DOI:
10.1039/D0GC01066A
(Paper)
Green Chem., 2020,
22, 3860-3866
High enhancement of the hydrolysis rate of cellulose after pretreatment with inorganic salt hydrates
Received
25th March 2020
, Accepted 18th May 2020
First published on 18th May 2020
Abstract
We study the use of inorganic salt hydrates as solvents in the dissolution/precipitation pretreatment of cellulose. The dissolution of cellulose was very fast (15 min in some cases) at the low temperature of 70 °C. ZnCl2·4H2O, ZnBr2·4H2O, LiCl·8H2O and LiBr·4H2O were studied as solvent. The dissolution/precipitation process dramatically modified the cellulose structure, which was completely deconstructed, as corroborated by both XRD and SEM. The nature of these salts affects cellulose dissolution. The change in cellulose morphology after dissolution/precipitation pretreatment produced an increase in the rate of hydrolysis with respect to that of untreated cellulose. The acidic catalyst employed in hydrolysis had a moderate effect on the reaction results. The best performance was obtained with H4SiW12O40 (0.05 M) at 140 °C for 300 min, where the cellulose conversion was close to 99% and the glucose yield was 90%.
Introduction
At present, the search for new sources of energy as substitutes for fossil fuels is required because fossil fuels are a finite resource. A new alternative is the use of lignocellulosic waste from energy crops because such waste offers a high potential for the production of bioproducts and biofuels that can be used as raw materials or energy sources in agricultural activities within a circular economy.1 However, due to its recalcitrance and low activity, lignocellulosic biomass must be pretreated to obtain products selectively at mild temperatures in an efficient way.2 The three main components of lignocellulosic biomass are cellulose, hemicellulose and lignin. Among them, cellulose is a very interesting feedstock, that can be transformed in compounds of high interest.3–6 Cellulose is a polymer of glucose linked by β-O-glycosidic bonds. It has inter- and intramolecular hydrogen bonds, which explains its chemical stability and its low solubility in water and in most organic solvents.7 Pretreatments have as a goal to improve the reactivity of the lignocellulosic biomass.8–12 Each pretreatment has its own effect(s) on the cellulose, hemicellulose and lignin; the three main components of lignocellulosic biomass. The pretreatments proposed in the bibliography includes physical, chemical and biological operations, recently a combination of pretreatments seems to be very effective.13–18 Interestingly, cellulose can be dissolved in ionic liquids (IL),19–22 although their high price and possible incompatibility with downstream operations limits the use of these materials to the dissolution/precipitation method,23–29 which separates the pretreatment of cellulose from the hydrolysis step. This method produces a more porous and spongy solid that has a different morphology due to the loss of crystallinity,25–27 facilitating catalyst access to β-O-glycosidic bonds and increasing the reactivity of cellulose. However, the drawback is still the possibility of loss of expensive IL; consequently, the use of an alternative solvent is necessary.
A very interesting alternative to cellulose solvents is inorganic salt hydrates.30,31 Compared with IL, inorganic salt hydrates are cheaper, recyclable, nontoxic and require lower working temperatures. The formation of these materials is simple, making them very attractive solvents. The salt hydrate intercalates between the cellulose fibers, breaking hydrogen bonds and allowing the dissolution of cellulose.32–35 The structure of salt hydrates is governed by coordination complexation between Cl− or Br− and water around Zn2+ or Li+. The proposed structure of inorganic zinc hydrate is {[Zn(H2O)6][ZnX4]·2H2O} (where X = Cl or Br).33,36 It is a complex with an octahedral structure where zinc is surrounded by 6 water molecules [Zn(H2O)6] and a tetrahedral structure where another zinc is bound to chlorine or bromine with additional lattice water molecules (Fig. 1).33,36
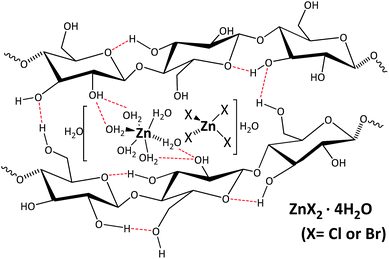 |
| Fig. 1 Proposed zinc-cellulose complex formation in cellulose treated in ZnX2·4H2O. (Adapted from33 and36). | |
The O–H⋯O hydrogen bonds between water molecules as donors and ZnCl2 tetrahedral and water molecules as acceptor groups lead to the formation of a three-dimensional network. In the case of lithium salts, water molecules surround lithium ions, the anions interact with hydrogen bonds and break them, and the water molecules dissolve the cellulose.
Cellulose is bound through strong hydrogen bonding interactions, and when the ions of the corresponding inorganic salt bind to OH groups in cellulose, the hydrogen bonds break down. The cellulose chain gains flexibility, and therefore, water molecules penetrate the network, producing the dissolution of cellulose.37
Several studies have investigated the dissolution and hydrolysis of cellulose dissolved in inorganic salt hydrates;7,38,39 however, the separation of obtained sugars from inorganic salt hydrates is very difficult due to the high solubility of sugars in the dissolution media, and despite some promising studies, such separation remains a challenge.38
The aim of this work is to study the dissolution of cellulose in different inorganic salt hydrates and its subsequent precipitation by the addition of excess water. The regenerated cellulose structure was characterized by several physicochemical techniques and finally tested in hydrolysis with inorganic acids; the results obtained were compared with those obtained using untreated cellulose.
Materials and methods
Materials and chemicals
Zinc chloride, anhydrous, 98+% was purchased from Alfa Aesar. Lithium bromide, 99+% for analysis, anhydrous and lithium chloride, 99% for analysis, anhydrous were purchased from Acros Organics. Zinc bromide, puriss, anhydrous, ≥98% was purchased from Honeywell Fluka, and Cellulose Avicel® PH-101 was purchased from Fluka. Tungstosilicic acid hydrate and phosphotungstic acid hydrate were purchased from Sigma-Aldrich, and sulfuric acid (1 M) was purchased from Scharlab.
Dissolution and precipitation
Cellulose (1.5 g) was added slowly to 28.5 g of each inorganic salt hydrate at 70 °C in a Mettler-Toledo Easy Max 102® reactor equipped with mechanical stirring. The complete dissolution of the added solute was determined by direct visual observation. Then, the material was precipitated by the addition of water (25 mL). The obtained solid was separated by vacuum filtration with a nylon membrane and was washed several times with HCl (0.1 N) and distilled water to eliminate all remaining inorganic salt hydrate.
Hydrolysis
Hydrolysis reactions were carried out batchwise in a magnetically stirred 100 mL thermostatic Teflon-lined steel Berghof reactor equipped with a pressure addition funnel. In a typical run, 0.5 g of material and 40 mL of water were mixed in the reactor, the suspension was heated to the reaction temperature (140 °C), and the appropriate amount of catalyst to obtain a concentration of 0.2 M was added. The “reaction time” was measured from this moment. The total volume of liquid in the reactor was 50 mL. Aliquots were periodically taken from the reactor. In all cases, the reaction was stopped after 5 h, and the mixture was quickly cooled. The solution was filtered off, and the solid was washed with distilled water and dried at 80 °C overnight. The amount of solid isolated was determined by weighing.
The liquid was analyzed by HPLC (Agilent Technologies HPLC 1200). The chromatographic separations were carried out in a Hi-PLEX H column at 60 °C using 0.6 mL min−1 sulfuric acid aqueous solution (0.01 M) as the mobile phase.
Characterization
X-ray diffraction profiles of samples were recorded with an X'Pert Pro PANalytical diffractometer equipped with a CuKα radiation source (λ = 0.15418 nm) and X'Celerator detector based on real-time multiple strip (RTMS) technology. The samples were ground and placed on a stainless steel plate. The diffraction patterns were recorded in steps over a range of Bragg angles (2θ) between 4° and 90° at a scanning rate of 0.02° per step and an accumulation time of 50 s. Diffractograms were analyzed with X'Pert HighScore Plus software.
Scanning electron micrographs of original and pretreated cellulose were taken with a Hitachi S-3000 N instrument. The samples were treated with increasing concentrations of ethanol to fix the structure and to dehydrate the samples. The samples were metallized in a Balzers SCD 004 gold-sputter coater.
The composition of original and recovered inorganic hydrate salts was determined by inductively coupled optical emission spectrometry (ICP-OES) using an Analytik Jena ICP-OES PlasmaQuant PQ 9000 spectrometer.
Results and discussion
Pretreatment with inorganic salts hydrates
The dissolution capacity of cellulose depends on the water content, the state of the solution and the species of the inorganic salts used.40 The molten salt hydrates reported to be able to dissolve cellulose are ZnCl2·4H2O, ZnBr2·4H2O, LiCl·8H2O, and LiBr·4H2O. These salts were chosen to be able to compare the relationship between cations with different anions and between two cations with the same anions. However, LiCl has R = 8 due to the need to add more water to form a liquid salt hydrate.7 The amount of water added to this inorganic salt has been studied in other works, which revealed that the relationship with R = 4 is an interesting option for the dissolution of lignocellulosic biomass.33
Table 1 shows the time it took the different molten salt hydrates used to dissolve the cellulose Avicel®. ZnBr2·4H2O dissolved the cellulose sample in only 15 min, being the fastest of all used, followed by LiBr·4H2O in 25 min and finally ZnCl2·4H2O in 30 min, which may indicate that bromides are better solvents and more effective than chlorides. Possibly, the larger size of the anion Br− favors the opening of the cellulose chains, causing faster dissolution. However, in the case of LiCl·8H2O, after 60 min, the cellulose was not able to dissolve, so the solution pretreatment was stopped. A possible explanation for this result is that LiCl·8H2O is twice as hydrated as the other salts, and as already described in previous works, an excess of water present in solution makes it difficult or impossible to dissolve cellulose.23,31 The amount of cellulose recovered after the pretreatment is almost quantitative for all solvent employed (Table 1).
Table 1 Dissolution time of cellulose in different hydrated ionic salts at 70 °C, proportion of cellulose recovered after precipitation and crystallinity index (CI) obtained from XRD measurements
Sample |
Dissolution time (min) |
Cellulose recovered (%) |
CI (%) |
Original Avicel PH-101 |
— |
— |
82 |
ZnCl2·4H2O |
30 |
≈ 100 |
45 |
ZnBr2·4H2O |
15 |
97 |
21 |
LiCl·8H2O |
>60 |
≈ 100 |
79 |
LiBr·4H2O |
25 |
99 |
36 |
X-ray diffraction
The XRD profiles of the samples in Fig. 2 show the morphological differences between the untreated cellulose samples and those pretreated with the different ionic salts. The dissolution treatment modified the shape of the crystal structure. The profile of untreated cellulose shows typical peaks of the crystal structure. The prominent peak at 23° shows a reflection corresponding to (200) and a peak width from 15–17°, which represents the combination of the two reflections corresponding to (110) and (1
0).41 After the process of dissolution/regeneration, the diffraction profiles of the pretreated samples exhibit a clear change: the peaks of original cellulose disappear, and we detect only a doublet at 22.0° and 20.3° that indicates a loss of the original crystallinity (cellulose type I) and the formation of an incipient type II cellulose with low crystallinity.41 However, the samples treated with LiCl·8H2O gave an XRD profile quite similar to the original, with a small decrease in intensity, which implies that the loss of crystallinity is very small. This result is consistent with the low dissolution of the cellulose sample in LiCl·8H2O.
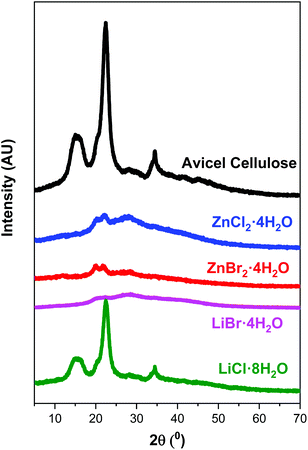 |
| Fig. 2 XRD of untreated cellulose and solids obtained after dissolution/precipitation with inorganic salt hydrates. | |
The crystallinity index has been measured according to the following expression after the normalization of the different XRD profiles. CI = (I23 − I18)/I23 where I23 and I18 are the intensities diffractogram at 23° and 18° respectively.42,43
The CI of starting cellulose is relatively high (Table 1), but the treatment of dissolution/precipitation produces a tremendous decrease in the CI, the order of CI is the following ZnBr2·4H2O < LiBr·4H2O < ZnCl2·4H2O < LiCl·8H2O. The sample treated with LiCl·8H2O has a CI similar to the starting material, as consequence of the low dissolution of the cellulose in this solvent. A lower dissolution time yields a lower CI in the cellulose recovered.
Scanning electron microscopy
The morphology of the original and pretreated cellulose samples with different inorganic salt hydrates was examined by SEM (Fig. 3). The micrographs in the upper part of the figure show the original cellulose without pretreatment. This sample contains small fragmented fibers, typical of cellulose structure, which are more apparent in the SEM images recorded at a higher magnification (right side).
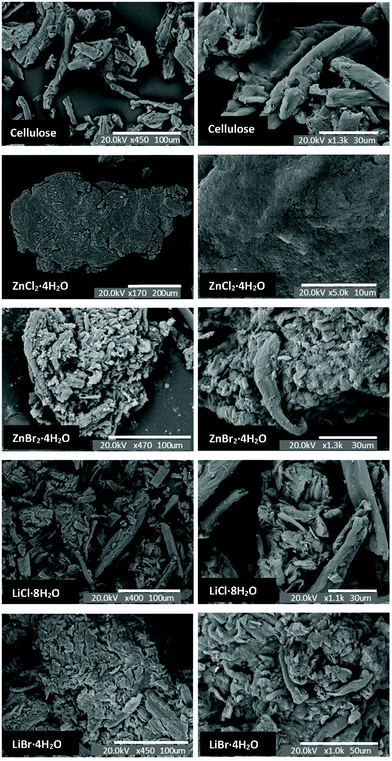 |
| Fig. 3 SEM micrographs of the original cellulose (Avicel PH-101) without pretreatment and the solids obtained after pretreatment with different inorganic salt hydrates. The left panel depicts the samples at lower magnification, and the right panel depicts the same samples with higher magnification. | |
The morphology of the cellulose samples after dissolution/precipitation with salts (ZnCl2·4H2O, ZnBr2·4H2O and LiBr·4H2O) suffers a dramatic change; the fibers disappear, and the solid material presents porous structures and spongier particles (Fig. 3). The details are clearer in the higher-magnification micrographs (on the right). However, with the salt LiCl·8H2O, the structure of cellulose observed in the SEM micrographs is quite similar to that of the starting material (Fig. 3), and the small fragmented fibers of cellulose present in the micrographs are quite similar to untreated Avicel PH-101 cellulose. This observation agrees with the XRD analysis and dissolution experiments: dissolution of the cellulose sample in LiCl·8H2O did not occur.
The changes observed in pretreated cellulose are quite similar to those observed in previous works,25,27 where pretreatment by dissolution/precipitation in ionic liquids led to a dramatic change in the structure and morphology of cellulosic substrates.
Cellulose hydrolysis
After dissolution/precipitation pretreatment, we tested the samples in a hydrolysis reaction with H2SO4 to evaluate the influence of the changes in morphology and crystallinity induced by pretreatment. For comparison purposes, we used the original cellulose as a reference. We selected the reaction conditions, a temperature of 140 °C and an acid catalyst (H2SO4) concentration of 0.2 M, based on previous works.25,27
The hydrolysis results for the celluloses pretreated with ZnCl2·4H2O, ZnBr2·4H2O and LiBr·4H2O present a higher conversion of cellulose and yield of glucose than those of the reference untreated cellulose (Table 2). As expected, the sample pretreated with LiCl·8H2O, which exhibited no dissolution crystallinity or morphology changes, the reaction results were quite similar to those of untreated samples. These results agree with the lack of changes in the structure of cellulose based on the characterization data (XRD and SEM). The reactivity trend has the opposite to the CI (Tables 1 and 2) samples with lower CI yields higher reactivity.
Table 2 Hydrolysis of cellulose in samples treated with different hydrated inorganic salts at 140 °C for 300 min
Samples treated with |
Conversion of cellulose (%) |
Yield of glucose (%) |
Selectivity of glucose (%) |
Original Avicel PH-101 |
25 |
12 |
48 |
ZnCl2·4H2O |
60 |
54 |
90 |
ZnBr2·4H2O |
88 |
80 |
91 |
LiCl·8H2O |
26 |
12 |
46 |
LiBr·4H2O |
74 |
63 |
85 |
The highest conversion and yield of glucose were obtained with pretreatment with ZnBr2·4H2O (88% and 80%, respectively), followed by the sample pretreated with LiBr2·4H2O and the sample pretreated with ZnCl2·4H2O. These results seem to be related to the dissolution time and CI. Treatment with salt hydrates that are able to dissolve cellulose quickly is more effective and reduces the CI. The salt hydrates that contain Br− anions are better solvents of cellulose, yielding a more accessible structure, which is more porous and spongier. These changes make easier to hydrolyze to glucose because access to β-O-glycosidic bonds is facilitated by changes in the structure of the cellulose.
The most efficient pretreatment was when ZnBr2·4H2O was employed as a solvent. Thus, we selected this pretreatment to compare the performance of different acidic homogeneous catalysts to study the efficiency of the hydrolysis of the β-glycosidic bonds of cellulose. The catalysts employed were heteropolyacids, which are very strong acids (H3PW12O40 and H4SiW12O40), and their performance was compared with that of H2SO4 as a reference catalyst. However, the number of protons released by these acids was different in the reaction conditions, and if we used the same concentration, the number of protons involved in the reaction would be different. In the case of H2SO4 (0.2 M), only one acidic proton should be considered (the first ionization) under the conditions employed for this hydrolysis of cellulose.25,44 However, we consider that all protons are active in the heteropolyacids; for this reason, we employed a lower concentration for these acids than sulfuric acid. For H2SO4, we used 0.2 M (1 proton), for H3PW12O40, 0.067 M (3 protons), and for H4SiW12O40, 0.05 M (4 protons).
All the catalysts exhibited a high conversion of cellulose and glucose yield in the hydrolysis of pretreated samples. The cellulose conversion ranged between 88 and 99% (Table 3), and the glucose yield ranged between 80 and 90% (Fig. 4). The main byproduct obtained was levulinic acid, which is produced by the dehydration of glucose, we detected also the presence of small amounts of 5-hydroxymethylfurfural (<3% yield) and small amounts of furfural (<0.5% yield). The yields of levulinic acid were low in all samples (Fig. 5), but the yield of levulinic acid can be related to the concentration of glucose in the reaction media. The original sample produced a low amount of glucose in dissolution, and the yield of levulinic acid was very low (<2%); however, when the samples were pretreated, the concentration of glucose in solution was high, and the levulinic acid content increased to approximately 8% at the end of the reaction in all reactions. However, we detected some differences in the levulinic acid yield among the acids employed as catalysts. The highest glucose/levulinic acid ratio was obtained for H4SiW12O40, followed by H3PW12O40 and finally H2SO4. In all cases, the amount of other by-products was very low.
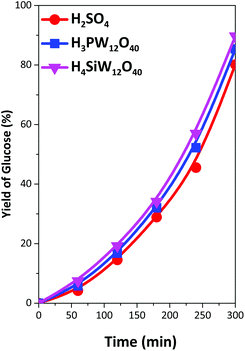 |
| Fig. 4 Yield of glucose in the hydrolysis of cellulose with H2SO4 (0.2 M), H3PW12O40 (0.067 M) and H4SiW12O40 (0.05 M) at 140 °C for cellulose treated with ZnBr2·4H2O. | |
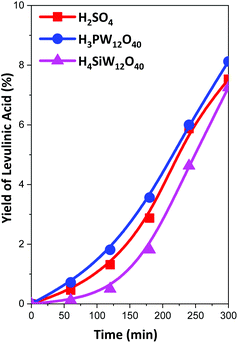 |
| Fig. 5 Yield of levulinic acid in the hydrolysis of cellulose with H2SO4 (0.2 M), H3PW12O40 (0.067 M) and H4SiW12O40 (0.05 M) at 140 °C for cellulose treated with ZnBr2·4H2O. | |
Table 3 Hydrolysis of cellulose in samples treated with ZnBr2·4H2O at 140 °C for 300 min
Catalyst |
Conversion of cellulose (%) |
H2SO4 |
88 |
H3PW12O40 |
95 |
H4SiW12O40 |
99 |
Salt hydrate reuse
Finally, we studied the reuse of ZnBr2·4H2O. Although this solvent is not expensive and has a low environmental impact, the reuse of solvents is an important point to be studied. The liquid obtained after precipitation of cellulose, filtration and washing was collected. This solution contained the salt hydrate (ZnBr2·4H2O) and an excess of water. The excess water was evaporated under reduced pressure, and the original amount of salt hydrate was almost completely recovered, the amount of salt hydrate recovered is higher than 96% (Table 4), then the salt hydrate can be reused without any additional supply of fresh salt. The recovered solvent was employed in cellulose pretreatment, and the reused salt hydrate was recovered again. The second recovery was similar to the first one, and the newly recovered salt hydrate was also employed in the pretreatment of cellulose.
Table 4 Reuse of ZnBr2·4H2O. Recovery of salt hydrate and hydrolysis of cellulose in samples treated at 140 °C for 300 min using H2SO4 (0.2 M)
Samples treated with |
ZnBr2·4H2O recovered (%) |
Conversion of cellulose (%) |
Yield of glucose (%) |
ZnBr2·4H2O fresh |
|
88 |
80 |
1st reuse |
97 |
87 |
81 |
2nd reuse |
96 |
89 |
80 |
The time required for the dissolution of cellulose was almost the same for the original salt hydrate and the once- and twice-reused solvents. The obtained pretreated cellulose was employed in hydrolysis with sulfuric acid at 140 °C. The conversion values and yield of glucose were quite similar for all three experiments, showing a high reusability of the salt hydrate.
Conclusions
Inorganic salt hydrates are good alternative solvents for cellulose, are an economical alternative to other solvents such as ionic liquids and are less noxious to the environment. In addition, the dissolution of the samples was very fast and at the low temperature of 70 °C, cellulose dissolved in 15 min. The dissolution/precipitation process dramatically modified the cellulose structure, which was completely deconstructed, as corroborated by both XRD and SEM. The nature of these salts affects cellulose dissolution. Bromide salt hydrates (ZnBr2·4H2O and LiBr·4H2O) are more efficient and dissolve cellulose faster than their chloride counterparts.
The change in cellulose morphology after dissolution/precipitation pretreatment produced an increase in the rate of hydrolysis with respect to that of untreated cellulose. The acidic catalyst employed in hydrolysis had a moderate effect on the reaction results. The best performance was obtained with H4SiW12O40 (0.05 M) at 140 °C for 300 min, where the cellulose conversion was close to 99% and the glucose yield was 90%.
Salt hydrates can be reused after the evaporation of the excess water used in the precipitation and washing of treated samples.
Conflicts of interest
The authors declare no conflicts of interest.
Acknowledgements
This research was funded by Comunidad de Madrid (Spain) and ERDF (European Regional Development Fund), grant numbers S2013/MAE-2882 (RESTOENE-2-CM), P2018/EMT-4344 (BIOTRES-CM) and CSIC (201880E029). MLS acknowledges the support of the European Social Fund and Community of Madrid for her contract. We acknowledge support of the publication fee by the CSIC Open Access Publication Support Initiative through its Unit of Information Resources for Research (URICI).
References
- B. Padrino, M. Lara-Serrano, S. Morales-delaRosa, J. M. Campos-Martín, J. L. G. Fierro, F. Martínez, J. A. Melero and D. Puyol, Front. Bioeng. Biotechnol., 2018, 6, 119 CrossRef PubMed.
- J.-P. Lange, Biofuels, Bioprod. Biorefin., 2007, 1, 39–48 CrossRef CAS.
- Y. B. Huang, T. Yang, Y. T. Lin, Y. Z. Zhu, L. C. Li and H. Pan, Green Chem., 2018, 20, 1323–1334 RSC.
- A. A. Marianou, C. C. Michailof, D. Ipsakis, K. Triantafyllidis and A. A. Lappas, Green Chem., 2019, 21, 6161–6178 RSC.
- M. Tyufekchiev, A. Kolodziejczak, P. Duan, M. Foston, K. Schmidt-Rohr and M. T. Timko, Green Chem., 2019, 21, 5541–5555 RSC.
- F. Jérôme, G. Chatel and K. D. O. Vigier, Green Chem., 2016, 18, 3903–3913 RSC.
- W. Deng, J. R. Kennedy, G. Tsilomelekis, W. Zheng and V. Nikolakis, Ind. Eng. Chem. Res., 2015, 54, 5226–5236 CrossRef CAS.
- A. T. W. M. Hendriks and G. Zeeman, Bioresour. Technol., 2009, 100, 10–18 CrossRef CAS PubMed.
-
E. Tomás-Pejó, P. Alvira, M. Ballesteros and M. J. Negro, in Biofuels, Elsevier Inc., 2011, pp. 149–176 Search PubMed.
- S. Behera, R. Arora, N. Nandhagopal and S. Kumar, Renewable Sustainable Energy Rev., 2014, 36, 91–106 CrossRef CAS.
- Y. Liao, B. O. de Beeck, K. Thielemans, T. Ennaert, J. Snelders, M. Dusselier, C. M. Courtin and B. F. Sels, Mol. Catal., 2020, 487, 110883 CrossRef CAS.
- A. Lorenci Woiciechowski, C. J. Dalmas Neto, L. Porto de Souza Vandenberghe, D. P. de Carvalho Neto, A. C. Novak Sydney, L. A. J. Letti, S. G. Karp, L. A. Zevallos Torres and C. R. Soccol, Bioresour. Technol., 2020, 304, 122848 CrossRef PubMed.
- N. Meine, R. Rinaldi and F. Schuth, ChemSusChem, 2012, 5, 1449–1454 CrossRef CAS PubMed.
- Q. Zhang and F. Jérôme, ChemSusChem, 2013, 6, 2042–2044 CrossRef CAS PubMed.
- P. Dornath, H. J. Cho, A. Paulsen, P. Dauenhauer and W. Fan, Green Chem., 2015, 17, 769–775 RSC.
- L. S. Ribeiro, J. J. M. Órfão and M. F. R. Pereira, Green Chem., 2015, 17, 2973–2980 RSC.
- F. Boissou, N. Sayoud, K. D. O. Vigier, A. Barakat, S. Marinkovic, B. Estrine and F. Jérôme, ChemSusChem, 2015, 8, 3263–3269 CrossRef CAS PubMed.
- Q. Liu, W. Li, Q. Ma, S. An, M. Li, H. Jameel and H. M. Chang, Bioresour. Technol., 2016, 211, 435–442 CrossRef CAS PubMed.
- A. Pinkert, K. N. Marsh, S. Pang and M. P. Staiger, Chem. Rev., 2009, 109, 6712–6728 CrossRef CAS PubMed.
- A. Pinkert, K. N. Marsh and S. Pang, Ind. Eng. Chem. Res., 2010, 49, 11121–11130 CrossRef CAS.
- Z. Usmani, M. Sharma, P. Gupta, Y. Karpichev, N. Gathergood, R. Bhat and V. K. Gupta, Bioresour. Technol., 2020, 304, 123003 CrossRef PubMed.
- A. Brandt, J. Gräsvik, J. P. Hallett and T. Welton, Green Chem., 2013, 15, 550–583 RSC.
- S. Morales-delaRosa, J. M. Campos-Martin and J. L. G. Fierro, Chem. Eng. J., 2012, 181–182, 538–541 CrossRef CAS.
-
S. Morales-Delarosa and J. M. Campos-Martin, in Advances in Biorefineries: Biomass and Waste Supply Chain Exploitation, ed. K. Waldron, Woodhead Publishing, 2014, pp. 152–198 Search PubMed.
- S. Morales-delaRosa, J. M. Campos-Martin and J. L. G. Fierro, ChemSusChem, 2014, 7, 3467–3475 CrossRef CAS PubMed.
- M. Lara-Serrano, F. Sáez Angulo, M. J. Negro, S. Morales-Delarosa, J. M. Campos-Martin and J. L. G. Fierro, ACS Sustainable Chem. Eng., 2018, 6, 7086–7095 CrossRef CAS.
- S. Morales-delaRosa, J. M. Campos-Martin and J. L. G. Fierro, Catal. Today, 2018, 302, 87–93 CrossRef CAS.
- M. Lara-Serrano, S. Morales-delaRosa, J. M. Campos-Martín and J. L. G. Fierro, Appl. Sci., 2019, 9, 1862 CrossRef CAS.
-
S. Morales-delaRosa, J. M. Campos-Martin and J. L. G. Fierro, WO2015/004296 (A1), 2015, asignee to CSIC.
- S. Fischer, H. Leipner, K. Thümmler, E. Brendler and J. Peters, Cellulose, 2003, 10, 227–236 CrossRef CAS.
- S. Sen, J. D. Martin and D. S. Argyropoulos, ACS Sustainable Chem. Eng., 2013, 1, 858–870 CrossRef CAS.
- X. Zhang, N. Xiao, H. Wang, C. Liu, X. Pan, S. Sen, B. P. Losey, E. E. Gordon, D. S. Argyropoulos, J. D. Martin, A. A. Awosusi, A. Ayeni, R. Adeleke, M. O. Daramola, W. Deng, J. R. Kennedy, G. Tsilomelekis, W. Zheng, V. Nikolakis, E. Hennings, H. Schmidt and W. Voigt, Ind. Eng. Chem. Res., 2016, 54, 5226–5236 Search PubMed.
- A. A. Awosusi, A. Ayeni, R. Adeleke and M. O. Daramola, J. Chem. Technol. Biotechnol., 2017, 92, 2468–2476 CrossRef CAS.
- E. Hennings, H. Schmidt and W. Voigt, Acta Crystallogr., Sect. E: Struct. Rep. Online, 2014, 70, 515–518 CrossRef CAS PubMed.
- X. Zhang, N. Xiao, H. Wang, C. Liu and X. Pan, Polymers, 2018, 10, 614 CrossRef PubMed.
- S. Sen, B. P. Losey, E. E. Gordon, D. S. Argyropoulos and J. D. Martin, J. Phys. Chem. B, 2016, 120, 1134–1141 CrossRef CAS PubMed.
- Q. Xu, C. Chen, K. Rosswurm, T. Yao and S. Janaswamy, Carbohydr. Polym., 2016, 149, 274–281 CrossRef CAS PubMed.
- Q. Liu, Q. Ma, S. Sabnis, W. Zheng, D. G. Vlachos, W. Fan, W. Li and L. Ma, Green Chem., 2019, 21, 5030–5038 RSC.
- I. Bodachivskyi, U. Kuzhiumparambil and D. B. G. Williams, Catal. Sci. Technol., 2019, 9, 4693–4701 RSC.
- Y. Cao, H. Li, Y. Zhang, J. Zhang and J. He, J. Appl. Polym. Sci., 2010, 116, 547–554 CrossRef CAS.
- A. D. French and M. Santiago Cintrón, Cellulose, 2013, 20, 583–588 CrossRef CAS.
- S. Morales-delaRosa, J. M. Campos-Martin and J. L. G. Fierro, Cellulose, 2014, 21, 2397–2407 CrossRef CAS.
- M. E. Himmel, S. Park, D. K. Johnson, J. O. Baker and P. A. Parilla, Biotechnol. Biofuels, 2010, 3, 10 CrossRef PubMed.
- C.-H. Lin, A. H. Conner and C. G. Hill, J. Appl. Polym. Sci., 1992, 45, 1811–1822 CrossRef CAS.
Footnote |
† Deceased (1948–2020) |
|
This journal is © The Royal Society of Chemistry 2020 |