacteria as real biocatalytic hosts in continuous cultures. Our approach strongly limits biomass accumulation, preserves photosynthetic activity, and maintains cell fitness, thus enabling engineered cyanobacteria to act as long-lived biocatalysts for ethylene photoproduction.
Conflicts of interest
There are no conflicts to declare.
Acknowledgements
The study is financially supported by the Novo Nordisk Fonden (project #NNF16OC0021626), the Academy of Finland (AlgaLEAF project #322754) and the Nordforsk Nordic Center of Excellence program “NordAqua” #82845. We are thankful to Dr Pauli Kallio (University of Turku, FI) for the generous gift of Synechocystis efe mutant.
References
- J. Zhou, T. Zhu, Z. Cai and Y. Li, Microb. Cell Fact., 2016, 15, 1–9 CrossRef.
- N. J. Oliver, C. A. Rabinovitch-Deere, A. L. Carroll, N. E. Nozzi, A. E. Case and S. Atsumi, Curr. Opin. Chem. Biol., 2016, 35, 43–50 CrossRef CAS.
- D. Lips, J. M. Schuurmans, F. Branco dos Santos and K. J. Hellingwerf, Energy Environ. Sci., 2018, 11, 10–22 RSC.
- T. Kaneko, S. Sato, H. Kotani, A. Tanaka, E. Asamizu, Y. Nakamura, N. Miyajima, M. Hirosawa, M. Sugiura, S. Sasamoto, T. Kimura, T. Hosouchi, A. Matsuno, A. Muraki, N. Nakazaki, K. Naruo, S. Okumura, S. Shimpo, C. Takeuchi, T. Wada, A. Watanabe, M. Yamada, M. Yasuda and S. Tabata, DNA Res., 1996, 3, 109–136 CrossRef CAS.
- E. Englund, F. Liang and P. Lindberg, Sci. Rep., 2016, 6, 1–12 CrossRef.
- D. Liu and H. B. Pakrasi, Microb. Cell Fact., 2018, 17, 1–8 CrossRef.
- J. Ungerer, L. Tao, M. Davis, M. Ghirardi, P. C. Maness and J. Yu, Energy Environ. Sci., 2012, 5, 8998–9006 RSC.
- V. P. Haribal, Y. Chen, L. Neal and F. Li, Engineering, 2018, 4, 714–721 CrossRef CAS.
- K. L. C. Wang, H. Li and J. R. Ecker, Plant Cell, 2002, 14, 131–152 CrossRef.
- K. Nagahama, T. Ogawa, T. Fujii, M. Tazaki, S. Tanase, Y. Morino and H. Fukuda, J. Gen. Microbiol., 1991, 137, 2281–2286 CrossRef CAS.
- M. Sakai, T. Ogawa, M. Matsuoka and H. Fukuda, J. Ferment. Bioeng., 1997, 84, 434–443 CrossRef CAS.
- K. Takahama, M. Matsuoka, K. Nagahama and T. Ogawa, J. Biosci. Bioeng., 2003, 95, 302–305 CrossRef CAS.
- V. Carbonell, E. Vuorio, E. M. Aro and P. Kallio, World J. Microbiol. Biotechnol., 2019, 35, 1–9 CrossRef CAS.
- F. Guerrero, V. Carbonell, M. Cossu, D. Correddu and P. R. Jones, PLoS One, 2012, 7(11), e50470 CrossRef CAS.
- T. Zhu, X. Xie, Z. Li, X. Tan and X. Lu, Green Chem., 2015, 17, 421–434 RSC.
- W. Xiong, J. A. Morgan, J. Ungerer, B. Wang, P. C. Maness and J. Yu, Nat. Plants, 2015, 1, 1–6 Search PubMed.
- K. Thiel, E. Mulaku, H. Dandapani, C. Nagy, E. M. Aro and P. Kallio, Microb. Cell Fact., 2018, 17, 1–12 CrossRef.
- C. Durall, P. Lindberg, J. Yu and P. Lindblad, Biotechnol. Biofuels, 2020, 13, 1–13 CrossRef.
- J. N. Markham, L. Tao, R. Davis, N. Voulis, L. T. Angenent, J. Ungerer and J. Yu, Green Chem., 2016, 18, 6266–6281 RSC.
- S. Aiola, G. Amico, P. Battaglia and E. Battistelli, Clin. Microbiol. Rev., 2002, 15, 167–193 CrossRef.
- F. Rossi and R. De Philippis, Life, 2015, 5, 1218–1238 CrossRef CAS.
- L. Hall-Stoodley, J. W. Costerton and P. Stoodley, Nat. Rev. Microbiol., 2004, 2, 95–108 CrossRef CAS.
- H. C. Flemming and J. Wingender, Nat. Rev. Microbiol., 2010, 8, 623–633 CrossRef CAS.
- S. J. Edwards and B. V. Kjellerup, Appl. Microbiol. Biotechnol., 2013, 97, 9909–9921 CrossRef CAS.
- A. Hoschek, I. Heuschkel, A. Schmid, B. Bühler, R. Karande and K. Bühler, Bioresour. Technol., 2019, 282, 171–178 CrossRef CAS.
- M. C. Flickinger, J. L. Schottel, D. R. Bond, A. Aksan and L. E. Scriven, Biotechnol. Prog., 2007, 23, 2–17 CrossRef CAS.
- S. N. Kosourov and M. Seibert, Biotechnol. Bioeng., 2009, 102, 50–58 CrossRef CAS.
- H. Leino, S. N. Kosourov, L. Saari, K. Sivonen, A. A. Tsygankov, E. M. Aro and Y. Allahverdiyeva, Int. J. Hydrogen Energy, 2012, 37, 151–161 CrossRef CAS.
- M. Jämsä, S. Kosourov, V. Rissanen, M. Hakalahti, J. Pere, J. A. Ketoja, T. Tammelin and Y. Allahverdiyeva, J. Mater. Chem. A, 2018, 6, 5825–5835 RSC.
- S. N. Pawar and K. J. Edgar, Biomaterials, 2012, 33, 3279–3305 CrossRef CAS.
- R. Rippka, J. Deruelles and J. B. Waterbury, J. Gen. Microbiol., 1979, 111, 1–61 Search PubMed.
- H. K. Lichtenthaler, Methods Enzymol., 1987, 148, 350–382 CAS.
- E. S. Fry, G. W. Kattawar and R. M. Pope, Appl. Opt., 1992, 31, 2055–2065 CrossRef CAS.
- K. Mokashi, V. Shetty, S. A. George and G. Sibi, Achiev. Life Sci., 2016, 10, 111–117 Search PubMed.
- J. Li, C. Li, C. Q. Lan and D. Liao, Microb. Cell Fact., 2018, 17, 1–10 CrossRef.
- Z. Tu, L. Liu, W. Lin, Z. Xie and J. Luo, Bioresour. Technol., 2018, 266, 109–115 CrossRef CAS.
- R. Schwarz and K. Forchhammer, Microbiology, 2005, 151, 2503–2514 CrossRef CAS.
- S. Kosourov, G. Murukesan, M. Seibert and Y. Allahverdiyeva, Algal Res., 2017, 28, 253–263 CrossRef.
- T. Zavřel, H. Knoop, R. Steuer, P. R. Jones, J. Červený and M. Trtílek, Bioresour. Technol., 2016, 202, 142–151 CrossRef.
- W. Xiong, J. A. Morgan, J. Ungerer, B. Wang, P. C. Maness and J. Yu, Nat. Plants, 2015, 1, 1–36 Search PubMed.
- A. G. Miller, G. S. Espie and D. T. Canvin, Can. J. Bot., 1990, 68, 1291–1302 CrossRef CAS.
- M. R. Badger and G. D. Price, J. Exp. Bot., 2003, 54, 609–622 CrossRef CAS.
- T. C. Lee, W. Xiong, T. Paddock, D. Carrieri, I. F. Chang, H. F. Chiu, J. Ungerer, S. H. Hank Juo, P. C. Maness and J. Yu, Metab. Eng., 2015, 30, 179–189 CrossRef CAS.
- Z. Zhu, G. Luan, X. Tan, H. Zhang and X. Lu, Biotechnol. Biofuels, 2017, 10, 1–11 CrossRef.
- X. Liu, R. Miao, P. Lindberg and P. Lindblad, Energy Environ. Sci., 2019, 12, 2765–2777 RSC.
- K. Qiao, T. Takano and S. Liu, Algal Res., 2015, 9, 245–253 CrossRef.
- C. E. De Farias Silva, B. Grisa, E. Sforza, N. La and A. B. Rocca, Chem. Eng. Trans., 2016, 49, 241–246 Search PubMed.
- A. Nagarajan, M. Zhou, A. Y. Nguyen, M. Liberton, K. Kedia, T. Shi, P. Piehowski, A. Shukla, T. L. Fillmore, C. Nicora, R. D. Smith, D. W. Koppenaal, J. M. Jacobs and H. B. Pakrasi, Biomolecules, 2019, 9, 1–19 CrossRef.
- A. Karradt, J. Sobanski, J. Mattow, W. Lockau and K. Baier, J. Biol. Chem., 2008, 283, 32394–32403 CrossRef CAS.
- C. C. Zhang, C. Z. Zhou, R. L. Burnap and L. Peng, Trends Plant Sci., 2018, 23, 1116–1130 CrossRef CAS.
- A. A. Esteves-Ferreira, M. Inaba, A. Fort, W. L. Araújo and R. Sulpice, Crit. Rev. Microbiol., 2018, 44, 541–560 CrossRef CAS.
- J. A. Berges and P. G. Falkowski, Limnol. Oceanogr., 1998, 43, 129–135 CrossRef CAS.
- C. Ross, L. Santiago-Vázquez and V. Paul, Aquat. Toxicol., 2006, 78, 66–73 CrossRef CAS.
- K. D. Bidle, Curr. Biol., 2016, 26, R594–R607 CrossRef CAS.
- S. Choudhary and C. Schmidt-Dannert, Appl. Microbiol. Biotechnol., 2010, 86, 1267–1279 CrossRef CAS.
- D. T. Fedeson, P. Saake, P. Calero, P. I. Nikel and D. C. Ducat, Microb. Biotechnol., 2020, 1–15 Search PubMed.
-
S. N. Kosourov, M. He, Y. Allahverdiyeva and M. Seibert, in Microalgal Hydrogen Production: Achievements and Prospectives, ed. M. Seibert and G. Torzillo, The Royal Society of Chemistry, 2018, 16, 355–383 Search PubMed.
Footnote |
† Electronic supplementary information (ESI) available. See DOI: 10.1039/d0gc01830a |
|
This journal is © The Royal Society of Chemistry 2020 |
Click here to see how this site uses Cookies. View our privacy policy here.