DOI:
10.1039/C9FO02425E
(Paper)
Food Funct., 2020,
11, 1074-1086
Comparisons of protective effects between two sea cucumber hydrolysates against diet induced hyperuricemia and renal inflammation in mice†
Received
16th October 2019
, Accepted 12th November 2019
First published on 12th November 2019
Abstract
Hyperuricemia is an important risk factor for many diseases including hypertension and type 2 diabetes. This study investigated and compared the effects of hydrolysates of two sea cucumber species, Apostichopus japonicus and Acaudina leucoprocta, on the alleviation of diet-induced hyperuricemia and renal inflammation. The structure and abundance of oligopeptides in enzymatic hydrolysates of A. japonicus (EH-JAP) and A. leucoprocta (EH-LEU) were identified via MALDI-TOF/TOF-MS, and the anti-hyperuricemic and anti-inflammatory effects of the hydrolysates were explored in a diet-induced hyperuricemic mouse model. Both EH-JAP and EH-LEU inhibit uric acid biosynthesis and promote uric acid excretion, leading to the alleviation of the hyperuricemic phenotype. In addition, these two treatments down-regulated the transcription of pro-inflammatory cytokines, up-regulated the transcription of anti-inflammatory cytokines, and inhibited the activation of the Toll-like receptor 4/myeloid differentiation factor 88/NF-kappaB (TLR4/MyD88/NF-κB) signaling pathway, leading to the alleviation of renal inflammation. EH-JAP had better effects than EH-LEU due to differences in their regulation of uric acid biosynthesis, uric acid excretion and release of anti-inflammatory cytokines. In addition, EH-JAP and EH-LEU treatment alleviated the dysfunction of the gut microbiota by increasing the abundance of beneficial Lactobacillus and short-chain fatty acid producers and decreasing the abundance of opportunistic pathogens. This study provides a valuable reference for the development of sea cucumber applications.
Introduction
The level of uric acid in serum is mainly controlled by the balance between uric acid production in the liver and its excretion by the kidneys.1 Production of excessive uric acid by the liver beyond the renal excretion capacity leads to high levels of uric acid in serum, directly causing hyperuricemia.2 High serum levels of uric acid cause inflammatory cells to release pro-inflammatory chemokines and cytokines, increasing the risk of kidney inflammation and injury.3 Some studies reported that TLR4 activation initiates an inflammatory response by producing NF-κB-dependent inflammatory factors and that the cytosolic TLR adapter protein MyD88 is involved in this process.4 Therefore, reducing serum uric acid levels and alleviating renal inflammation are two necessary tasks in the treatment of hyperuricemia. The drugs currently used to reduce serum urate levels can be divided into three categories: drugs that inhibit uric acid synthesis (allopurinol), drugs that enhance uric acid excretion (benzbromarone) and drugs that decrease renal inflammation (cortin).5 However, due to the side effects of these drugs,6 exploring novel natural, effective and affordable anti-hyperuricemic substances with minimal side effects is highly desirable. Bioactive substances, such as peptides, unsaturated fatty acids and polysaccharides, have been found to have many biological functions, including uric acid lowering activity.7,8 Some reports showed that fucoidan and fucoxanthin can regulate the expression of uric acid transporters, such as glucose transporter type 9 (GLUT9), ATP-binding cassette subfamily G member 2 (ABCG2), organic anion transporter 1 (OAT1) and urate anion transporter 1 (URAT1) in the kidneys, thereby reducing the uric acid level in serum.9 In addition, polysaccharides from Laminaria japonica exert an anti-inflammatory effect on hyperuricemia by suppressing the activation of the NF-κB signaling pathway.10 Compared with other bioactive substances, oligopeptides often exhibit high activity, stability and specificity.6 Protein-based anti-hyperuricemic substances, such as shark cartilage water extract and milk-derived dipeptides, have been reported to regulate uric acid levels by inhibiting XOD activity.11,12 However, studies on the anti-hyperuricemic effect of oligopeptides are still in their infancy, and the mechanism through which oligopeptides reduce hyperuricemia is still unclear.13
Hyperuricemia runs in families, and multiple genetic variants of ABCG2, URAT1 and GLUT9, which are proposed to be associated with dysfunctional serum uric acid control, have been identified by genome-wide association studies.14 Diet also plays a significant role in the development of hyperuricemia. On the one hand, epidemiological studies have shown that a purine-rich diet increases the risk of developing hyperuricemia.15 On the other hand, diet has been shown to have dramatic effects on the gut microbiota and thereby to affect the host metabolism. ‘Gut microbiota’ refers to the microbes that reside inside the gut and partake in several functions beneficial to the host, including the fermentation of otherwise indigestible dietary fiber and other food components.16 Previous studies have shown that the gut microbiota was altered during the onset and alleviation of hyperuricemia. On the one hand, the increased abundance of Bacteroides caccae and decreased abundance of Bifidobacterium pseudocatenulatum were observed during the onset of hyperuricemia.17 On the other hand, the alleviation of hyperuricemia resulting from allopurinol and benzbromarone treatments increased the abundance of the genera Bifidobacterium and Collinsella and decreased that of the genera Adlercreutzia and Anaerostipes.18Bifidobacteria and Lactobacilli have been directly used as a probiotic therapy to modulate the gut microbiota and alleviate hyperuricemia.19 Therefore, the modulation of the gut microbiota needs to be taken into consideration in exploring the mechanism of anti-hyperuricemic effects.
Sea cucumbers belong to the phylum Echimodermata; nearly 1400 species of sea cucumbers are found in the world, and more than 40 species are edible.20A. japonicus is one of the most important commercial sea cucumber species in China with various beneficial functions, such as lowering blood pressure and lipid, enhancing immunity, and exhibiting antifatigue, antibacterial and antioxidant activities.21,22A. leucoprocta is another sea cucumber species. It is of low market value and its production is underdeveloped due to its poor flavor and appearance. Previous studies have confirmed that A. leucoprocta also possesses lipid-lowering, antioxidant, and anti-fatigue activities and that it improves memory.23 However, the differences in the anti-hyperuricemic and anti-inflammatory effects of these two species of sea cucumbers and the mechanisms underlying these effects remain unknown.
In this study, the structure and abundance of oligopeptides in the enzymatic hydrolysates of A. japonicus (EH-JAP) and A. leucoprocta (EH-LEU) were determined via MALDI-TOF/TOF-MS, and the anti-hyperuricemic and anti-inflammatory effects of oligopeptides were investigated and compared in a diet-induced hyperuricemic mouse model. In addition, the transcription of genes involved in uric acid metabolism, the release of chemokines and cytokines, and the regulation of inflammation-related signaling pathways was measured via qRT-PCR. Moreover, the modulation of the gut microbiota by the oligopeptides was analyzed by sequencing the V3–V4 region of the16S rRNA gene in fecal samples.
Materials and methods
Preparation of EH-JAP and EH-LEU
A. japonicus was purchased from Dalian Bangchui Island Seafood Enterprise Group Co. (Dalian, Liaoning, China), and A. leucoprocta was purchased from Ningbo Bo Wang Aquaculture Co., Ltd (Ningbo, Zhejiang, China). The enzymatic hydrolysates EH-JAP and EH-LEU were prepared according to the optimal conditions described previously,24 that is, by trypsin and alkaline protease (Yuanye Bioengineering Institute, Shanghai, China) at a ratio of 2
:
1 and a temperature of 55 °C, for 4 h; the solid–liquid ratio was 1
:
9, and the enzyme concentration was 4%. After enzymatic hydrolysis, the samples were inactivated, centrifuged, and freeze-dried.
Characterization of the structure and abundance of oligopeptides
As previously described, EH-JAP and EH-LEU were resuspended in 60 μL of acetonitrile/water (50
:
50, v/v) containing 0.2% formic acid and 2 mM sodium acetate prior to matrix-assisted laser dissociation time-of-flight mass spectrometry analysis (MALDI-TOF/TOF AB SCIEX, Applied Biosystems, Foster City, CA, USA).25 The sample was analyzed using a matrix-assisted laser desorption ionization (MALDI) mass spectrometer. The 100-fold diluted sample was mixed with an equal volume of dihydroxybenzoic acid-matrix (10 mg mL−1 of dihydroxybenzoic acid in acetonitrile/water, 50
:
50, v/v). Then, 0.5 mL of the mixture was deposited onto the MALDI target plate, and the MALDI plate was dried under vacuum to ensure uniform crystallization. The samples were collected and analyzed by MASCOT, and the structures of the oligopeptides were obtained from the NCBI library. The precise molecular weight was determined by primary mass spectrometry, and the amino acid sequence was determined by secondary mass spectrometry.26
Experimental design
All experimental procedures and animal care were performed in accordance with the Guide for the Care and Use of Laboratory Animals prepared by the Ningbo University Laboratory Animal Center (affiliated with the Zhejiang Laboratory Animal Common Service Platform), and all of the animal protocols were approved by the Ningbo University Laboratory Animal Center under permit number SYXK (ZHE 2008-0110).
ICR mice were provided by the Shanghai Slake Laboratory Animals Co., Ltd (Shanghai, China). The certificate number for the mice is SCXK ZHE 2014-0001, No. 3100200170. Food and water were available ad libitum. The animals were housed at a constant temperature of 23 ± 1 °C and a regular 12-hour light/dark cycle (lights on from 8 am to 8 pm). The mice were fed a standard chow diet (MD 17121) purchased from Mediscience Co., Ltd (Yangzhou, Jiangsu, China). After one week of acclimatization to the standard chow diet, a group of 32 7-week-old ICR mice (26 ± 2 g, male) were randomly assigned to 4 groups: a blank control group (control), a hyperuricemic model group (model), an EH-JAP treated group and an EH-LEU treated group, with eight mice per group, and the mice from each group were kept in one cage. All mice used in this study were fed the standard diet; the mice in the control group received 200 μL of saline daily for 8 weeks by gavage, and the animals in the other groups received 200 μL of high purine solution daily for 8 weeks by gavage. The purine solution contained hypoxanthine (200 mg kg−1 d−1), yeast extract (30 mg kg−1 d−1) and potassium oxalate (200 mg kg−1 d−1).27,28 The animals in the EH-JAP and EH-LEU treated groups also received 150 mg kg−1 d−1 EH-JAP and 150 mg kg−1 d−1 EH-LEU, respectively, by gavage daily for 8 weeks. The choice of dose, which was based on previous studies,29 is equivalent to a dose of 729.6 mg d−1 in a 60 kg human.30
The body weight of each animal was recorded weekly during the 8 weeks of dietary treatment. At the end of the study, fecal samples were collected from each mouse and stored at −80 °C. After this, the mice were anesthetized with isoflurane, blood samples were obtained from the eye sockets, and serum was separated via centrifugation for 15 min at 4 °C and 3000 rpm and stored at −80 °C for biochemical tests. After sample collection, the mice were sacrificed by cervical dislocation, and their visceral organs were excised, weighed, and immediately stored at −80 °C until further analysis.
Quantitative real-time PCR (qRT-PCR)
Total RNA extraction from kidney and liver tissue was performed using the TransZol Up Plus RNA kit (TransGen Biotech Co., Ltd, Beijing, China) according to the manufacturer's instructions. Isolated RNA was quantified using a Thermo NanoDrop 2000C spectrophotometer (Thermo Fisher Scientific Inc., Waltham, MA, USA), and its integrity was confirmed by agarose gel electrophoresis. After RNA extraction, equal amounts of RNA were reverse transcribed into cDNA using a High Capacity cDNA Reverse Transcription Kit (Applied Biosystems, Life Technologies, Carlsbad, CA, USA). qRT-PCR was performed with SYBR Green Master Mix and Quant Studio 6 Flex (Thermo Fisher Scientific Inc., Waltham, MA, USA) according to the protocol provided by the manufacturer. All samples were run in duplicate in a single plate, and relative quantification was performed using the 2−ΔΔCt method.31 The qRT-PCR primers used in this study are presented in ESI Table S1.† β-Actin was used as an internal control.
PCR amplification of the V3–V4 region of bacterial 16S rRNA genes
The V3–V4 region of the prokaryotic (bacterial and archaeal) small-subunit (16S) rRNA gene was amplified using the slightly modified versions of primers 338F (5′-ACTCCTACGGGAGGCAGCAG-3′) and 806R (5′-GGACTACHVGGGTWTCTAAT-3′). The 5′ ends of the primers were tagged with specific barcodes and universal sequencing primers. PCR amplification was then performed as described previously.32
The samples were sequenced on an Illumina MiSeq platform provided by LianChuan Biotech Co., Ltd (Hangzhou, Zhejiang, China) according to the manufacturer's recommendations. Paired-end reads were allocated to samples based on their unique barcodes and shortened by cutting off the barcode and primer sequences. Paired-end reads were integrated via FLASH. Quality filtering of the raw tags was performed under specific filtering conditions to obtain high-quality clean tags according to FastQC (V 0.10.1). Chimeric sequences were filtered using Verseach software (v2.3.4). Sequences with ≥97% similarity were assigned to the same operational taxonomic units (OTUs) via Verseach (v2.3.4). Information on the abundance of OTUs was normalized using a standard sequence length corresponding to that of the samples with the shortest sequences. Alpha and beta diversity analyses were performed using QIIME (Version 1.8.0). The OTUs, which were identified by redundancy analysis (RDA), were heat map analyzed using HemI software (version 1.0.3.3). An RDA model was established to determine the abundance of OTUs in animals that received high purine diets and EH-JAP or EH-LEU enzymatic hydrolysates. The relative abundance of each OTU was standardized prior to the establishment of the RDA model using Canaco (Microcomputer Power, Ithaca, NY, USA) according to the manufacturer's instructions. The correlation of the gut microbiota composition with hyperuricemic phenotype and renal inflammation was calculated by Spearman's correlation analysis in SPSS (version 19.0; Chicago, IL, USA) with Spearman correlation coefficients (R) and P values. The type of treatment was used as an enviromental variable. When p < 0.05 and the false discovery rate <0.25, the correlation was considered significant.
Measurement of short-chain fatty acids (SCFAs) by GC-MS
Mice fecal samples were prepared and subsequently analyzed using gas chromatography as previously described.33 Briefly, the samples were weighed (30 ± 1 mg) and an extraction solution (2 ml) containing water (0.8 ml), 50% H2SO4 (0.2 ml) and ether (1 ml) were added. The solution was subsequently extracted for 15 min on a horizontal shaker at 4 °C and centrifuged for 10 min at 15
000g at 4 °C. After this CaCl2 was added to the supernatant to absorb the water, and a 0.22 μm filter was used before the supernatant was injected into the gas chromatography-mass spectrometric system. Concentrations of the SCFAs were measured using an Agilent-7890 gas chromatography system (Agilent Technologies Inc., Palo Alto, America) coupled to a M7-80Ei mass spectrometric detector (Beijing Purkinje General Instrument Co., Ltd, Beijing, China).
Statistical analysis
All data are presented as the mean ± standard deviation (SD). All normally distributed datasets were analyzed via one-way analysis of variance (ANOVA) and Tukey's post hoc test (SPSS) and when the distribution of the data did not conform to the Gaussian model of heterogeneity, non-parametric Kruskal–Wallis analysis was performed.34 Differences were considered statistically significant when p < 0.05.
Accession number
The GM sequence data were deposited in the NCBI Sequence Read Archive Database under accession number SRP224124.
Results
Oligopeptide composition of EH-JAP and EH-LEU
The amino acid sequences of oligopeptides in EH-JAP and EH-LEU were determined via MALDI-TOF/TOF-MS; peaks at 630 and 483 in the m/z range 0–2000 were detected in EH-JAP and EH-LEU, respectively (ESI Fig. S1 and S2†). GPSGRP (Gly-Pro-Ser-Gly-Arg-Pro) and GPAGPR (Gly-Pro-Ala-Gly-Pro-Arg) were identified as the major oligopeptides in EH-JAP, whereas PQGETGA (Pro-Gln-Gly-Glu-Thr-Gly-Ala) and GFDGPEGPR (Gly-Phe-Asp-Gly-Pro-Glu-Gly-Pro-Arg) were detected with the highest abundance in EH-LEU. Detailed information on the ten oligopeptides that were present in the highest abundance in EH-JAP and EH-LEU is provided in Tables 1 and 2, respectively.
Table 1 Structure and abundance of oligopeptides in EH-JAP
Polypeptide sequence |
Polypeptide expected Mr |
Polypeptide calculated Mr |
Polypeptide score |
Relative intensity (%) |
Protein description |
GPSGRP |
569.3978 |
569.2922 |
14.00 |
4.04 |
Collagen |
GPAGPR |
553.4009 |
553.2972 |
25.48 |
2.79 |
Collagen |
NGVIPR |
654.4662 |
654.3813 |
9.13 |
1.02 |
Major yolk protein 2 |
IPELE |
599.4081 |
599.3166 |
12.68 |
0.72 |
Myc |
PQGETGA |
658.3787 |
658.2922 |
12.45 |
0.70 |
Collagen |
PGFGIISHV |
925.5594 |
925.5022 |
3.52 |
0.67 |
Cytochrome oxidase subunit I, (mitochondrion) |
PGKPGE |
583.4103 |
583.2966 |
19.06 |
0.67 |
Wnt16 |
GLTGAP |
514.3555 |
514.2751 |
3.30 |
0.62 |
Collagen |
ATGPR |
500.3630 |
500.2707 |
9.34 |
0.61 |
Collagen |
VPSLGVKMDAVPGR |
1424.8389 |
1424.7810 |
7.76 |
0.60 |
Cytochrome c oxidase subunit II (mitochondrion) |
Table 2 Structure and abundance of oligopeptides in EH-LEU
Polypeptide sequence |
Polypeptide expected Mr |
Polypeptide calculated Mr |
Polypeptide score |
Relative intensity (%) |
Protein description |
PQGETGA |
658.3945 |
658.2922 |
14.83 |
2.37 |
Collagen |
GFDGPEGPR |
930.5654 |
930.4196 |
43.92 |
2.04 |
Collagen |
GPAGPR |
553.4070 |
553.2972 |
21.18 |
1.82 |
Collagen |
NGVIPR |
654.4775 |
654.3813 |
10.34 |
1.81 |
Major yolk protein 2 |
PAGAPGSPGVAGER |
1221.7508 |
1221.6102 |
10.32 |
1.19 |
Collagen |
GNIGIP |
569.4039 |
569.3173 |
7.09 |
0.96 |
Collagen |
LKISAP |
627.4270 |
627.3955 |
11.43 |
0.86 |
Voltage-dependent calcium channel beta subunit |
PLGSLVS |
671.5039 |
671.3854 |
6.01 |
0.61 |
Aldehyde dehydrogenase 7A1 |
SSAVMALQG |
878.5629 |
878.4168 |
6.42 |
0.59 |
Histone H3, partial |
IGIPGPR |
708.5181 |
708.4283 |
3.42 |
0.58 |
Collagen |
Effect of EH-JAP and EH-LEU on physiological indexes of hyperuricemic mice
There were no significant differences in the body weights of the animals in any of the groups (p > 0.05) (Fig. 1A). Compared with the control group, the indexes of the liver, kidneys and spleen were increased in the model group; these indexes were restored to normal in the EH-JAP and EH-LEU groups and were not significantly different in these two groups (p > 0.05) (Fig. 1B–D).
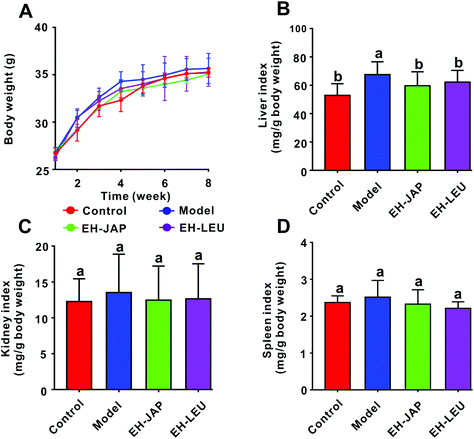 |
| Fig. 1 Effects of EH-JAP and EH-LEU on physiological indexes of hyperuricemic mice. (A) Body weight, (B) liver index, (C) kidney index, and (D) spleen index. Data are expressed as mean ± SD; n = 8. Different letters correspond to statistically significant differences (p < 0.05) between groups. | |
Effect of EH-JAP and EH-LEU on biochemical indexes of hyperuricemic mice
As shown in Fig. 2A–C, the levels of serum uric acid (SUA), blood urea nitrogen (BUN) and serum creatinine (SCR) in the model group were significantly increased compared with those in the control group. Compared to the model group, EH-JAP treatment significantly decreased SUA, BUN and SCR levels by 41.04% (192.01 ± 15.99 μmol L−1), 40.22% (6.51 ± 0.49 μmol L−1) and 39.73% (40.03 ± 7.28 μmol L−1), respectively. The corresponding reductions in the EH-LEU group were 39.95% (195.54 ± 20.95 μmol L−1), 8.3% (9.97 ± 1.53 μmol L−1) and 29.31% (46.95 ± 8.26 μmol L−1) (p < 0.05). Compared to the EH-LEU group, the serum related biochemical indexes in the EH-JAP group were closer to those in the control group.
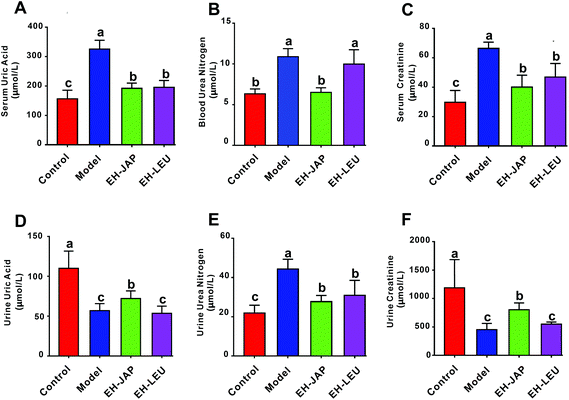 |
| Fig. 2 Effects of EH-JAP and EH-LEU on biochemical indexes of hyperuricemic mice. (A) Serum uric acid, (B) blood urea nitrogen, (C) serum creatinine, (D) urine uric acid, (E) urine urea nitrogen, and (F) urine creatinine. Data are expressed as mean ± SD; n = 8. Different letters correspond to statistically significant differences (p < 0.05) between groups. | |
In addition, as shown in Fig. 2D–F, the urine urea nitrogen (UUN) level was significantly enhanced in the model group, while urine uric acid (UUA) and urine creatinine (UCR) levels decreased compared with those in the control group (p < 0.05). After EH-JAP treatment, UUA and UCR levels increased significantly by 25.42% (72.15 ± 9.05 μmol L−1) and 77.53% (802.67 ± 107.14 μmol L−1), respectively, and the UUN level decreased significantly by 37.59% (27.69 ± 2.84 μmol L−1). After EH-LEU supplementation, UUA and UCR levels increased by 4.63% (59.56 ± 8.42 μmol L−1) and 77.53% (802.67 ± 107.14 μmol L−1), respectively, and the UUN level was significantly reduced by 30.31% (30.92 ± 6.83 μmol L−1). Compared to the EH-LEU group, the urine-related biochemical indexes in the EH-JAP group were closer to those in the control group.
EH-JAP and EH-LEU suppress uric acid biosynthesis in hyperuricemic mice
The liver is the primary site of uric acid biosynthesis. Phosphate ribose pyrophosphate synthetase (PRPS), purine nucleoside phosphorylase (PNP) and xanthine oxidase (XOD), which catalyze the formation of uric acid from adenosine monophosphate by tandem catalysis, are the key enzymes in this process. Compared to the control group, the transcription levels of PRPS, PNP and XOD were significantly up-regulated in the model group; in animals that received EH-JAP or EH-LEU supplementation, the transcription levels of these three genes were restored to normal, with no significant difference between the EH-JAP and EH-LEU groups (Fig. 3).
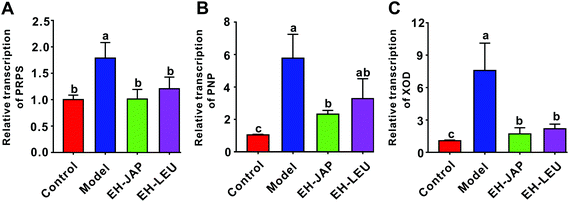 |
| Fig. 3 EH-JAP and EH-LEU suppress the transcription of genes involved in the urate biosynthesis pathway. The mRNA expression of urate biosynthesis genes was evaluated by RT-PCR. (A) PRPS, (B) PNP, and (C) XOD. The data are expressed as mean ± SD, n = 8. Different letters correspond to statistically significant differences (p < 0.05) between groups. | |
EH-JAP and EH-LEU promote uric acid excretion in hyperuricemic mice
Uric acid transporters include urate absorptive transporters and urate secretory transporters. The former, which include organic anion transporter 4 (OAT4), GLUT9 and URAT1, reabsorb urate across the apical membrane and promote the entry of urate into the cell. The latter, which include ABCG2, multidrug resistance protein 4 (MRP4) and OAT1, transport urate from the epithelial cell to the apical membrane. Compared to the control group, the expression of urate absorptive transporters (OAT4, GLUT9 and URAT1) was significantly up-regulated in the model group, whereas the expression of urate secretory transporters (ABCG2, MRP4 and OAT1) was significantly down-regulated (Fig. 4). However, in animals that received EH-JAP or EH-LEU supplementation, the transcription levels of urate absorptive and secretory transporters were restored compared with those in the model group.
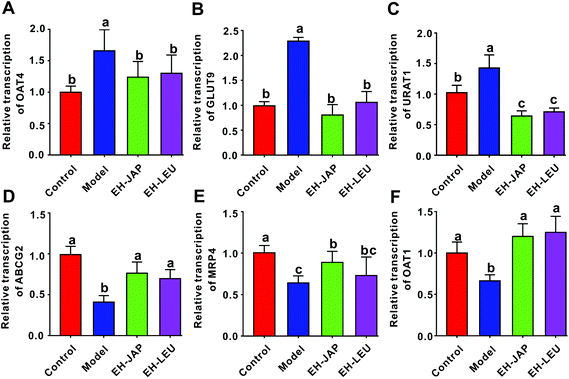 |
| Fig. 4 EH-JAP and EH-LEU suppress the transcription of genes encoding urate absorptive transporters and activate urate secretory transporters. The mRNA expressions of genes encoding urate transport-related transporters were evaluated by RT-PCR. (A) OAT4, (B) GLUT9, (C) URAT1, (D) ABCG2, (E) MRP4, and (F) OAT1. The data were expressed as mean ± SD, n = 8. Different letters correspond to statistically significant differences (p < 0.05) between groups. | |
EH-JAP and EH-LEU inhibit the activation of the TLR4/MyD88/NF-κB signaling pathway in hyperuricemic mice
The renal transcription levels of inflammatory cytokines, including the pro-inflammatory cytokines IL-1β, TNF-α and IL-6 and the anti-inflammatory cytokines transforming growth factor β (TGF-β) and IL-10, were examined. Compared to the control group, the transcription levels of pro-inflammatory cytokines were significantly up-regulated in the model group (Fig. 5A–C), whereas those of anti-inflammatory cytokines were significantly down-regulated (Fig. 5D and E). After treatment with EH-JAP and EH-LEU, the transcription of these inflammatory genes was restored compared with the model group. Compared to the EH-LEU group, the EH-JAP-treated group showed a better effect on the restoration of the transcription levels of these inflammatory genes.
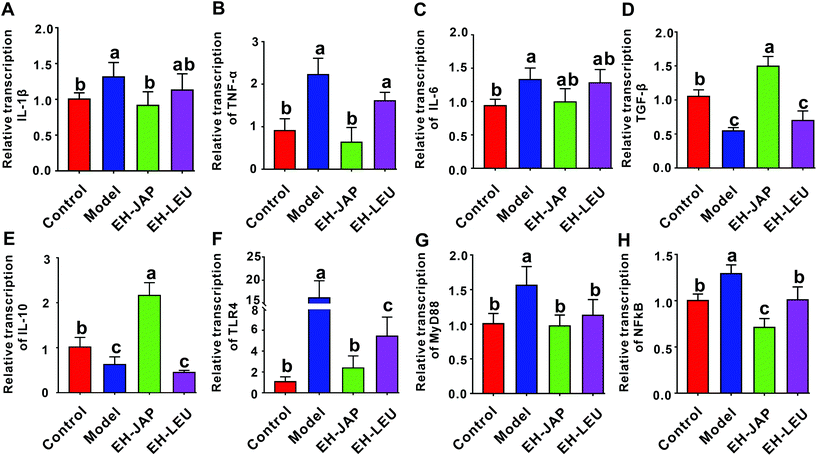 |
| Fig. 5 Regulation of inflammatory cytokines by EH-JAP and EH-LEU. The mRNA expression of inflammatory cytokines was evaluated by RT-PCR. (A) IL-1β, (B) TNF-α, (C) IL-6, (D) TGF-β, (E) IL-10, (F) TLR4, (H) MyD88, and (G) NF-κB. The data are expressed as mean ± SD; n = 8. Different letters correspond to statistically significant differences (p < 0.05) between groups. | |
The TLR4/MyD88/NF-κB signaling pathway plays an essential role in the inflammatory response. The transcription levels of TLR4, MyD88 and NF-κB were significantly up-regulated in the model group compared with those in the control group, whereas both EH-JAP and EH-LEU treatment significantly down-regulated the transcription of all these genes. Similarly, EH-JAP treatment showed a better effect on suppressing the activation of the TLR4/MyD88/NF-κB signaling pathway compared to EH-LEU treatment (Fig. 5F–H).
Effect of EH-JAP and EH-LEU on the modulation of the gut microbiota in hyperuricemic mice
We compared the gut microbial communities of mice in the experimental groups and the control group by sequencing the V3–V4 regions of the 16S rRNA gene. To appraise the species richness and diversity of the gut microbiota, the Chao1 index and the Shannon index, respectively, were calculated (ESI Table S2†). Compared to the control group, species richness and diversity were significantly decreased in the model group. EH-JAP and EH-LEU treatment increased both species richness and diversity compared to the model group, and relatively higher species richness and diversity were observed in the EH-JAP group (Fig. 6A and B).
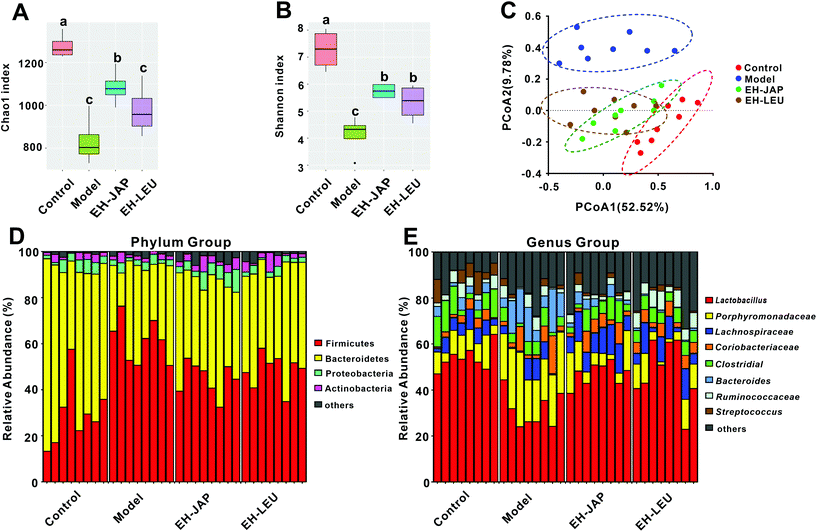 |
| Fig. 6 Effects of EH-JAP and EH-LEU on the modulation of the gut microbiota structure in hyperuricemic mice. (A) Chao1 index, (B) Shannon index, (C) principal coordinate analysis (PCoA) of the gut microbial communities, and (D) RDP classification at the phylum level. (E) RDP classification at the genus level. | |
To measure possible differences in the gut microbiota structure, principal coordinates analysis (PCoA) was performed based on the weighted (Fig. 6C) and unweighted (ESI Fig. S3†) UniFrac distances for the evaluation of the community composition. The result showed that treatment with EH-JAP or EH-LEU shifts the gut microbiota structure from that of the model group to that of the control group; different gut microbiota structures were also observed in the EH-JAP or EH-LEU groups. As shown in Fig. 6D, Firmicutes, Bacteroidetes, Proteobacteria and Actinobacteria were the four dominant bacterial phyla in all groups. Compared with the control group, the relative abundance of Firmicutes and Actinobacteria was significantly increased in the model group, while that of Bacteroidetes and Proteobacteria was significantly decreased. Compared with the model group, the EH-JAP and EH-LEU groups restored the relative abundance of Bacteroidetes and Firmicutes. However, the abundance of Actinobacteria and Proteobacteria was only restored in the EH-JAP group, while further aggravated in the EH-LEU group (Fig. 6D and ESI Table S3†).
The genera with high abundance identified in all groups are shown in Fig. 6E. Compared with the control group, the relative abundance of Porphyromonadaceae, Coriobacteriaceae and Bacteroides increased in the model group, while that of Lactobacillus, Lachnospiraceae, Ruminococcaceae, Clostridiales and Streptococcus decreased. In animals that received EH-JAP or EH-LEU supplementation, the relative abundance of Lactobacillus, Clostridiales, Porphyromonadaceae, Lachnospiraceae and Bacteroides was restored, whereas Coriobacteriaceae was restored only in the EH-JAP group, and Streptococcus and Ruminococcaceae were restored only in the EH-LEU group. Furthermore, the relative abundance of Streptococcus and Ruminococcaceae in the EH-JAP group was further decreased (Fig. 6E and ESI Table S4†).
Key phylotypes of the gut microbiota responding to EH-JAP and EH-LEU in hyperuricemic mice
The key OTUs were identified via RDA. Compared to the model group, 108, 85 and 83 key OTUs were identified in the control, EH-JAP and EH-LEU groups, respectively, and 67 key OTUs were shared by all the groups (ESI Fig. S4 and Table S5†). Among these 67 OTUs, 46 key OTUs were up-regulated and 21 key OTUs were down-regulated in the model group compared with the control group. Subsequently, 49 and 53 OTUs were restored by EH-JAP and EH-LEU treatment, respectively, and 37 OTUs were restored by both treatments. Among the 37 OTUs that were restored by both EH-JAP and EH-LEU, 9, 6 and 5 belong to the families Bacteroidaceae, Porphyromonadaceae and Lactobacillaceae, respectively. Twelve OTUs were restored only by EH-JAP treatment; 4 and 3 of these OTUs belong to the families Lactobacillus and Lachnospiraceae, respectively. Sixteen OTUs were only restored in the EH-LEU group; 11 of these OTUs belong to the family Porphyromonadaceae (Fig. 7B). The results indicate that JAP and LEU have different regulatory effects on the gut microbiota.
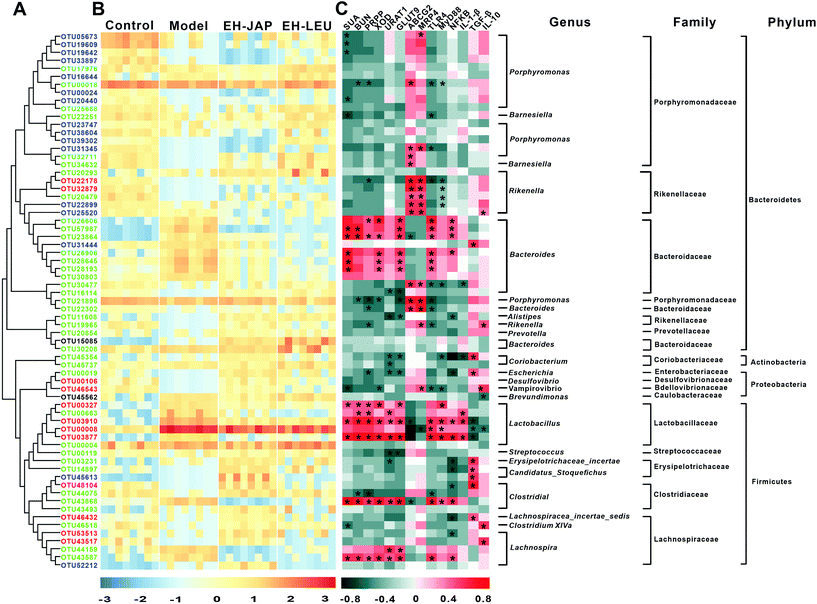 |
| Fig. 7 Key OTUs that responded to EH-JAP and EH-LEU treatment identified via redundancy analysis. (A) Phylogenetic tree of the gut microbiota. To study the phylogenetic relationship, we constructed a phylogenetic tree by comparing the base differences in the OTU sequence with the species annotation information for each OTU sequence at a defined classification level. Red color indicates OTUs that were restored in the EH-JAP group, blue color indicates OTUs that were restored in the EH-LEU group, green color indicates OTUs that were restored in both groups, and black color indicates OTUs that were not restored in either the EH-JAP or the EH-LEU group. (B) Heatmap of the relative abundance of gut bacterial genera. Rows correspond to operational taxonomic units (OTUs), and columns represent the animals in the 4 different diet groups. (C) Spearman's correlation analysis of 67 OTUs with SUA and BUN in the serum, PRPS and XOD in the liver, and URAT1, GLUT9, ABCG2, MRP4, TLR4, MyD88, NFκB, IL-6, TGF-β and IL-10 in the kidneys. Red color indicates a positive correlation, and green color indicates a negative correlation. *p < 0.05. The taxonomy of the OTUs (genus, family and phylum) is described on the right. | |
Spearman's correlation analysis was performed to correlate the 67 OTUs with hyperuricemic and inflammation-related indexes. The former include biochemical indexes (SUA and BUN) and urate biosynthesis-related genes (PRPS and XOD) and uric acid transporters (URAT1, GLUT9, ABCG2 and MRP4), while the latter include signaling pathway genes (TLR4, MyD88 and NF-κB) and genes related to inflammation (IL-1β, TGF-β and IL-10). Forty-two OTUs were significantly correlated with the hyperuricemic phenotype. Of these, 28 negatively correlated OTUs belong to the families Porphyromonadaceae (n = 10) and Rikenellaceae (n = 7), whereas 14 positively correlated OTUs belong to the families Bacteroidaceae (n = 6) and Lactobacillaceae (n = 5). In addition, 40 OTUs were significantly correlated with inflammation phenotypes. Of these, 26 were negatively correlated OTUs, most belonging to the families Porphyromonadaceae (n = 4), Rikenellaceae (n = 6), Erysipelotrichaceae (n = 3) and Lachnospiraceae (n = 2); 14 positively correlated OTUs were found, most of which belong to the families Bacteroidaceae (n = 6) and Lactobacillaceae (n = 5). Overall, the abundances of microorganisms in the families Porphyromonadaceae, Rikenellaceae, Bacteroidaceae and Lactobacillaceae may be important in hyperuricemia and in the alleviation of the associated renal inflammation. Furthermore, 32 OTUs were found to be correlated with both the hyperuricemic and inflammation phenotypes.
Effect of EH-JAP and EH-LEU on the concentrations of SCFAs in hyperuricemic mice
The concentrations of four SCFAs, acetic acid, propionic acid, butyric acid and valeric acid, were measured by GC-MS in this study (Fig. 8). Compared to the control group, the concentrations of propionic acid and butyric acid in the model group were significantly increased. After EH-JAP treatment, their concentrations increased by 6.76% (5.21 ± 0.12 μmol g−1, p > 0.05) and 17.96% (6.83 ± 0.09 μmol g−1, p < 0.05), respectively. However, EH-LEU supplementation significantly decreased the concentrations of propionic acid and butyric acid by 22.30% (3.98 ± 0.04 μmol g−1) and 17.78% (4.77 ± 0.03 μmol g−1), respectively. In addition, there were no significant differences in the concentrations of acetic acid and valeric acid among all four groups (p > 0.05).
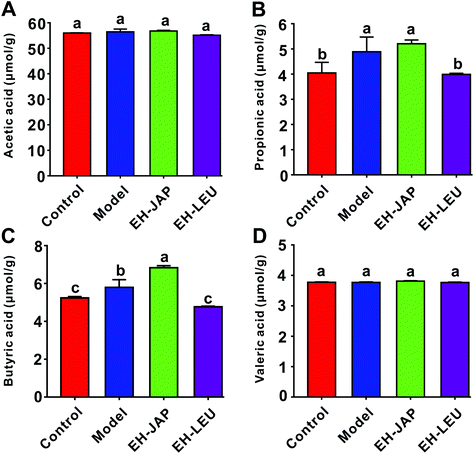 |
| Fig. 8 Effect of EH-JAP and EH-LEU on the concentrations of SCFAs in hyperuricemic mice. The concentrations of (A) acetic acid, (B) propionic acid, (C) butyric acid and (D) valeric acid. Data are expressed as mean ± SD; n = 8. Different letters correspond to statistically significant differences (p < 0.05) between groups. | |
Discussion
Hyperuricemia occurs as a result of uric acid overproduction, renal underexcretion, or a combination of the two.35 Because uric acid is the end product of purine metabolism, avoidance of purine-rich foods such as red meat is commonly recommended to hyperuricemic patients.15 In addition, long-term fructose and alcohol administration were reported to suppress the renal excretion of uric acid, resulting in elevated serum uric acid levels.15 Multiple enzymes, including PRPS, PNP and XOD, participate in uric acid biosynthesis. These enzymes play important roles in the synthesis of uric acid through a series of tandem catalysis reactions. PRPS acts at one of the earliest steps of uric acid biosynthesis, in which phosphoribosyl pyrophosphate is formed through the phosphoribosylation of ribose-5-phosphate.36 PNP is a key enzyme in the purine salvage pathway, which allows cells to utilize existing bases and nucleosides to synthesize nucleotides. It has been reported that ulodesine, a new anti-hyperuricemic drug, relieves hyperuricemia by inhibiting PNP activity, thereby reducing the accumulation of hypoxanthine and xanthine. XOD plays an important role in uric acid biosynthesis by converting hypoxanthine to uric acid. Various anti-hyperuricemic substances, such as anthocyanins, tuna protein hydrolysates and Grifola frondosa, have shown inhibition effects on XOD activity.37–39 In this study, EH-JAP and EH-LEU treatment significantly decreased serum uric acid levels as well as the mRNA levels of PRPS, PNP and XOD in hyperuricemic mice, suggesting that EH-JAP and EH-LEU inhibit uric acid production by affecting multiple targets (Fig. 3). In healthy humans, the production and excretion of uric acid are in balance. Uric acid is excreted mainly via the kidneys (70% of the total) and the gastrointestinal tract (30% of the total).40 Almost all uric acid is filtered by the renal glomeruli, and post-glomerular reabsorption mediated by GLUT9, URAT1 and OAT4 and secretion mediated by MRP4, ABCG2 and OAT1 regulate the amount of uric acid excretion.14 Our results indicated that uric acid excretion was inhibited in the model group by the promotion of uric acid reabsorption and reduction of uric acid secretion in the kidneys. These stimulatory effects were restored by treatment with EH-JAP and EH-LEU. EH-JAP showed better efficiency than EH-LEU in uric acid biosynthesis inhibition and promotion of uric acid excretion, while there was no significant difference in the effects of EH-JAP and EH-LEU on uric acid reabsorption (Fig. 4A–C). This may be one reason for the better anti-hyperuricemic effect observed in the EH-JAP group.
The inflammatory response is a characteristic pathologic feature of hyperuricemia.41 It is reported that hyperuricemia promotes the expression of pro-inflammatory cytokines and chemokines in the kidneys, such as cytokines IL-1β, TNF-α, and IL-6, which will induce renal inflammation and further induce kidney injury.3 Among them, IL-1β, also known as an endogenous pyrogen, is a highly inflammatory cytokine that participates in neutrophil chemotaxis and influx into the site of inflammation.42 TNF-α and IL-6 also participate in the inflammatory response and promote the proliferation of glomerular mesangial cells and the mesangial matrix.43 On the other hand, unlike pro-inflammatory cytokines, TGF-β and IL-10 are recognized as the prime anti-inflammatory cytokines in the resolution of acute hyperuricemia. TGF-β is a powerful modulator of inflammation, and it has been implicated in the regulation of leukocyte and vascular endothelial cell activation,44 while IL-10 inhibits TNF-α action by inducing macrophage production and macrophage inflammatory proteins (MIP-1α and MIP-1β) in response to hyperuricemia.45 Previous studies have shown that the expression of the pro-inflammatory cytokine IL-1β is regulated by the NF-κB pathway, in which TLR4 signaling and the cytosolic TLR adapter protein MyD88 are involved.46 In this study, both EH-JAP and EH-LEU inhibited the activation of the TLR4/MyD88/NF-κB signaling pathway, down-regulated the transcription of the pro-inflammatory cytokines IL-1β, TNF-α and IL-6 and up-regulated the transcription of the anti-inflammatory cytokine TGF-β, thereby relieving renal inflammation. However, the transcription of IL-10, a potent anti-inflammatory cytokine, was up-regulated only in EH-JAP-treated mice and was further decreased in EH-LEU treated mice, which may have led to a relatively poor anti-inflammatory effect after EH-LEU treatment (Fig. 5).
A potential role of the gut microbiome in metabolic diseases has recently been revealed.47 Lactobacillus, which is widely distributed in the gut, has been shown to have anti-hyperuricemic effects. On the one hand, Lactobacillus synthesizes uricase, allantoinase and allantoicase, and these three enzymes can sequentially degrade uric acid to 5-hydroxyisourate, allantoin and allantoate and eventually to urea.48 On the other hand, Lactobacillus decreases the absorption of purines by the human gut, preventing increased serum uric acid levels and further hyperuricemia.49 In this study, compared with the model group, the abundance of Lactobacillus was significantly increased in the EH-JAP and EH-LEU groups. Clostridiales and Ruminococcaceae, which are known to produce SCFAs,50 were also detected in this study. SCFAs are organic fatty acids that contain fewer than six carbon atoms; previous studies have confirmed that SCFAs, especially propionic acid and butyric acid, provide energy in the form of ATP for the excretion of uric acid by the cells in the intestinal wall.51 Compared with the model group, the abundance of Clostridiales was significantly increased in both the EH-JAP group and the EH-LEU group, while Ruminococcaceae was uniquely increased in the EH-LEU group. The relative abundances of other families of bacteria, including the members of Coriobacteriaceae, Porphyromonadaceae and Bacteroides, were also changed after EH-JAP and EH-LEU treatment. The family Coriobacteriaceae has been linked to host lipid metabolism and is associated with dyslipidemic phenotypes.52 Both Porphyromonadaceae and Bacteroides are believed to be opportunistic pathogens in the human gut,18 and Porphyromonadaceae is related to the onset of ankylosing spondylitis and Crohn's disease.53 In this study, Bacteroides and Porphyromonadaceae were decreased in both the EH-JAP and EH-LEU groups, while Coriobacteriaceae was uniquely decreased in the EH-JAP group (Fig. 6E). The observed alterations in the gut microbiota are proposed to be one of the mechanisms through which hyperuricemia is relieved by the EH-JAP and EH-LEU treatment. However, whether the beneficial effects of EH-JAP and EH-LEU are mediated by changes in the gut microbiota should be further explored via fecal microbiota transplantation.
Conclusion
In conclusion, our results demonstrate that both EH-JAP and EH-LEU alleviate hyperuricemia and relieve renal inflammation in mice with diet-induced hyperuricemia. Relatively better effects were observed after treatment with EH-JAP. Both EH-JAP and EH-LEU inhibit uric acid biosynthesis, promote uric acid excretion and suppress inflammatory cytokine expression via inhibition of the activation of the TLR4/MyD88/NFκB signaling pathway. In addition, EH-JAP and EH-LEU were found to reverse the dysfunction in the gut microbiota in hyperuricemic mice, increasing the abundance of beneficial bacteria such as probiotics (Lactobacillus) and SCFA producers (Clostridiales) and decreasing that of opportunistic pathogens such as Porphyromonadaceae and Bacteroides. On the other hand, inconsistent changes in Coriobacteriaceae and Ruminococcaceae were observed in the two groups. Whether or not the anti-hyperuricemic and anti-inflammatory effects of these extracts are mediated by the gut microbiota will require further studies.
Conflicts of interest
All authors declare that they have no conflict of interest.
Acknowledgements
This work was sponsored by the National Key R&D Program of China (2018YFD0901102), the Natural Science Foundation of Zhejiang Province (LY18C010001 and LY19C010003), the Public Welfare Project of Ningbo city (2019C10064), and the K. C. Wong Magna Fund in Ningbo University.
References
- N. Anzai, A. Enomoto and H. Endou, Renal urate handling: clinical relevance of recent advances, Curr. Rheumatol. Rep., 2005, 7(3), 227–234 CrossRef CAS.
- Y. Ye, Y. Zhang, B. Wang, W. Walana, J. Wei, J. R. Gordon and F. Li, CXCR1/CXCR2 antagonist G31P inhibits nephritis in a mouse model of uric acid nephropathy, Biomed. Pharmacother., 2018, 107, 1142–1150 CrossRef CAS.
- Y. Zhou, L. Fang, L. Jiang, P. Wen, H. Cao, W. He, C. Dai and J. Yang, Uric Acid Induces Renal Inflammation via Activating Tubular NF-κB Signaling Pathway, PLoS One, 2012, e39738 CrossRef CAS.
- J. V. Bonventre and L. Yang, Cellular pathophysiology of ischemic acute kidney injury, J. Clin. Invest., 2011, 4210–4221 CrossRef CAS.
- T. Pascart and F. Liote, Gout: state of the art after a decade of developments, Rheumatology, 2019, 58(1), 27–44 Search PubMed.
- N. Liu, Y. Wang, M. Yang, W. Bian, L. Zeng, S. Yin, Z. Xiong, Y. Hu, S. Wang, B. Meng, J. Sun and X. Yang, New Rice-Derived Short Peptide Potently Alleviated Hyperuricemia Induced by Potassium Oxonate in Rats, J. Agric. Food Chem., 2019, 67(1), 220–228 CrossRef CAS.
- P. Yu and H. Gu, Bioactive substances from marine fishes, shrimps, and algae and their functions: present and future, Crit. Rev. Food Sci. Nutr., 2015, 55(8), 1114–1136 CrossRef CAS.
- T. T. Zhang, J. Xu, Y. M. Wang and C. H. Xue, Health benefits of dietary marine DHA/EPA-enriched glycerophospholipids, Prog. Lipid Res., 2019, 75, 100997 CrossRef CAS.
- Y. T. Chau, H. Y. Chen, P. H. Lin and A. S. Hsia, Preventive Effects of Fucoidan and Fucoxanthin on Hyperuricemic Rats Induced by Potassium Oxonate, Mar. Drugs, 2019, 17(6), 343 CrossRef CAS.
- D. Zhang, H. Liu, P. Luo and Y. Li, Production Inhibition and Excretion Promotion of Urate by Fucoidan from Laminaria japonica in Adenine-Induced Hyperuricemic Mice, Mar. Drugs, 2018, 16(12), 472 CrossRef CAS.
- I. Murota, S. Taguchi, N. Sato, E. Y. Park, Y. Nakamura and K. Sato, Identification of antihyperuricemic peptides in the proteolytic digest of shark cartilage water extract using in vivo activity-guided fractionation, J. Agric. Food Chem., 2014, 62(11), 2392–2397 CrossRef CAS.
- A. B. Nongonierma, C. Mooney, D. C. Shields and R. J. Fitzgerald, Inhibition of dipeptidyl peptidase IV and xanthine oxidase by amino acids and dipeptides, Food Chem., 2013, 141(1), 644–653 CrossRef CAS.
- Q. Li, X. Kang, C. Shi, Y. Li, K. Majumder, Z. Ning and J. Ren, Moderation of hyperuricemia in rats via consuming walnut protein hydrolysate diet and identification of new antihyperuricemic peptides, Food Funct., 2018, 9(1), 107–116 RSC.
- A. Reginato, D. Mount, I. Yang and H. K. Choi, The genetics of hyperuricemia and gout, Nat. Rev. Rheumatol., 2012, 610–621 CrossRef CAS.
- L. Zgaga, E. Theodoratou, J. Kyle, S. M. Farrington, F. Agakov, A. Tenesa, M. Walker, G. McNeill, A. F. Wright, I. Rudan, M. G. Dunlop and H. Campbell, The association of dietary intake of purine-rich vegetables, sugar-sweetened beverages and dairy with plasma urate, in a cross-sectional study, PLoS One, 2012, 7(6), e38123 CrossRef CAS.
- F. Backhed, H. Ding, T. Wang, L. V. Hooper, G. Y. Koh, A. Nagy, C. F. Semenkovich and J. I. Gordon, The gut microbiota as an environmental factor that regulates fat storage, Proc. Natl. Acad. Sci. U. S. A., 2004, 101(44), 15718–15723 CrossRef.
- T. Shao, L. Shao, H. Li, Z. Xie, Z. He and C. Wen, Combined Signature of the Fecal Microbiome and Metabolome in Patients with Gout, Front. Microbiol., 2017, 8, 268 Search PubMed.
- Y. Yu, Q. Liu, H. Li, C. Wen and Z. He, Alterations of the Gut Microbiome Associated With the Treatment of Hyperuricaemia in Male Rats, Front. Microbiol., 2018, 9, 2233 CrossRef.
- T. Cao, X. Li, T. Mao, H. Liu, Q. Zhao, X. Ding, C. Li, L. Zhang and Z. Tian, Probiotic therapy alleviates hyperuricemia in C57BL/6 mouse model, Biomed. Res., 2017, 28, 2244–2249 CAS.
- J. Xu, J. Duan, C. Xue, T. Feng, P. Dong, T. Sugawara and T. Hirata, Analysis and comparison of glucocerebroside species from three edible sea cucumbers using liquid chromatography-ion trap-time-of-flight mass spectrometry, J. Agric. Food Chem., 2011, 59(22), 12246–12253 CrossRef CAS.
- Y. C. Zhao, C. H. Xue, T. T. Zhang and Y. M. Wang, Saponins from Sea Cucumber and Their Biological Activities, J. Agric. Food Chem., 2018, 66(28), 7222–7237 CrossRef CAS.
- C. C. Wang, H. H. Shi, L. Y. Zhang, L. Ding, C.-H. Xue, T. Yanagita, T. T. Zhang and Y.-M. Wang, The rapid effects of eicosapentaenoic acid (EPA) enriched phospholipids on alleviating exercise fatigue in mice, RSC Adv., 2019, 9(58), 33863–33871 RSC.
- Y. Wang, X. Gao, J. Zhou, C. Zhang, Y. Li, Z. Wang, B. Yuan, J. Dai, Q. Qian and X. Su, Studies on the Hypolipidemic Function of Acaudina leucoprocta hydrolyzate hydrolysed by Bacillus licheniformis, J. Chin. Inst. Food Sci. Technol., 2017, 17, 44–50 Search PubMed.
- F. Charte, I. Romero, M. Pérez-Godoy, A. Rivera and E. Castro, Comparative analysis of data mining and response surface methodology predictive models for enzymatic hydrolysis of pretreated olive tree biomass, Comput. Chem. Eng., 2017, 101, 23–30 CrossRef CAS.
- J. Y. Je, K. H. Lee, M. Lee and C.-B. Ahn, Antioxidant and antihypertensive protein hydrolysates produced from tuna liver, Food Res. Int., 2009, 42, 1266–1272 CrossRef CAS.
- T. Dilger, H. Melzl and A. Gessner, Rapid and reliable identification of waterborne Legionella species by MALDI-TOF mass spectrometry, J. Microbiol. Methods, 2016, 127, 154–159 CrossRef CAS.
- L. Xu and L. Shi, Establishment of hyperuricemia rat model with different doses of hypoxanthine and oxonic acid potassium salt, J. Pharmacol. Toxicol. Methods, 2008, 22, 306–310 Search PubMed.
- G. L. Chen, Z. Wei and S. Y. Xu, Effect and Mechanism of Total Saponin of Dioscorea on Animal Experimental Hyperuricemia, Am. J. Chin. Med., 2006, 34, 77–85 CrossRef CAS.
- J. Ye, S. Caihong, H. Yayan, X. Zhang and X. Meitian, Anti-fatigue activity of sea cucumber peptides prepared from Stichopus japonicas in an endurance swimming rat model: Anti-fatigue activity of sea cucumber peptides, J. Sci. Food Agric., 2017, 97, 4548–4556 CrossRef CAS.
- S. Reagan-Shaw, M. Nihal and N. Ahmad, Dose translation from animal to human studies revisited, FASEB J., 2008, 22(3), 659–661 CrossRef CAS.
- S. U. Morton, M. Joshi, T. Savic, A. H. Beggs and P. B. Agrawal, Skeletal muscle microRNA and messenger RNA profiling in cofilin-2 deficient mice reveals cell cycle dysregulation hindering muscle regeneration, PLoS One, 2015, 10(4), e0123829 CrossRef.
- J. G. Caporaso, K. Bittinger, F. D. Bushman, T. Z. DeSantis, G. L. Andersen and R. Knight, PyNAST: a flexible tool for aligning sequences to a template
alignment, Bioinformatics, 2010, 26(2), 266–267 CrossRef CAS.
- S. Abdulkadir and M. Tsuchiya, One-step method for quantitative and qualitative analysis of fatty acids in marine animal samples, J. Exp. Mar. Biol. Ecol., 2008, 354, 1–8 CrossRef CAS.
- G. Dasilva, M. Pazos, E. García-Ejido, J. Pérez-Jiménez, J. Torres, M. Giralt, M. R. Nogués and I. Medina, Lipidomics to analyze the influence of diets with different EPA:DHA ratios in the progression of Metabolic Syndrome using SHROB rats as a model, Food Chem., 2016, 205, 196–203 CrossRef CAS.
- R. Johnson, T. Nakagawa, L. Sánchez-Lozada, M. Shafiu, S. Sundaram, M. Le, T. Ishimoto, Y. Y. Sautin and M. Lanaspa, Sugar, Uric Acid, and the Etiology of Diabetes and Obesity, Diabetes, 2013, 3307–3315 CrossRef CAS.
- W. Pei, L. Xu, G. K. Varshney, B. Carrington, K. Bishop, M. Jones, S. C. Huang, J. Idol, P. R. Pretorius, A. Beirl, L. A. Schimmenti, K. S. Kindt, R. Sood and S. M. Burgess, Additive reductions in zebrafish PRPS1 activity result in a spectrum of deficiencies modeling several human PRPS1-associated diseases, Sci. Rep., 2016, 6, 29946 CrossRef CAS.
- X. Qian, X. Wang, J. Luo, Y. Liu, J. Pang, H. Zhang, Z. Xu, J. Xie, X. Jiang and W. Ling, Hypouricemic and nephroprotective roles of anthocyanins in hyperuricemic mice, Food Funct., 2019, 10(2), 867–878 RSC.
- W. He, G. Su, D. Sun-Waterhouse, G. I. N. Waterhouse, M. Zhao and Y. Liu, In vivo anti-hyperuricemic and xanthine oxidase inhibitory properties of tuna protein hydrolysates and its isolated fractions, Food Chem., 2019, 272, 453–461 CrossRef CAS.
- T. Yong, Y. Xie, S. Chen, D. Chen, J.-Y. Su, C. Jiao, H. Hu and C. Xiao, Hypouricemic effect of Grifola frondosa on hyperuricemic mice and virtual screening of bioactives by 3D QSAR pharmacophore modeling, J. Funct. Foods, 2018, 40, 582–588 CrossRef CAS.
- L. B. Sorensen and D. J. Levinson, Origin and extrarenal elimination of uric acid in man, Nephron, 1975, 14(1), 7–20 CrossRef CAS.
- Y. Ding, H. Yang, W. Xiang, X. He, W. Liao and Z. Yi, CD200R1 agonist attenuates LPS-induced inflammatory response in human renal proximal tubular epithelial cells by regulating TLR4-MyD88-TAK1-mediated NF-kappaB and MAPK pathway, Biochem. Biophys. Res. Commun., 2015, 460(2), 287–294 CrossRef CAS.
- F. Martinon, V. Petrilli, A. Mayor, A. Tardivel and J. Tschopp, Gout-associated uric acid crystals activate the NALP3 inflammasome, Nature, 2006, 440(7081), 237–241 CrossRef CAS.
- M. Dungey, K. L. Hull, A. C. Smith, J. O. Burton and N. C. Bishop, Inflammatory factors and exercise in chronic kidney disease, Int. J. Endocrinol., 2013, 2013, 569831 Search PubMed.
- D. Yagnik, B. Evans, O. Florey, J. Mason, R. Landis and D. Haskard, Macrophage release of transforming growth factor during resolution of monosodium urate monohydrate crystal-induced inflammation, Arthritis Rheum., 2004, 2273–2280 CrossRef CAS.
- Y. Murakami, T. Akahoshi, S. Kawai, M. Inoue and H. Kitasato, Antiinflammatory effect of retrovirally transfected interleukin-10 on monosodium urate monohydrate crystal-induced acute inflammation in murine air pouches, Arthritis Rheum., 2002, 46(9), 2504–2513 CrossRef CAS PubMed.
- M. X. Wang, X. J. Zhao, T. Y. Chen, Y. L. Liu, R. Q. Jiao, J. H. Zhang, C. H. Ma, J. H. Liu, Y. Pan and L. D. Kong, Nuciferine Alleviates Renal Injury by Inhibiting Inflammatory Responses in Fructose-Fed Rats, J. Agric. Food Chem., 2016, 64(42), 7899–7910 CrossRef CAS.
- J. Li, F. Zhao, Y. Wang, J. Chen, J. Tao, G. Tian, S. Wu, W. Liu, Q. Cui, B. Geng, W. Zhang, R. Weldon, K. Auguste, L. Yang, X. Liu, L. Chen, X. Yang, B. Zhu and J. Cai, Gut microbiota dysbiosis contributes to the development of hypertension, Microbiome, 2017, 5(1), 14 CrossRef.
- N. El Husseini, O. Kaskar and L. Goldstein, Chronic Kidney Disease and Stroke, Adv. Chronic Kidney Dis., 2014, 500–508 CrossRef.
- N. Yamada, C. Iwamoto, H. Kano, N. Yamaoka, T. Fukuuchi, K. Kaneko and Y. Asami, Evaluation of purine utilization by Lactobacillus gasseri strains with potential to decrease the absorption of food-derived purines in the human intestine, Nucleosides, Nucleotides Nucleic Acids, 2016, 35(10–12), 670–676 CrossRef CAS.
- L. R. Lopetuso, F. Scaldaferri, V. Petito and A. Gasbarrini, Commensal Clostridia: leading players in the maintenance of gut homeostasis, Gut Pathog., 2013, 5(1), 23 CrossRef.
- M. Nieuwdorp, P. W. Gilijamse, N. Pai and L. M. Kaplan, Role of the microbiome in energy regulation and metabolism, Gastroenterology, 2014, 146(6), 1525–1533 CrossRef CAS.
- I. Martinez, D. J. Perdicaro, A. W. Brown, S. Hammons, T. J. Carden, T. P. Carr, K. M. Eskridge and J. Walter, Diet-induced alterations of host cholesterol metabolism are likely to affect the gut microbiota composition in hamsters, Appl. Environ. Microbiol., 2013, 79(2), 516–524 CrossRef CAS PubMed.
- S. Mondot, P. Lepage, P. Seksik, M. Allez, X. Treton, Y. Bouhnik, J. F. Colombel, M. Leclerc, P. Pochart, J. Dore and P. Marteau, Structural robustness of the gut mucosal microbiota is associated with Crohn's disease remission after surgery, Gut, 2016, 65(6), 954–962 CrossRef CAS PubMed.
Footnote |
† Electronic supplementary information (ESI) available. See DOI: 10.1039/c9fo02425e |
|
This journal is © The Royal Society of Chemistry 2020 |