DOI:
10.1039/C9FO02164G
(Paper)
Food Funct., 2020,
11, 187-199
Chitosan reduces vitamin D bioaccessibility in food emulsions by binding to mixed micelles
Received
17th September 2019
, Accepted 8th December 2019
First published on 9th December 2019
Abstract
Consumption of sufficiently high quantities of dietary fibers has been linked to a range of health benefits. Recent research, however, has shown that some dietary fibers interfere with lipid digestion, which may reduce the bioavailability of oil-soluble vitamins and nutraceuticals. For this reason, we examined the impact of a cationic polysaccharide (chitosan) on the bioaccessibility of vitamin D using the standardized INFOGEST in vitro digestion model. The vitamin D was encapsulated within an emulsion-based delivery system that contained whey protein-coated corn oil droplets. Our results showed that chitosan promoted severe droplet flocculation in the small intestine and reduced the amount of free fatty acids detected using a pH-stat method. However, a back-titration of the digested sample showed that the lipids were fully digested at all chitosan levels used (0.1–0.5%), suggesting that chitosan may have bound some of the free fatty acids released during lipid digestion. The presence of the chitosan decreased the bioaccessibility of vitamin D by about 37%, but this effect did not depend strongly on chitosan concentration (0.1–0.5%). It was hypothesized that chitosan bound to the vitamin-loaded mixed micelles and promoted their precipitation. The knowledge gained in this study might provide useful insights in designing emulsion-based delivery systems with high vitamin bioaccessibility.
1. Introduction
There has been considerable interest in the food industry in the design of functional foods and beverages that not only taste good, but also have specific health benefits.1 A number of approaches are being utilized to create these healthier versions of processed foods. One approach is to decrease the levels, or at least reduce the negative effects, of food ingredients that have been linked to increased health risks, such as fat, sugar, and salt.1 Another approach is to control the structural organization of the constituents within foods so as to alter their behavior inside the gastrointestinal tract (GIT). An example of this approach is to create foods in which the macronutrients are digested more slowly, thereby inhibiting glucose or lipid spikes in the blood that may lead to diabetes or heart disease.2,3 Yet another approach is to fortify foods with bioactive ingredients that have been linked to beneficial health effects, such as vitamins, minerals, and nutraceuticals.4,5 For example, vitamin D is vital for bone health and other critical physiological functions,6 but in many countries, some segments of the population are deficient in this essential micronutrient.7 Nevertheless, the amount of fortification must be carefully controlled because excessive vitamin D intake can have adverse health outcomes, such as gastrointestinal disorders and kidney dysfunction.8,9 For this reason, the design of food matrices that can regulate the release and absorption of micronutrients like vitamin D is of great interest to the modern food industry.10
The purpose of the current study was to examine the impact of a dietary fiber (chitosan) on the bioavailability of vitamin D encapsulated within emulsion-based delivery systems. In general, dietary fibers are claimed to have a number of potentially beneficial health effects.11–16 For instance, they can inhibit glucose absorption, promote satiety, and thereby reduce overall calorie consumption.17 Dietary fibers have also been shown to modulate the gut microflora,18 which is claimed to promote a healthy immune system and reduce the susceptibility to certain chronic diseases.19 Dietary fibers have also been shown to modulate the rate and extent of lipid digestion in emulsion-based delivery systems,20,21 which may impact the bioavailability of encapsulated oil-soluble vitamins. Several possible mechanisms have been proposed to account for the ability of dietary fibers to interfere with lipid digestion: (1) formation of coatings around oil droplets;22,23 (2) promotion of droplet flocculation;24 (3) thickening or gelling of gastrointestinal fluids;25–27 (4) binding to critical components in the lipid digestion process, such as bile salts, free fatty acids,24,28 digestive enzymes,29 and, calcium ions;30 (5) binding to mixed micelles.31
The tendency for specific dietary fibers to be involved in these different mechanisms depends on their molecular and physicochemical characteristics (such as molecular weight, charge, conformation, and solubility), as well as food matrix properties (such as composition and structure). We hypothesized that chitosan could impact the bioaccessibility of vitamin D by interfering with the lipid digestion process by one or more of the above mechanisms.
Food-grade chitosan is commonly produced by deacetylation of the chitin found in the shells of crustaceans, such as crabs and shrimps.32,33 It is one of the few positively charged polysaccharides available and so has been widely used as a functional ingredient in foods and other commercial products for its binding, structure building, film forming, and other functional attributes.34 Chitosan is widely used in research studies but does not currently have GRAS approval for use as a food additive in the US,35 although it is used in some other countries.36 The positive charge on chitosan molecules is due to aliphatic amino groups that are protonated (–NH3+) under acidic conditions: pKa ∼ 6–7.37 The cationic nature of chitosan is believed to be responsible for many of its physiological effects in the human gut after digestion. Several studies have shown that chitosan can inhibit lipid digestion and reduce the bioaccessibility of hydrophobic bioactives (such as curcumin).38,39 These effects have been attributed to some of the physicochemical mechanisms listed earlier. For instance, cationic chitosan can form a protective coating around anionic oil droplets, or promote their flocculation, which inhibits lipid digestion by reducing the ability of lipase to reach the lipid phase.40 Besides, chitosan can bind to anionic free fatty acids and bile salts,31 which might alter the number of nutraceutical-loaded mixed micelles available for adsorption in the upper gastrointestinal tract (GIT).
The main objective of the current study was therefore to examine the impact of chitosan on the gastrointestinal fate of vitamin D-loaded oil droplets in emulsion-based delivery systems. In particular, we used the standardized INFOGEST simulated GIT model to examine the impact of chitosan on lipid digestion and vitamin bioaccessibility.41 The results obtained from this study may facilitate the design of more effective emulsion-based delivery systems for oil-soluble vitamins and other non-polar bioactive agents.
2. Materials and methods
2.1. Materials
Corn oil (Mazola, ACH Food Companies, Memphis, TN, USA) was obtained from a supermarket. Whey protein isolate (WPI) was provided by Agropur Inc. (Eden Prairie, MN). Chitosan (Chitoclear cg 800, degree of deacetylation >75%, viscosity 600–1200 mPa s) was provided by Primex ehf. (Siglufjordur, Iceland). Vitamin D3 (1.0 Mill. I.U. g−1) was supplied by BASF (Ludwigshafen, Germany). Porcine gastric mucin, pepsin from porcine gastric mucosa (250 units per mg), pancreatin from porcine pancreas, porcine lipase (100–400 units per mg), porcine bile extract, and bile acid assay kit were purchased from the Sigma-Aldrich Company (St Louis, MO, USA). Ethyl alcohol (ACS/USP grade) was purchased from Pharmco Products, Inc. (Shelbyville, KY, USA). All other chemicals and reagents (analytical grade or higher) were purchased from either Sigma-Aldrich or Fisher Scientific (Pittsburgh, PA, USA). Double distilled water was produced by a laboratory water-purification system (Nanopure Infinity, Barnstaeas International, Dubuque, IA, USA) and was used to prepare all solutions in this study.
2.2. Emulsion preparation
Oil-in-water emulsions were prepared according to a method reported previously.42 An aqueous phase was prepared by dispersing powdered WPI into buffer solution (5 mM phosphate, pH 7.0) and then stirring for at least 3 hours at ambient temperature. The resulting solution was then stored at 4 °C overnight to completely hydrate the protein. The aqueous phase was filtered through Whatman qualitative filter paper (Fisher Scientific) before being used to remove any insoluble matter. The mixture of oil and aqueous phases was first subject to 2 min blending by a high-speed mixer (M133/1281-0, Biospec Products, Inc., ESGC, Switzerland) at 10
000 rpm, and then homogenized by passing through a microfluidizer (M110Y, Microfluidics, Newton, MA) at an operation pressure of 12
000 psi for five times. The final composition of the emulsion was 10 wt% oil phase (2 wt% vitamin D in corn oil) and 90 wt% aqueous phase (1.11 wt% WPI in buffer solution). This led to a WPI concentration of 1 wt% in the final emulsion.
2.3. Chitosan solution preparation
A chitosan stock solution was prepared by dissolving 1 wt% chitosan in 0.5% (v/v) acetic acid solution and then storing at 4 °C overnight to ensure complete hydration. Any insoluble matter was then removed from this solution by centrifugation (Sorvall Lynx 4000 centrifuge, Thermo Scientific, Waltham, MA, USA) at 15
000 rpm for 30 min at room temperature.
2.4 Emulsion and chitosan mixing method
The chitosan stock solution was diluted with different amounts of 0.5% (v/v) acetic acid solution to obtain a series of solutions containing different chitosan levels (0%, 0.2%, 0.4%, 0.6%, 0.8% and 1%). These solutions were then mixed with the emulsions at a 1
:
1 (v/v) ratio by adding the emulsions dropwise into beakers containing the chitosan solutions. The resulting mixtures were then stirred for 5 min to ensure they were homogenous and then placed at room temperature overnight before in vitro digestion.
2.5. Particle size measurements
The particle size distribution of the emulsions was determined using laser diffraction (Mastersizer 2000, Malvern Instruments Ltd, Malvern, Worcestershire, UK). Samples were diluted in aqueous solutions and stirred in the dispersion unit at a speed of 1200 rpm to ensure homogeneity. A 1
:
1 (v/v) mixture of 0.5% (v/v) acetic acid and phosphate buffer (5 mM, pH 7.0) was used to dilute the initial samples. Double distilled water with pH adjustment was used for the mouth (pH 4.8) and stomach (pH 3) samples. Phosphate buffer (5 mM, pH 7.0) was used to dilute the small intestine samples. The refractive index of the corn oil used in the calculations was 1.472. Average particle sizes are reported as the surface-weighted mean diameter (D3,2). The particle size of the mixed micelle samples was measured by dynamic light scattering (Zetasizer, Nano ZS series, Malvern Instruments Ltd). Phosphate buffer (5 mM, pH 7.0) was used to dilute the samples prior to analysis to avoid multiple scattering effects.
2.6. Particle surface potential measurements
The surface potential (ζ-potential) of the particles in the emulsions was measured using electrophoresis (Zetasizer Nano ZS series, Malvern Instruments Ltd). Prior to analysis, the emulsions were diluted with the same solutions used for the laser diffraction measurements.
2.7. Confocal microscopy
Confocal microscopy images were acquired according to a method described previously.43 Prior to measurement, the oil phase was dyed with Nile red solution (1 mg Nile red in 1 ml ethanol) at a ratio of 1
:
20 (v/v) of dye to oil phase. An aliquot (5 μL) of sample was put onto a microscope slide and then a coverslip was placed on top. The excitation and emission wavelengths used for Nile red fluorescence analysis were 543 and 605 nm, respectively. The microscopy images were acquired using a confocal scanning laser microscope (Nikon D-Eclipse C1 80i, Nikon, Melville, NY, USA) with a 60× oil immersion objective lens. The images obtained were stored and analyzed using the instrument software (NIS-Elements, Nikon, Melville, NY).
2.8.
In vitro digestion
The chitosan-emulsion mixtures were passed through a simulated GIT model based on the standardized INFOGEST method.41 All solutions were preheated to 37 °C prior to use and the whole GIT procedure was performed at this temperature.
Mouth phase.
Preheated simulated saliva fluid containing 0.003 g ml−1 mucin was mixed 1
:
1 (v/v) with the test samples. The sample was then agitated using a thermally-incubated shaker (Model 4080, New Brunswick Scientific, New Brunswick, NJ, USA) for 2 min at a shaking speed of 100 rpm.
Stomach phase.
The sample from the mouth phase was mixed 1
:
1 (v/v) with simulated gastric fluids containing pepsin (2000 U ml−1 in the final digestion mixture). After adjusting to pH 3.0, the sample was then agitated using the same incubated shaker for 2 h at 100 rpm.
Small intestine phase.
The sample from the stomach phase was mixed 1
:
1 (v/v) with simulated intestinal fluids containing bile salts (10 mM in the final mixture) and pancreatic enzymes (trypsin activity of 100 U ml−1 and lipase activity of 2000 U ml−1 in the final mixture). After adjusting to pH 7.0, the sample was incubated in a water bath for 2 h with continuous stirring. During this time, an automatic titration unit (Metrohm, USA, Inc.) was used to maintain the sample at pH 7.0 by adding alkaline solution (0.25 M NaOH) to titrate the free fatty acids (FFAs) released. The volume of alkaline solution required to achieve neutralization was recorded and used to calculate the % of FFAs generated. A back titration to pH 9.0 was also carried out to determine the total level of free fatty acids released (since some of them may be protonated at neutral pH). A blank test was carried out using the same composition as the sample being tested but without the oil to determine the contribution of any non-lipid components to the titration curves. After 2 h of digestion under small intestine conditions, an aliquot of intestine sample was centrifuged (Sorvall Lynx 4000 centrifuge, Thermo Scientific, Waltham, MA, USA) at 30
970g (18
000 rpm) for 50 min at 4 °C to separate the micelle phase.
2.9. Direct mixing of micelle and chitosan
In one series of experiments, we aimed to determine the impact of adding chitosan to the mixed micelles formed during digestion. To achieve this, a corn oil-in-water emulsion (5% oil) was subjected to the above in vitro digestion procedure, and then the resulting mixed micelle phase was collected after centrifugation. Different amounts of chitosan stock solution (1 wt%) was then mixed with the mixed micelle samples to reach 0%, 0.0125%, 0.025%, 0.03%, 0.0375%, 0.05% and 0.0625% of chitosan, which is similar to the chitosan levels present in the chitosan–emulsion mixtures after in vitro digestion. The mixed micelle–chitosan mixtures were then placed in the incubated shaker at 37 °C at 100 rpm for 2 h. Afterwards, the micelle–chitosan mixtures were centrifuged (Sorvall Lynx 4000 centrifuge) at 30
970g (18
000 rpm) for 30 min at 4 °C to precipitate the sediment. The vitamin D concentration in the micelle–chitosan mixtures and the supernatants formed after centrifugation were measured using the method described in the following section. The mass of the sediment was weighed after oven-drying at 37 °C overnight. The percentage of sediment formed was then calculated using the following expression:
Here, mmixture and msediment are the mass of the micelle–chitosan mixture and the sediment, respectively.
2.10 Vitamin D measurement
The extraction and analysis of the vitamin D concentration was carried out using a protocol based on a previous study.44 The vitamin D was extracted using an organic solvent consisting of a 1
:
1 (v/v) mixture of hexane and ethanol for three times. After being treated using a saturated sodium chloride solution, the combined extracts were dried under a nitrogen atmosphere and then re-dissolved in HPLC-grade methanol. After being passed through a 0.45 μm filter to remove any particles (VWR International, Philadelphia, PA, USA), the samples were analyzed by HPLC.
A reverse phase-HPLC system (Agilent 1100 series, Agilent Technologies, Santa Clara, CA, USA) with a Zorbax SB-C18 column (4.6 × 250 mm, 5 μm, Agilent Technologies, Santa Clara, CA, USA) was used to carry out the separation and analysis. The mobile phase consisted of a methanol and water mixture (95
:
5, v/v), and a flow rate of 1 ml min−1 was used. After injection of 20 μL of sample, the absorbance of the eluent was monitored at 265 nm.
The vitamin bioaccessibility (%) was calculated from measurements of the vitamin D concentrations in the total digest and in the mixed micelles:
Here, Cmicelle and Cdigest are the concentrations of vitamin D in the mixed micelle phase and in the total digest collected after the simulated small intestine phase, respectively.
2.11. Statistical analysis
The digestion process and all measurements were carried out in triplicate, while the emulsion preparation was carried out in duplicate. The means and standard deviations were calculated by combining the data from these different measurements. Depending on the homogeneity of the variances, either a Duncan test (homogenous) or Dunnett's T3 test (inhomogeneous) was used in the analysis of variance (ANOVA) at a confidence level of 95% to determine the statistical differences among treatments. Statistical calculations were carried out using commercial software (SPSS, IBM Corp., Armonk, NY, USA).
3. Results and discussion
3.1. Physical and structural properties during in vitro gastrointestinal digestion
Initially, corn oil-in-water emulsions containing different chitosan concentrations were prepared. These mixtures were then passed through the in vitro digestion model to obtain a better understanding of their potential gastrointestinal fate, especially the impact of the chitosan on lipid digestion and vitamin bioaccessibility. The particle size, surface charge, and microstructure of the emulsion–chitosan mixtures were measured in each stage of the GIT model.
Initial.
The emulsion–chitosan mixtures were prepared by adding the corn oil emulsion (in phosphate buffer, pH 7) drop-by-drop into the chitosan solution (in acetic acid solution, pH 3.7). The resulting solutions had pH values ranging from 3.9 to 4.6, increasing with decreasing chitosan concentration. The surface charge of the particles in all of the emulsions was highly positive. The pure emulsion (no chitosan) had a surface charge of +48.8 mV (Fig. 3), because the final pH was below the isoelectric point of the adsorbed proteins (pI ≈ 5). The pure emulsion had a monomodal distribution and a mean particle diameter of 148 nm, which is fairly similar to that of the original emulsion i.e., 139 nm (Fig. 1 and 2a). The confocal microscopy images showed that these emulsions contained fine oil droplets that were evenly distributed throughout the samples (Fig. 4). These results indicate that the pure emulsion was stable under these pH conditions, which is probably due to the strong electrostatic repulsion between the highly cationic droplets.
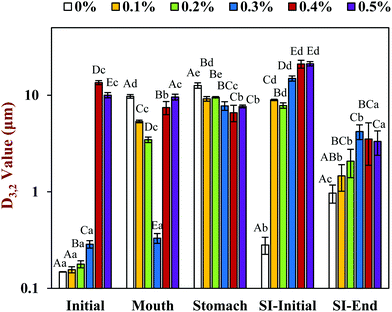 |
| Fig. 1 The effect of different chitosan concentrations on the surface-weighted mean particle diameter (D3,2) of the corn oil in water emulsion during stimulated gastrointestinal tract. Significant difference was labeled by different capital letters (A, B, C) for different chitosan concentrations (same phase), whereas lower-case letters (a, b, c) for different phases (same chitosan concentration). Abbreviation: small intestine (SI). | |
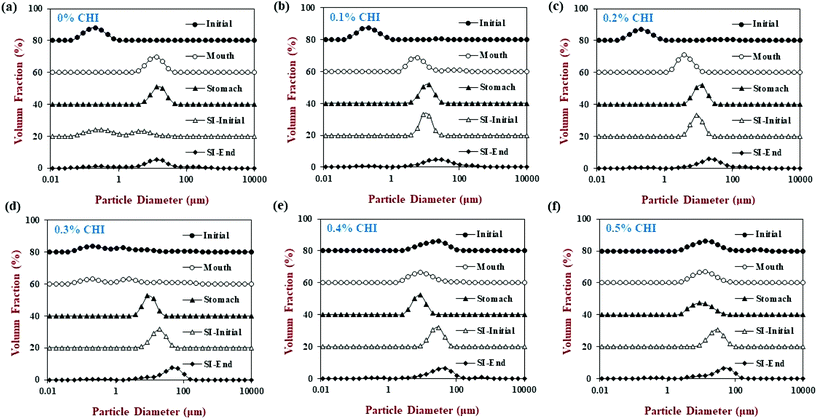 |
| Fig. 2 The effect of different chitosan concentrations on the particle size distribution of the corn oil in water emulsion during stimulated gastrointestinal tract. Abbreviation: small intestine (SI). Note: the volume fraction was stacked up the y-axis for comparison. | |
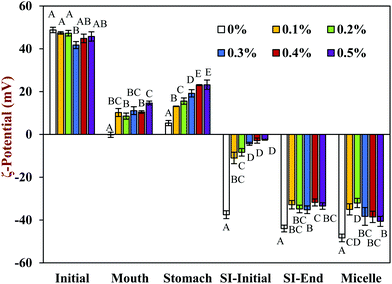 |
| Fig. 3 The effect of different chitosan concentrations on the ζ-potential of the corn oil in water emulsion during stimulated gastrointestinal tract. Capital letters (A, B, C) were used for distinguish significant difference of samples with different chitosan concentration (same phase). Abbreviation: small intestine (SI). | |
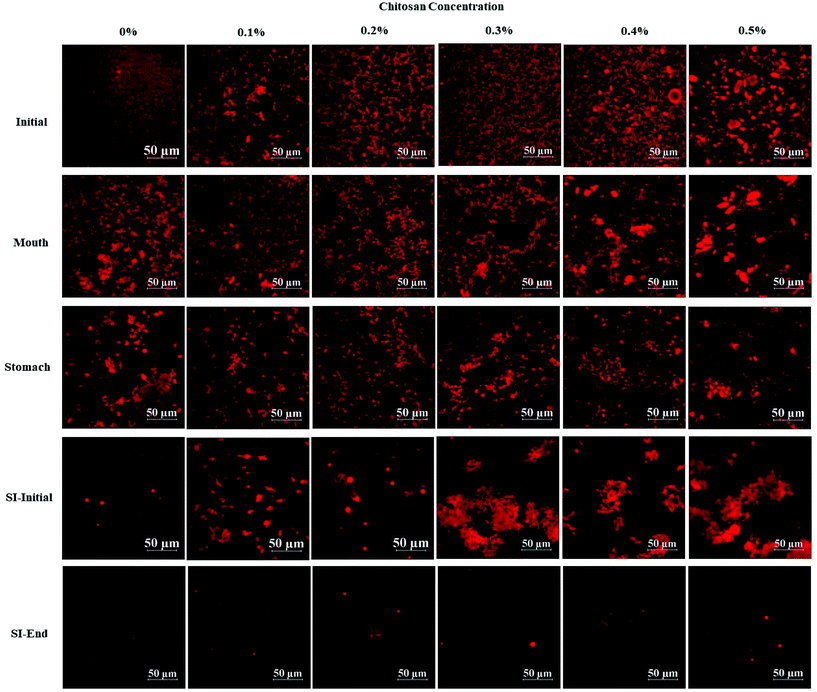 |
| Fig. 4 The effect of different chitosan concentrations on the confocal microscopy of the corn oil in water emulsion during stimulated gastrointestinal tract. Abbreviation: small intestine (SI). | |
In the presence of chitosan, the positive surface potential of the emulsions remained relatively high and positive (+41.7 to +47.3 mV) with increasing chitosan concentration (0.1–0.5%) (Fig. 3). This effect may have been because the chitosan did not bind strongly to the droplet surfaces under these acidic conditions because both the chitosan and lipid droplets had strong positive charges, leading to an electrostatic repulsion. In addition, as the pH increased from 4.1 to 4.6, and therefore moved closer to the isoelectric point of the adsorbed whey proteins, some of the cationic chitosan molecules could have bound to anionic patches formed on the protein-coated droplet surfaces.45 As a result, the net positive charge remained relatively high. The confocal microscopy images suggest that appreciable droplet flocculation occurred in the emulsions at all chitosan levels used, which is most likely a result of bridging or depletion flocculation.45,46 The extent and nature of flocculation depended on the chitosan concentration. At low chitosan levels (0.1%), severe droplet flocculation occurred (Fig. 4) and the emulsions visually separated into a cream layer on top and a serum layer at the bottom (Fig. 5). Surprisingly, the measured mean particle diameter only increased slightly to 156 nm in the presence of 0.1% chitosan (Fig. 1), which suggests that either the flocculated droplets were only held together by relatively weak reversible attractive interactions, or that only a small fraction of the droplets were flocculated.
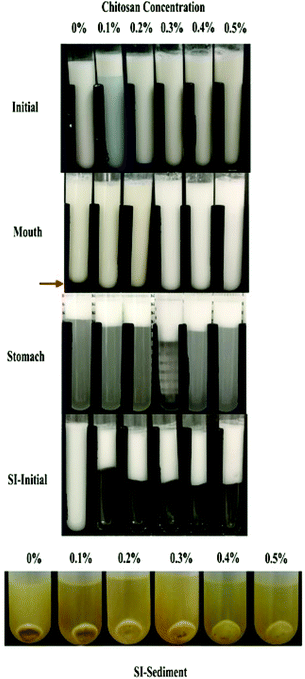 |
| Fig. 5 Appearance of emulsions containing different chitosan concentrations at different digestive stages. Abbreviation: SI-initial = the initial stages of the small intestine phase. | |
When the chitosan concentration was increased to 0.3%, the flocculated oil droplets appeared to be more evenly spread (less clumping) and the emulsions showed less phase separation (Fig. 4 and 5). In this case, the mean particle diameter increased significantly (p < 0.05) to 286 nm (Fig. 1) and the particle size distribution became bimodal. These results suggest that the oil droplets were more strongly aggregated to each other and formed a 3D-network that was more resistant to gravitational separation. At 0.4 and 0.5% chitosan, there was some evidence of clumping of the flocculated droplets, a steep increase in mean particle diameter, and a shift in the particle size distribution to higher values (Fig. 1, 2 and 4). These effects may have been due to increasing bridging and/or depletion flocculation as the chitosan level was increased.46,47
Mouth.
The physical and structural properties of the samples changed appreciably after exposure to the oral phase. After mixing with the simulated salivary fluids, the solutions had pH values from 4.5 to 5.1. The pure emulsion was unstable under mouth conditions with visible phase separation, an increase in mean particle diameter (D3,2 = 9.7 μm), and evidence of floc formation in the microscopy images (Fig. 1, 4 and 5). This effect may have been because of the relatively low surface potential (ζ = −0.17 mV) of the protein-coated oil droplets near the isoelectric point of the proteins, which led to a relatively weak electrostatic repulsion between them.
The addition of chitosan improved the stability of the emulsions by an amount that depended on the concentration used (Fig. 5). As the chitosan level was increased, the mean particle diameter decreased reaching a value of 331 nm at 0.3% chitosan level (Fig. 1), but a broad particle size distribution was observed (Fig. 2d). This effect may have been due to the ability of the chitosan molecules to adsorb to the surfaces of the protein-coated oil droplets, increase their positive charge, and strengthen the electrostatic repulsion between them. This hypothesis is supported by the observation that the ζ-potential of the droplets became increasingly positive as the chitosan level increased (Fig. 3). Nevertheless, the confocal microscopy images showed that the protein-coated oil droplets were still highly flocculated in the presence of chitosan (Fig. 4). These results suggest that there were still some bridging and/or depletion flocculation at high polysaccharide levels, but the flocs formed were weak enough to dissociate when the samples were diluted for the particle size measurements.
Stomach.
In the stomach phase, the emulsion samples were exposed to highly acidic conditions (pH 3), as well as digestive enzymes (pepsin). All the samples were highly unstable to droplet aggregation (Fig. 1, 2, and 4) and phase separation (Fig. 5). The mean particle diameter was relatively high (>7 μm) at all chitosan levels (0 to 0.5%) (Fig. 1). Droplet aggregation was probably a result of changes in electrostatic interactions, bridging flocculation, and protein digestion. After exposure to the stomach phase, the ζ-potential of the particles was positive in all the samples, increasing from +5.3 mV in the absence of chitosan to +23.2 mV in the presence of 0.5%, chitosan. One might have expected that this large increase in particle charge would have led to stronger electrostatic repulsion between the oil droplets, but there was not a major change in particle aggregation with increasing chitosan levels. This phenomenon might be explained by a number of factors: (1) the change in pH caused partial or full displacement of the chitosan from the oil droplet surfaces; (2) the dilution of the samples reduced the amount of chitosan coating the droplet surfaces, thereby promoting bridging; (3) the digestion of the adsorbed proteins by pepsin reduced the stabilizing effects of the emulsifier; and (4) the higher ionic strength of the gastric fluids screened the electrostatic repulsion.48
SI-initial.
Since pH is known to play a critical role in determining the physical and structural properties of protein-stabilized emulsions,49 we measured the properties of the samples at the beginning of the small intestine phase before adding the digestive enzymes and bile salts. This was accomplished by adjusting the sample collected after the stomach phase to pH 7. The properties of all the samples changed appreciably when the pH was increased. The average mean particle diameter of the pure emulsion decreased steeply to 281 nm (Fig. 1), and populations of both small and large particles were seen in the particle size distribution (Fig. 2a). This result matched with the confocal microscopy images, which showed most of the flocs were dispersed into small oil droplets with only a few large droplets (Fig. 4). The breakdown of the flocs can mainly be attributed to an increase in the electrostatic repulsion between the droplets due to an increase in their surface potential to a high negative value (−37.5 mV) (Fig. 3). The fact that there were large individual oil droplets present suggests that some coalescence occurred within the mouth and/or stomach phases. Visual observation of the pure emulsions indicated that they were stable at the beginning of the small intestine phase (Fig. 5). Overall, these results suggest that most of the oil droplets stayed intact and were evenly dispersed throughout the samples.
In contrast, the emulsions containing chitosan became more unstable when they were moved from the stomach phase to the initial part of the small intestine phase. Visual observations showed that all these emulsions underwent phase separation, with a white cream layer on top and a clear serum layer at the bottom (Fig. 5). The addition of chitosan significantly (p < 0.05) increased the mean particle diameter of these samples, with the effect becoming more pronounced with increasing chitosan concentration (Fig. 1). Extensive droplet flocculation was also observed in the confocal microscopy images (Fig. 4). This effect can be attributed to at least two phenomena. First, chitosan is slightly positively charged at pH 7 whereas the protein-coated droplets are strongly negatively charged, thereby promoting bridging flocculation. Second, chitosan loses most of its positive charge under neutral conditions (pKa around 6.5), which causes it to precipitate. These two mechanisms are supported by the electrophoresis measurements, which show that the negative charge on the particles decreased with increasing chitosan concentration: from −37.5 mV in the absence of chitosan to −2.5 mV in the presence of 0.5% chitosan (Fig. 3). In summary, the presence of the chitosan appears to promote the aggregation of the oil droplets.
SI-end.
The properties of the samples were measured again after bile salts and digestive enzymes were added and the samples were then incubated for 2 hours. At the end of the small intestinal phase, the mean particle diameter of the pure emulsions increased significantly (p < 0.05) to around 970 nm (Fig. 1). This change might be brought about by the digestion of the lipid droplets and the formation of large colloidal structures like vesicles and insoluble calcium soaps.50 The magnitude of the surface potential in the pure emulsion sample increased slightly to −44.0 mV (Fig. 3), which has been attributed to the presence of colloidal particles comprised of anionic constituents, such as free fatty acids, bile salts, and peptides.50 The confocal microscopy images indicated that most of the oil droplets had been digested by the end of the small intestine phase (Fig. 4).
Compared to the beginning of the small intestinal phase, the mean particle diameters of the samples containing chitosan decreased greatly after the small intestine phase. Even so, the D3,2 value still increased with increasing chitosan concentration: from 0.97 μm in the absence of chitosan to 3.33 μm in the presence of 0.5% chitosan (Fig. 1). The confocal microscopy images also indicated that most of the oil droplets were digested at all chitosan concentrations, but that there were slightly more lipid-rich particles remaining at higher chitosan levels (Fig. 4). Interestingly, these results show that even though the emulsions containing chitosan were highly flocculated at the beginning of the small intestine phase, most of the oil droplets were still digested by the end. Unlike at the beginning of the small intestine phase, the surface potential of the samples containing chitosan was highly negative and did not change much at different chitosan levels ranging from −32.0 to −40.6 mV. This effect can be attributed to the relatively high level of anionic components in the small intestine phase, such as free fatty acids and bile salts. Presumably, these components bound to the positively charged chitosan molecules and neutralized their charge.
The surface potential of the mixed micelles collected after centrifugation of the digest were also measured (Fig. 3). The ζ-potential of the mixed micelle samples were fairly similar to those of the equivalent whole intestinal samples, suggesting that the mixed micelles dominated the overall charge characteristics.
3.2. Release of free fatty acids during intestinal digestion
The release of free fatty acids (FFA) from the emulsions within the small intestine phase was recorded using a pH stat method by adding increasing volumes of alkaline solution to maintain a neutral pH. The FFA-time release profiles are shown in Fig. 6a and the final FFA values are shown in Fig. 6b. The measured FFA values increased rapidly during the first few minutes of digestion but then increased more steadily at longer times. In general, the level of FFAs detected in the pure emulsions was higher than that detected in the emulsions containing chitosan. For instance, the final FFA value was around 65% for the pure emulsion, whereas it was around 47% to 53% for the emulsions containing chitosan. These results appear to be inconsistent with the confocal microscopy images, which showed that most of the oil droplets had been fully digested by the end of the small intestine phase (Fig. 4). This apparent inconsistency might be due to the fact that a fraction of the FFAs were not ionized at pH 7 and so could not be titrated. To test this hypothesis, a back titration to pH 9 was carried out, since this protocol has previously been shown to be capable of measuring all of the FFAs present.51 After back titration, the final FFA values of all the samples (102% to 115%) suggested that full lipid digestion had occurred. This result suggests that chitosan did not inhibit lipid digestion, but instead altered the amount of FFAs that could be detected by the pH stat method at pH 7. Presumably, the cationic chitosan molecules bind to any anionic free fatty acids at neutral pH thereby reducing the number of titratable protons (H+) released.
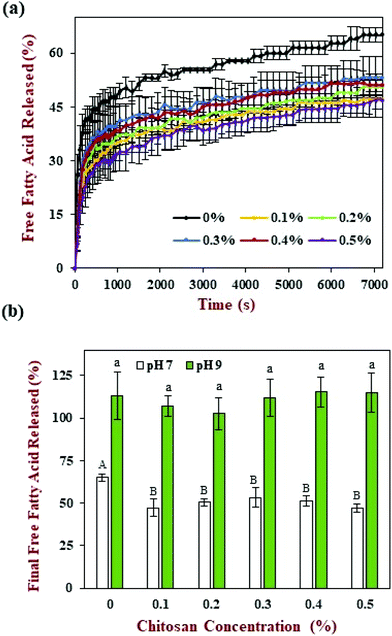 |
| Fig. 6 Impact of chitosan concentration on the release of free fatty acids (FFA) from corn oil-in-water emulsions during simulated digestion. (a) FFA profile at pH 7, (b) final FFA value at pH 7 and pH 9. Capital (A, B, C) and lower-case (a, b, c) letters were used to designate significant difference among different chitosan concentrations for final FFA value at pH 7 and pH 9 respectively. | |
In previous studies, it has been suggested that chitosan can inhibit lipid digestion through a number of mechanisms, including inducing oil droplet flocculation, forming coatings around oil droplets, or binding to digestive components (like enzymes or bile salts).52 Our current results suggest that some of these earlier observations might have been because a back-titration step was not carried out. As a result, not all of the FFAs released during lipid digestion were measured. In addition, most of the earlier studies were carried out using an in vitro digestion method that contained different levels of digestive enzymes, calcium, and other components to the standardized INFOGEST digestion method used in the current study.41 In particular, the INFOGEST method uses higher levels of pancreatic enzymes and lower levels of calcium, which may alter the rate and extent of lipid digestion. Having said this, our results still show that chitosan does interfere with the lipid digestion process under neutral conditions.
3.4. Vitamin bioaccessibility
The vitamin D bioaccessibility in the emulsion–chitosan mixtures was measured after they had been passed through the entire in vitro simulated digestion model (Fig. 7). The pure emulsion had the highest bioaccessibility (68.5%), which is quite similar to the value published previously (75.2%) for a fairly similar system.44 The addition of chitosan significantly (p < 0.05) reduced the bioaccessibility of the vitamin in the emulsion (37.5% to 47.0%), but the decrease did not depend strongly on chitosan concentration. There have been few previous studies on the impact of chitosan on the bioaccessibility of lipophilic bioactives. One study showed that chitosan enhanced the bioaccessibility of curcumin,24 while others showed that it reduced the bioaccessibility of EGCG and carotenoids.52,53 These differences might be due to differences in the bioactive agents, food matrices, and in vitro digestion models used by different researchers.
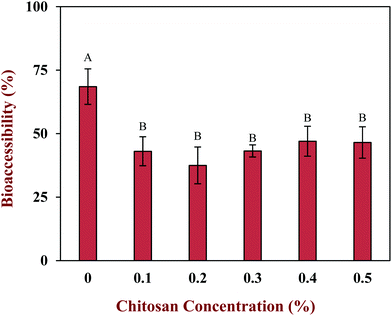 |
| Fig. 7 The effect of different chitosan concentrations on the vitamin D bioaccessibility of the corn oil in water emulsion during stimulated gastrointestinal tract. Samples with significant difference were labeled with different capital letters (A, B, C). | |
In general, the bioaccessibility of oil-soluble vitamins in emulsion-based delivery systems is governed by two processes: (1) their release from the oil droplets due to digestion of the triglycerides; (2) their solubilization within the mixed micelles. Several previous studies have suggested that there is a relationship between the extent of lipid digestion and the bioaccessibility of hydrophobic bioactives.24,38,52 As shown by Fig. 4 and 6, however, the majority of the oil phase was fully digested by the enzymes in this study, so all of the vitamin D should have been released from the oil droplets. Thus, the decrease in vitamin bioaccessibility observed in the presence of chitosan was probably not due to its impact on lipid digestion. Instead, we hypothesized that it was related to the micellization process. This process may be altered through a number of physicochemical mechanisms: (1) modification of the properties of the mixed micelles formed, such as the size of their hydrophobic domains;42 (2) reduction of the total amount of mixed micelles formed during digestion;54 and, (3) precipitation of some of the mixed micelles.55 In this study, we believe that the cationic chitosan molecules bound to the anionic vitamin-loaded mixed micelles, which led to the formation of large insoluble aggregates. These aggregates would be removed from the digest during the centrifugation process and so would not be detected as part of the mixed micelle phase. This hypothesis is supported by visual observations of the samples, which showed that an increasing amount of sediment was formed at the bottom of the tubes as the chitosan concentration was increased (Fig. 5). It should be noted, however, that these precipitated mixed micelles might be liberated when the chitosan is digested by the gut microbiota in the colon, and so the vitamin may be released and become bioaccessible again. This might be a good strategy for controlling the release of vitamin D in the human body, but further in vivo studies are needed to test this.
3.5. Interaction between micelle and chitosan
Finally, we carried out an additional experiment to examine the potential interactions between chitosan and mixed micelles. In this case, chitosan was directly mixed with the mixed micelle phase formed by digestion of a pure emulsion sample. In the absence of chitosan, the mean particle diameter of the mixed micelle solution was 218 nm (Fig. 8a), which is higher than that reported for simple micelles (<10 nm). This was probably because a variety of other colloidal structures were formed during digestion under fed state conditions, including vesicles, calcium soaps, and protein aggregates.56,57 The addition of 0% chitosan solution (just acetic acid solution), significantly (p < 0.05) increased the mean particle size of the mixed micelles to 380 nm. It is possible that the acetic acid changed the ionic equilibration of the free fatty acids thereby altering the mixed micelle structure. The addition of 0.1 to 0.5% chitosan solutions reduced the mean particle size, which was similar to that of the pure micelle samples. Interestingly, the chitosan level did not appear to alter the particle size.
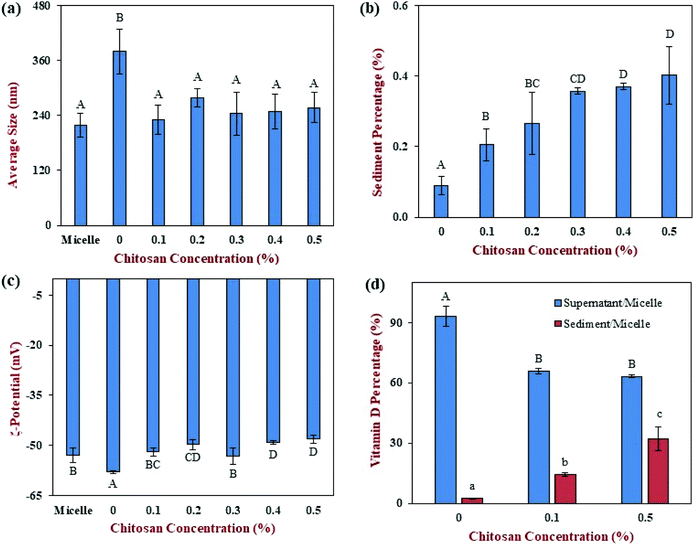 |
| Fig. 8 The effect of different chitosan concentrations on the physical properties and vitamin D distribution of mixed micelles prepared from corn oil-in-water emulsions after exposure to the simulated gastrointestinal tract: (a) mean particle diameter; (b) percentage of sediment formed; (c) ζ-potential; (d) vitamin D percentage in supernatant or sediment. Samples with significant differences were labeled with different capital letters (A, B, C), whereas samples with different lower-case letters (a, b, c) were used for vitamin D percentage of sediment. | |
We hypothesize that the cationic chitosan bound to some of the anionic mixed micelles and caused them to precipitate. As a result, only the properties of the mixed micelles remaining in the aqueous phase would be measured by the dynamic light scattering instrument. This hypothesis was supported by measurements of the percentage of sediment formed, with the amount increasing from 0.09% at 0% chitosan to 0.4% at 0.5% chitosan (Fig. 8b). This hypothesis is also supported by the surface potential measurements, which showed that the colloidal particles in the mixed micelle phase were strongly negatively charged at all chitosan levels (Fig. 8c). Any mixed micelles that bind to the chitosan are neutralized and precipitate, and are therefore not measured by the ζ-potential instrument.
The sedimentation of the mixed micelles in the presence of chitosan also influenced the location of the vitamin D in the system (Fig. 8d). The addition of chitosan significantly (p < 0.05) reduced the amount of vitamin D in the supernatant phase from 93.3% at 0% chitosan to 65.9% at 0.1% chitosan (Fig. 8d). Further addition of chitosan led to a further slight decrease in the percentage of vitamin D in the supernatant phase, reaching 63.3%. These results therefore support the bioaccessibility data discussed above. They suggest that chitosan binds to the vitamin-D loaded mixed micelles causing them to sediment, thereby reducing their bioaccessibility.
4. Conclusions
In this study, the impact of chitosan addition (0–0.5%) on the gastrointestinal fate of vitamin-loaded oil-in-water emulsions was examined. In particular, the effects of chitosan on the extent of lipid digestion and the bioaccessibility of vitamin D3 were investigated. The recently updated standardized INFOGEST protocol was used to simulate the conditions in the upper regions of the human gastrointestinal tract. Chitosan induced severe droplet flocculation in the small intestine phase but this did not reduce the total amount of free fatty acids released by the end of digestion. Indeed, the oil droplets in all the emulsions were fully digested, regardless of the amount of chitosan present. It is important to note that a fraction of the free fatty acids released were not completely ionized under neutral pH conditions, and so a back titration was required to measure the total amount of free fatty acids released. Comparison of the results with and without the back-titration suggested that chitosan altered the ionization state of the free fatty acids. The presence of chitosan (0.1 to 0.5%) decreased the bioaccessibility of vitamin D3 by about 40% when compared to the samples containing no chitosan, with the chitosan level used not having a major effect. As the triglycerides in all the samples were fully digested, we attribute the reduction in vitamin bioaccessibility to the ability of chitosan to modulate the micellization process. Cationic chitosan molecules bind to anionic vitamin-loaded mixed micelles, leading to the formation of insoluble precipitates that are removed from the mixed micelle phase. Our results therefore suggest that incorporating chitosan into foods as a functional ingredient could have a negative impact on vitamin bioaccessibility. However, in vivo experiments are required to determine whether the same effect occurs under more realistic gastrointestinal conditions.
Conflicts of interest
There are no conflicts to declare.
Acknowledgements
This material was partly based upon work supported by the National Institute of Food and Agriculture, USDA, Massachusetts Agricultural Experiment Station (MAS00491). We also thank the Chinese Scholarship Council (2017-06150098) for support.
References
-
D. J. McClements, Future Foods: How Modern Science is Transforming the Way We Eat, Springer Scientic, Njew York, NY, 2019 Search PubMed.
- M. N. Corstens, C. C. Berton-Carabin, R. de Vries, F. J. Troost, A. A. M. Masclee and K. Schroen, Food-grade micro-encapsulation systems that may induce satiety via delayed lipolysis: A review, Crit. Rev. Food Sci. Nutr., 2017, 57, 2218–2244 CrossRef CAS PubMed.
- P. J. Lillford, The impact of food structure on taste and digestibility, Food Funct., 2016, 7, 4131–4136 RSC.
- D. J. McClements, Enhanced delivery of lipophilic bioactives using emulsions: a review of major factors affecting vitamin, nutraceutical, and lipid bioaccessibility, Food Funct., 2018, 9, 22–41 RSC.
- M. Yokoyama, H. Origasa, M. Matsuzaki, Y. Matsuzawa, Y. Saito, Y. Ishikawa, S. Oikawa, J. Sasaki, H. Hishida, H. Itakura, T. Kita, A. Kitabatake, N. Nakaya, T. Sakata, K. Shimada and K. Shirato, Effects of eicosapentaenoic acid on major coronary events in hypercholesterolaemic patients (JELIS): a randomised open-label, blinded endpoint analysis, Lancet, 2007, 369, 1090–1098 CrossRef CAS.
- J. W. Pike and S. Christakos, Biology and mechanisms of action of the vitamin D hormone, Endocrinol. Metab. Clin. North Am., 2017, 46, 815–843 CrossRef PubMed.
- A. N. Moulas and M. Vaiou, Vitamin D fortification of foods and prospective health outcomes, J. Biotechnol., 2018, 285, 91–101 CrossRef CAS PubMed.
- F. Alshahrani and N. Aljohani, Vitamin D: deficiency, sufficiency and toxicity, Nutrients, 2013, 5, 3605–3616 CrossRef PubMed.
- H. A. Morris, Vitamin D: can you have too much of a good thing in chronic kidney disease?, Kidney Int., 2015, 88, 936–938 CrossRef CAS PubMed.
- S. Pilz, W. Marz, K. D. Cashman, M. E. Kiely, S. J. Whiting, M. F. Holick, W. B. Grant, P. Pludowski, M. Hiligsmann, C. Trummer, V. Schwetz, E. Lerchbaum, M. Pandis, A. Tomaschitz, M. R. Grubler, M. Gaksch, N. Verheyen, B. W. Hollis, L. Rejnmark, S. N. Karras, A. Hahn, H. A. Bischoff-Ferrari, J. Reichrath, R. Jorde, I. Elmadfa, R. Vieth, R. Scragg, M. S. Calvo, N. M. van Schoor, R. Bouillon, P. Lips, S. T. Itkonen, A. R. Martineau, C. Lamberg-Allardt and A. Zittermann, Rationale and Plan for Vitamin D Food Fortification: A Review and Guidance Paper, Front. Endocrinol., 2018, 9, 373 CrossRef PubMed.
- N. Veronese, M. Solmi, M. G. Caruso, G. Giannelli, A. R. Osella, E. Evangelou, S. Maggi, L. Fontana, B. Stubbs and I. Tzoulaki, Dietary fiber and health outcomes: an umbrella review of systematic reviews and meta-analyses, Am. J. Clin. Nutr., 2018, 107, 436–444 CrossRef PubMed.
- A. Lovegrove, C. H. Edwards, I. De Noni, H. Patel, S. N. El, T. Grassby, C. Zielke, M. Ulmius, L. Nilsson, P. J. Butterworth, P. R. Ellis and P. R. Shewry, Role of polysaccharides in food, digestion, and health, Crit. Rev. Food Sci. Nutr., 2017, 57, 237–253 CrossRef CAS PubMed.
- A. Reynolds, J. Mann, J. Cummings, N. Winter, E. Mete and L. Te Morenga, Carbohydrate quality and human health: a series of systematic reviews and meta-analyses, Lancet, 2019, 393, 434–445 CrossRef CAS.
- M. J. Gidley and G. E. Yakubov, Functional categorisation of dietary fibre in foods: Beyond ‘soluble’ vs ‘insoluble’, Trends Food Sci. Technol., 2019, 86, 563–568 CrossRef CAS.
- J. Singh, R. Metrani, S. R. Shivanagoudra, G. K. Jayaprakasha and B. S. Patil, Review on Bile Acids: Effects of the Gut Microbiome, Interactions with Dietary Fiber, and Alterations in the Bioaccessibility of Bioactive Compounds, J. Agric. Food Chem., 2019, 67, 9124–9138 CrossRef CAS PubMed.
- M. M. Wang, S. Wichienchot, X. W. He, X. Fu, Q. Huang and B. Zhang, In vitro colonic fermentation of dietary fibers: Fermentation rate, short-chain fatty acid production and changes in microbiota, Trends Food Sci. Technol., 2019, 88, 1–9 CrossRef CAS.
- X. Qi, F. H. Al-Ghazzewi and R. F. Tester, Dietary Fiber, Gastric Emptying, and Carbohydrate Digestion: A Mini-Review, Starch/Staerke, 2018, 70, 1700346 CrossRef.
- D. So, K. Whelan, M. Rossi, M. Morrison, G. Holtmann, J. T. Kelly, E. R. Shanahan, H. M. Staudacher and K. L. Campbell, Dietary fiber intervention on gut microbiota composition in healthy adults: a systematic review and meta-analysis, Am. J. Clin. Nutr., 2018, 107, 965–983 CrossRef PubMed.
- K. Makki, E. C. Deehan, J. Walter and F. Backhed, The Impact of Dietary Fiber on Gut Microbiota in Host Health and Disease, Cell Host Microbe, 2018, 23, 705–715 CrossRef CAS PubMed.
- B. Zeeb, C. L. Lopez-Pena, J. Weiss and D. J. McClements, Controlling lipid digestion using enzyme-induced crosslinking of biopolymer interfacial layers in multilayer emulsions, Food Hydrocolloids, 2015, 46, 125–133 CrossRef CAS.
- D. Qin, X. Yang, S. Gao, J. Yao and D. J. McClements, Influence of dietary fibers on lipid digestion: Comparison of single-stage and multiple-stage gastrointestinal models, Food Hydrocolloids, 2017, 69, 382–392 CrossRef CAS.
- Y. Li, M. Hu, Y. Du and D. J. McClements, Controlling lipid nanoemulsion digestion using nanolaminated biopolymer coatings, J. Microencapsulation, 2011, 28, 166–175 CrossRef CAS PubMed.
- M. Espert, A. Salvador and T. Sanz, In vitro digestibility of highly concentrated methylcellulose O/W emulsions: rheological and structural changes, Food Funct., 2016, 7, 3933–3942 RSC.
- H. D. Silva, E. Beldíková, J. Poejo, L. Abrunhosa, A. T. Serra, C. M. M. Duarte, T. Brányik, M. A. Cerqueira, A. C. Pinheiro and A. A. Vicente, Evaluating the effect of chitosan layer on bioaccessibility and cellular uptake of curcumin nanoemulsions, J. Food Eng., 2019, 243, 89–100 CrossRef CAS.
- Y. Li and D. J. McClements, Controlling lipid digestion by encapsulation of protein-stabilized lipid droplets within alginate–chitosan complex coacervates, Food Hydrocolloids, 2011, 25, 1025–1033 CrossRef CAS.
- Y. Li and D. J. McClements, Modulating lipid droplet intestinal lipolysis by electrostatic complexation with anionic polysaccharides: Influence of cosurfactants, Food Hydrocolloids, 2014, 35, 367–374 CrossRef.
- A. Mackie, B. Bajka and N. Rigby, Roles for dietary fibre in the upper GI tract: The importance of viscosity, Food Res. Int., 2016, 88, 234–238 CrossRef CAS.
- A. M. R. Pilosof, Potential impact of interfacial composition of proteins and polysaccharides stabilized emulsions on the modulation of lipolysis. The role of bile salts, Food Hydrocolloids, 2017, 68, 178–185 CrossRef CAS.
- M. D. Wilcox, I. A. Brownlee, J. C. Richardson, P. W. Dettmar and J. P. Pearson, The modulation of pancreatic lipase activity by alginates, Food Chem., 2014, 146, 479–484 CrossRef CAS PubMed.
- E. Capuano, The behavior of dietary fiber in the gastrointestinal tract determines its physiological effect, Crit. Rev. Food Sci. Nutr., 2017, 57, 3543–3564 CrossRef CAS PubMed.
- D. Qin, X. Yang, S. Gao, J. Yao and D. J. McClements, Influence of Hydrocolloids (Dietary Fibers) on Lipid Digestion of Protein-Stabilized Emulsions: Comparison of Neutral, Anionic, and Cationic Polysaccharides, J. Food Sci., 2016, 81, C1636–C1645 CrossRef CAS PubMed.
- K. Kurita, Chitin and chitosan:
Functional biopolymers from marine crustaceans, Mar. Biotechnol., 2006, 8, 203–226 CrossRef CAS PubMed.
- M. Rinaudo, Chitin and chitosan: Properties and applications, Prog. Polym. Sci., 2006, 31, 603–632 CrossRef CAS.
- H. K. No, S. P. Meyers, W. Prinyawiwatkul and Z. Xu, Applications of chitosan for improvement of quality and shelf life of foods: A review, J. Food Sci., 2007, 72, R87–R100 CrossRef CAS PubMed.
- B. Bellich, I. D'Agostino, S. Semeraro, A. Gamini and A. Cesaro, “The Good, the Bad and the Ugly” of Chitosans, Mar. Drugs, 2016, 14, 99 CrossRef PubMed.
- M. Borgogna, B. Bellich and A. Cesaro, Marine polysaccharides in microencapsulation and application to aquaculture: “from sea to sea”, Mar. Drugs, 2011, 9, 2572–2604 CrossRef CAS PubMed.
- M. Kumar, R. A. A. Muzzarelli, C. Muzzarelli, H. Sashiwa and A. J. Domb, Chitosan chemistry and pharmaceutical perspectives, Chem. Rev., 2004, 104, 6017–6084 CrossRef PubMed.
- R. Zhang, W. Wu, Z. Zhang, S. Lv, B. Xing and D. J. McClements, Impact of Food Emulsions on the Bioaccessibility of Hydrophobic Pesticide Residues in Co-Ingested Natural Products: Influence of Emulsifier and Dietary Fiber Type, J. Agric. Food Chem., 2019, 67, 6032–6040 CrossRef CAS PubMed.
- M. Espinal-Ruiz, F. Parada-Alfonso, L. P. Restrepo-Sanchez, C. E. Narvaez-Cuenca and D. J. McClements, Impact of dietary fibers [methyl cellulose, chitosan, and pectin] on digestion of lipids under simulated gastrointestinal conditions, Food Funct., 2014, 5, 3083–3095 RSC.
- S. Mun, E. A. Decker, Y. Park, J. Weiss and D. J. McClements, Influence of interfacial composition on in vitro digestibility of emulsified lipids: Potential mechanism for chitosan's ability to inhibit fat digestion, Food Biophys., 2006, 1, 21–29 CrossRef.
- A. Brodkorb, L. Egger, M. Alminger, P. Alvito, R. Assuncao, S. Ballance, T. Bohn, C. Bourlieu-Lacanal, R. Boutrou, F. Carriere, A. Clemente, M. Corredig, D. Dupont, C. Dufour, C. Edwards, M. Golding, S. Karakaya, B. Kirkhus, S. Le Feunteun, U. Lesmes, A. Macierzanka, A. R. Mackie, C. Martins, S. Marze, D. J. McClements, O. Menard, M. Minekus, R. Portmann, C. N. Santos, I. Souchon, R. P. Singh, G. E. Vegarud, M. S. J. Wickham, W. Weitschies and I. Recio, INFOGEST static in vitro simulation of gastrointestinal food digestion, Nat. Protoc., 2019, 14, 991–1014 CrossRef CAS PubMed.
- R. Zhang, W. Wu, Z. Zhang, Y. Park, L. He, B. Xing and D. J. McClements, Effect of the composition and structure of excipient emulsion on the bioaccessibility of pesticide residue in agricultural products, J. Agric. Food Chem., 2017, 65, 9128–9138 CrossRef CAS.
- A. H. Saberi and D. J. McClements, Fabrication of protein nanoparticles and microparticles within water domains formed in surfactant–oil–water mixtures: Phase inversion temperature method, Food Hydrocolloids, 2015, 51, 441–448 CrossRef CAS.
- Y. Tan, J. Liu, H. Zhou, J. Muriel Mundo and D. J. McClements, Impact of an indigestible oil phase (mineral oil) on the bioaccessibility of vitamin D3 encapsulated in whey protein-stabilized nanoemulsions, Food Res. Int., 2019, 120, 264–274 CrossRef CAS.
- D. Guzey and D. J. McClements, Formation, stability and properties of multilayer emulsions for application in the food industry, Adv. Colloid Interface Sci., 2006, 128–130, 227–248 CrossRef CAS PubMed.
- S. Mun, E. A. Decker and D. J. McClements, Influence of droplet characteristics on the formation of oil-in-water emulsions stabilized by surfactant-chitosan layers, Langmuir, 2005, 21, 6228–6234 CrossRef CAS PubMed.
- S. Ogawa, E. A. Decker and D. J. McClements, Production and characterization of O/W emulsions containing cationic droplets stabilized by lecithin-chitosan membranes, J. Agric. Food Chem., 2003, 51, 2806–2812 CrossRef CAS PubMed.
- H. N. Xu, Y. Liu and L. Zhang, Salting-out and salting-in: competitive effects of salt on the aggregation behavior of soy protein particles and their emulsifying properties, Soft Matter, 2015, 11, 5926–5932 RSC.
- J. Combrinck, A. Otto and J. du Plessis, Whey protein/polysaccharide-stabilized emulsions: Effect of polymer type and pH on release and topical delivery of salicylic acid, AAPS PharmSciTech, 2014, 15, 588–600 CrossRef CAS.
- C. Qian, E. A. Decker, H. Xiao and D. J. McClements, Nanoemulsion delivery systems: influence of carrier oil on beta-carotene bioaccessibility, Food Chem., 2012, 135, 1440–1447 CrossRef CAS PubMed.
- P. Sassene, K. Kleberg, H. D. Williams, J. C. Bakala-N'Goma, F. Carriere, M. Calderone, V. Jannin, A. Igonin, A. Partheil, D. Marchaud, E. Jule, J. Vertommen, M. Maio, R. Blundell, H. Benameur, C. J. H. Porter, C. W. Pouton and A. Mullertz, Toward the Establishment of Standardized In Vitro Tests for Lipid-Based Formulations, Part 6: Effects of Varying Pancreatin and Calcium Levels, AAPS J., 2014, 16, 1344–1357 CrossRef CAS PubMed.
- C. Zhang, W. Xu, W. Jin, B. R. Shah, Y. Li and B. Li, Influence of anionic alginate and cationic chitosan on physicochemical stability and carotenoids bioaccessibility of soy protein isolate-stabilized emulsions, Food Res. Int., 2015, 77, 419–425 CrossRef CAS.
- L. G. Gómez-Mascaraque, C. Soler and A. Lopez-Rubio, Stability and bioaccessibility of EGCG within edible micro-hydrogels. Chitosan vs. gelatin, a comparative study, Food Hydrocolloids, 2016, 61, 128–138 CrossRef.
- S. Lv, J. Gu, R. Zhang, Y. Zhang, H. Tan and D. J. McClements, Vitamin E encapsulation in plant-based nanoemulsions fabricated using dual-channel microfluidization: Formation, stability, and bioaccessibility, J. Agric. Food Chem., 2018, 66, 10532–10542 CrossRef CAS PubMed.
- Q. Lin, R. Liang, A. Ye, H. Singh and F. Zhong, Effects of calcium on lipid digestion in nanoemulsions stabilized by modified starch: Implications for bioaccessibility of β -carotene, Food Hydrocolloids, 2017, 73, 184–193 CrossRef CAS.
- D. G. Fatouros, I. Walrand, B. Bergenstahl and A. Mullertz, Colloidal structures in media simulating intestinal fed state conditions with and without lipolysis products, Pharm. Res., 2009, 26, 361–374 CrossRef CAS PubMed.
- T. Nawroth, P. Buch, K. Buch, P. Langguth and R. Schweins, Liposome formation from bile salt-lipid micelles in the digestion and drug delivery model FaSSIF(mod) estimated by combined time-resolved neutron and dynamic light scattering, Mol. Pharm., 2011, 8, 2162–2172 CrossRef CAS PubMed.
|
This journal is © The Royal Society of Chemistry 2020 |
Click here to see how this site uses Cookies. View our privacy policy here.