DOI:
10.1039/C9FO01790A
(Paper)
Food Funct., 2020,
11, 1027-1036
Mallotus oblongifolius extracts ameliorate ischemic nerve damage by increasing endogenous neural stem cell proliferation through the Wnt/β-catenin signaling pathway
Received
6th August 2019
, Accepted 15th November 2019
First published on 20th November 2019
Abstract
Mallotus oblongifolius (MO), an edible medicinal plant from Hainan in China, shows a wide range of bioactivities. The daily consumption of MO or its extracts has been observed to ameliorate ischemic nerve injury. However the mechanisms remain unclear. In this study, the effects of MO both in vitro and in vivo were investigated. The results indicated that MO improved the motor ability, neurosensory ability, balance and grasping ability of mice with ischemic injuries, induced by bilateral common carotid artery ligation (BCCAL). In addition, MO improved the morphology of neurons, resisted the loss of neurons, and enhanced the content of the nestin protein in the cerebral cortex and subgranular zone (SGZ) area. Furthermore, in the oxygen–glucose deprivation and reperfusion (OGD/R) treated cell model, MO could effectively activate the Wnt/β-catenin signaling pathway and promote the proliferation of neural stem cells (NSCs) and increase the protein expression levels of β-catenin and CyclinD1. Our results suggest that Mallotus oblongifolius may be used as nutraceuticals or functional foods to alleviate ischemic nerve damage and promote recovery from ischemic stroke.
1. Introduction
Mallotus oblongifolius [Mallotus oblongifolius (Miq.) Muell-Arg] is a shrub or a small tree of the genus Mallotus of the Euphorbiaceae family and is 2–10 meters tall. It is also known as shankucha, Maocha, or Hecha. The leaves are alternate or opposite, round and ovate, and 5–15 cm long. The leaves are green with corrugated teeth and pointed tip. There are a few transparent pinnate veins on the back of the leaves and the petiole is 1–3 cm long. Residents of Hainan, Guangdong, Guangxi, Yunnan, and Fujian often use the leaves of MO to make tea and drink it every day because of its rich aroma and sweet taste. There are more than 100 million MO tea drinkers in China and Southeast Asia.
MO is also a special kind of medicinal plant with a variety of pharmacological effects, and is commonly used by people of Li nationality in Hainan. MO is named “Hainan Ganoderma lucidum”.1 Recent studies have found that MO is rich in vitamins, ellagic acid, and gallic acid.2 It has been reported to have strong antioxidant and neuroprotective effects.3,4 However, evidence about the mechanism of its therapeutic effects on ischemic nerve injury is still limited.
Ischemic nerve injury refers to the neurological damage caused by the disruption of neurological blood supply to the brain tissue due to various reasons. Common diseases include ischemic stroke and ischemic senile dementia. With the aging of the population, the incidence of ischemic stroke and ischemic senile dementia is increasing.5 Most patients are unable to regain complete neurological function, which not only reduces the quality of life of patients, but also brings heavy financial burden to their family and society. The development of new methods for the treatment of ischemic stroke and ischemic senile dementia is imminent. Studies have shown that ischemic nerve injury is one of the common pathological basis of major diseases such as ischemic stroke and ischemic senile dementia. Restoring the neurological damage caused by ischemia has become the new treatment for stroke sequelae and ischemic dementia.6 It has been reported that cerebral ischemic nerve injury can activate brain-derived neural stem cells which participate in neurological rehabilitation through proliferation, migration, and direct differentiation into functional neurons.7 Finding ways to promote the proliferation of neural stem cells in the brain may contribute to the treatment of ischemic nerve damage.
The classical Wnt/β-catenin signaling pathway was found to play an important role in regulating the proliferation of NSCs.8 β-Catenin and CyclinD1 are the key factors in the Wnt/β-catenin signaling pathway. When the concentration of β-catenin in the cytoplasm is elevated, it can be actively transported to the nucleus to promote the expression of proliferating genes and determine whether the cells are in a proliferative state. CyclinD1 is an important component of the cell cycle regulatory network. CyclinD1 binds to CDK4 or CDK6, allowing cells to enter the S phase and thus promote cell proliferation and division. It has been found that the higher expression of β-catenin and CyclinD1 could promote the proliferation of NSCs.9,10 MO was found to be neuroprotective.11 However, the mechanism of MO in neuroprotection remains unknown.
In this study, we established a mouse model of cerebral ischemia–reperfusion injury to observe the behavioral and brain histopathological changes in mice after treatment with a water extract of MO. We also established a NSC oxygen–glucose deprivation model to detect the proliferation of NSCs after treatment with the water extract of MO and the protein levels of the key genes, β-catenin and CyclinD1, in the Wnt/β-catenin signaling pathway.
2. Materials and methods
2.1 Preparation of the water extract of MO
The leaves of Mallotus oblongifolius were collected in Lingshui, Hainan, China, and decocted with purified water to obtain the water extract of MO. According to the estimated daily intake amount of MO solution (1800 mL day−1 for each person), the medium dose of MO solution for mice was obtained according to the weight change algorithm. In the cell experiment, the dose of the water extract of MO was calculated according to the conversion formula.12
2.2 Reagents and equipment
All antibodies for the western-blot experiment were purchased from Abcam (UK). Nestin primary antibody for immunohistochemistry was purchased from Abcam (UK), FITC-labeled goat anti-rabbit secondary antibody IgG was purchased from Abbkine (USA), and the DAB developer was purchased from Wuhan Bude Biotech Corporation (China). The C17.2 cell line was purchased from Tianjin Saier Biotechnology Corporation (China). The BCA Protein Quantification Kit was purchased from Biyuntian Biotechnology Corporation (China). The BeyoClick™ EdU Cell Proliferation Kit with Alexa Fluor 488 was purchased from Biyuntian Biotechnology Corporation (China). The YLS-20A temperature control heater was purchased from Jinan Yiyan Technology Development Corporation (China), the TE16721 inverted fluorescence microscope was purchased from Olympus (Japan), and the HERA cell 150 cell incubator was purchased from Thermo (USA). The FlexStation 3 full-wavelength screening workstation was purchased from Molecular Devices (USA). The western-blot electrophoresis equipment was purchased from Bio-Rad (USA). The LEICA SP8 laser confocal microscope was purchased from LEICA (Germany), and the Tanon chemiluminescence imager was purchased from Shanghai Tanon Technology Corporation (China).
2.3 Animal model
SPF grade 6-week-old healthy male KM mice (20 ± 2 g) were purchased from Beijing Weitong Lihua Corporation (license number SCXK (Beijing) 2016-0002). All mice were housed in a constant temperature (23.0 ± 2.0 °C) and constant humidity (40–60%) breeding room with a 12 h light/dark cycle, with 2 mice in each cage and adaptive feeding for one week. Experimental protocols were approved by the Animal Care and Use Committee of the Minzu University of China. All animal experimental procedures were performed in accordance with the Guidelines for the Care and Use of Laboratory Animals (NIH publication, revised 1999, No. 3040-2, Bethesda, MD, USA). The model of cerebral ischemia–reperfusion injury was prepared using the bilateral common carotid artery ligation (BCCAL) method.13 All mice were anesthetized with 10% chloral hydrate (300 mg kg−1) and then fixed on the operating table which was heatable to maintain the body temperature of mice at about 37 °C. After making a 0.8–1 cm midline incision in the neck, the common carotid artery and the vagus nerve were separated by about 0.5–1 cm. A zero-wire was placed under the common carotid artery to tie a smooth toothpick and the artery together and block the blood flow for 30 min. Then the toothpick was removed and the blood flow was restored. The skin was sutured after wiping the cut with penicillin solution. Successfully modeled mice were randomly divided into 5 groups (Model, Rg1, H, M and L groups), 14 mice in each group. In the sham-operation group, the common carotid artery and the vagus nerve were separated without ligating the common carotid artery. All mice in the 6 groups were administered continuously for 10 days: (1) sham-operation group: the mice were administered with purified water (Sham); (2) model group: the mice with ischemic brain injury (model) were administered with purified water (Model); (3) control group: the mice with ischemic brain injury (model) were administered with ginsenoside Rg1 solution (0.03 g kg−1 d−1, Rg1); (4) high dose group of MO solution: the mice with ischemic brain injury (model) were administered with MO solution (equivalent to 5.10 g kg−1 d−1 leaves of Mallotus oblongifolius, H) (5) middle dose group of MO solution: the mice with ischemic brain injury (model) were administered with MO solution (equivalent to 1.70 g kg−1 d−1 leaves of Mallotus oblongifolius, M); and (6) low dose group of MO solution: the mice with ischemic brain injury (model) were administered with MO solution (equivalent to 0.57 g kg−1 d−1 leaves of Mallotus oblongifolius, L). Mouse behavioral tests were performed on day 4, day 7, and day 10. After the test, all mice were anesthetized with 10% chloral hydrate. The brain tissues were continuously infused with 4% paraformaldehyde fixative through the left ventricle. The perfusate flowed out from the right atrial appendage, and the perfusion was stopped when the mouse was stiff and the right atrial appendage was transparent. Whole brain tissues of the mice were taken and fixed in 4% paraformaldehyde for 24 h, and then routine pathological analysis and immunohistochemistry experiments were performed.
2.4 Behavioral tests
Open field test was used to detect the central nervous function recovery in mice with ischemia–reperfusion injury.14,15 The mice were placed in the center of a circular container (35 cm in diameter and 17 cm in height) and the number of times a mouse stood up within 3 min was recorded. The more severe the nerve damage in a mouse, the fewer the number of times the mouse stood up. Each mouse was tested three times and the average of three experiments was recorded and calculated. Suspension experiments were used to detect the grasping ability and balance ability of the mice.16 The mice were hung upside down in a cage 20 cm above the ground (the padding was placed under the cage to prevent the mouse from falling). The number of times the mouse fell was recorded. The time more than 3 min was just recorded as 3 min. Each mouse was tested three times and the average of three experiments was recorded and calculated. Hot plate experiments were used to detect the neurosensory ability of mice.17 The mice were placed on a (50 ± 0.1 °C) YLS-20A temperature-controlled heating pad and the movement of licking the back foot was set as the end point. The time more than 3 min was just recorded as 3 min. Each mouse was tested three times and the average of three experiments was recorded and calculated. Behavioral training was performed daily for 3 days before modeling.
2.5 Histological experiment
All mice were deeply anesthetized with 10% chloral hydrate.18 Whole brains of the mice were fixed with 4% paraformaldehyde for 24 h. After dehydration, transparencying with dimethylbenzene, sealing with wax, and other steps, coronal sections of the brains were sliced at 5 μm.19 Some sections were stained with hematoxylin dyeing solution for 15 min and then washed with distilled water and differentiated in 1% hydrochloric acid for 30 s. After the slices turned red, the differentiation was stopped quickly with distilled water. The red staining solution was counterstained for 15 s. After dehydration, transparencying with dimethylbenzene, baking, and sealing, the sections were photographed under a TE16721 inverted fluorescence microscope to observe the pathological conditions of the brain cortex and SGZ area.20
2.6 Immunohistochemistry experiment
Nestin is a hallmark protein of NSCs and can be used for the identification of NSCs.21 Immunohistochemistry experiments were performed according to the protocol:22 Paraffin slides were dewaxed and then hydrated with gradient alcohol. Slides were repaired with citrate buffer in a microwave. Then endogenous peroxidase was blocked with 3% H2O2, and the non-specific antigen was blocked with 5% fetal bovine serum. Rabbit anti-mouse nestin primary antibody (1
:
100 PBS) was added to the slides and the slides were incubated in a wet box in a refrigerator at 4 °C. After overnight incubation, FITC-labeled goat anti-rabbit secondary antibody IgG (1
:
500 PBS) was added dropwise to the slides and the slides were incubated at 37 °C for 30 min. The color was visualized and developed with freshly prepared DAB chromogen for 7 min at room temperature. The cytoplasm of nestin positive cells was brownish yellow. Images were taken at 200× and 400× magnification. The images (400×) were analyzed using the IHC Profiler plugin within ImageJ free software, which measures the intensity of the brown (DAB) color caused by the antigen–antibody reaction. The percentage of only “highly-positive” brown staining was measured, thus reducing the possibility of other colors influencing the brown stain and thus enhancing the accuracy of protein quantification. The percentage of highly-positive staining was averaged from 10 different sections.
2.7 Neural stem cells
C17.2 cells were cultured in a 37 °C, 95% O2, 5% CO2 cell culture incubator. DMEM medium was changed every 24 h for 3 days. The cells were identified by immunocytochemical staining,23 and the experiment proved that C17.2 cells were neural stem cells. The oxygen–glucose deprivation/recovery (OGD/R) model was used to simulate the ischemic brain damage of the brain tissue.24 After the C17.2 cells were cultured for 24 h, the DMEM cell culture medium was replaced with glucose-free DMEM cell culture medium, and cultured in a three-gas incubator with N2, O2 and CO2 concentrations of 94%, 1%, and 5%, respectively, for 6 h. The cells were divided into different groups: (1) the normal group was maintained in normal DMEM culture medium (Normal); (2) the model group was maintained in normal DMEM culture medium (Model); (3) the control group was maintained in DMEM culture medium containing ginsenoside Rg1 (0.01 mg mL−1, Rg1); (4) the high dose group was maintained in DMEM culture medium containing the water extract of MO (equivalent to 1.6 mg mL−1 leaves of Mallotus oblongifolius, H); (5) the medium dose group was maintained in DMEM culture medium containing the water extract of MO (equivalent to 0.53 mg mL−1 leaves of Mallotus oblongifolius, M); (6) the low dose group was maintained in DMEM culture medium containing the water extract of MO (equivalent to 0.18 mg mL−1 leaves of Mallotus oblongifolius, L), and the cells were cultured for 48 h in a cell culture incubator. The normal group was always cultured normally in a cell culture incubator.
2.8 MTT assay
The cell proliferation activity of each group was examined using a CCK-8 kit. C17.2 cells were cultured at a density of 5 × 104 cells per mL in 96-well plates (100 μl per well) with three parallel wells per set. After preparing OGD/R model in the other groups except the normal group, each group was treated with the corresponding drugs for 48 hours. CCK-8 solution (10 μl per well) was added to each well and incubated for 4 h in a cell culture incubator. The absorbance at 450 nm was measured using a FlexStation 3 full-wavelength microplate reader. The ratio of the absorbance value of each group to the normal group was calculated.
2.9 EdU staining
After preparing OGD/R model and the cells were administered with corresponding drugs for 48 hours, detection of EdU incorporation into the DNA was performed using the BeyoClick™ EdU Cell Proliferation Kit with Alexa Fluor 488 according to the manufacturer's instructions. In brief, after treatment with EdU (10 μM) for 2 h, the cells were fixed with 4% paraformaldehyde and then permeabilized with 0.3% Triton X-100 in PBS for 15 min. The cells were washed twice with PBS and fixed in 100 μl click reaction liquid containing Azide 488, click reaction buffer CuSO4, and click additive solution. After a 30 min incubation step at room temperature in the dark, the cells were washed twice with QuickBlock™ immunostaining blocking solution. For subsequent DNA staining, the cells were incubated with DAPI for 20 min.
2.10 Western blotting
Western blotting was performed according to the protocol.25 Neural stem cells of each group were collected, and total protein was extracted with cell lysis buffer containing 1% PMSF. The concentration of total protein in each group was determined using a BCA protein quantification kit and adjusted to a uniform concentration. Protein samples were separated on SDS-PAGE gel, then transferred to PVDF membranes, then the PVDF membranes were blocked with blocking solution and incubated overnight in a 4 °C freezer. After this, the PVDF membranes were incubated with the primary antibody for 2 h at room temperature. After washing with PBS, the membranes were incubated with the HRP-conjugated secondary antibody for 1.5 h at room temperature. The PVDF membranes were visualized by enhanced chemiluminescence and photographed using a Tanon chemiluminescence imager. Data were analyzed using ImageJ software.
2.11 Statistical analysis
Statistical calculations were performed using SPSS software for Windows, version 20.0 (SPSS Inc, Chicago, IL). Data are presented as medians (interquartile range) or
± SD in percent according to the types of variables. One-way ANOVA was performed using GraphPad Prism 6 software for variance. The variance was not transformed. P < 0.05 was considered statistically significant.
3. Results
3.1 Effect of MO on the behavior of mice
Giving the water extract of MO for 10 days to mice with ischemia–reperfusion injury significantly improved their neurobehavioral outcomes (Fig. 1). The results of the open field experiment (Fig. 1A) showed that compared with the sham-operation group, the number of times the mice stood up in the model group was significantly reduced on the 4th day (4.57 ± 3.54 times, P < 0.0001), on the 7th day (5.23 ± 2.27 times, P < 0.0001), and on the 10th day (6.14 ± 2.29 times, P < 0.0001), which means that the modeling is successful. Treatment with MO improved the performance of mice compared to that in the model group, and an improving trend can be observed in all MO groups during 4 to 10 days of treatment. For example, the number in the H group increased from 7.87 ± 0.48 times (P < 0.01) on the 4th day to 10.98 ± 5.32 times (P < 0.01) on the 7th day, and to 13.98 ± 9.44 times (P < 0.01) on the 10th day.
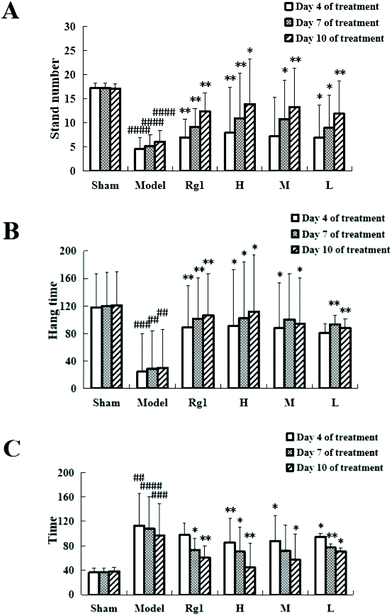 |
| Fig. 1 Effect of MO on the behavior of mice. (A) Open field experiment: the number of times the mice stood up upon treatment with the water extract of MO increased significantly; the number of times the mice stood up upon treatment with Rg1 also increased significantly. (B) Suspension experiment: the suspension time of the mice treated with the water extract of MO was significantly prolonged; the suspension time of the mice treated with Rg1 was also significantly prolonged. (C) Hot plate experiment: the time the mice in the MO groups licked their back foot for the first time was significantly shortened and the time the mice in the Rg1 group licked their back foot for the first time was also significantly shortened. All treatment groups: H – 5.10 g kg−1 d−1; M – 1.7 g kg−1 d−1; L – 0.57 g kg−1 d−1; and Rg1 – 0.03 g kg−1 d−1. Rg1 and H groups n = 13, model group, Rg1 group and M and L groups n = 12. Data are expressed as mean ± SD. ##P < 0.01, ###P < 0.001, ####P < 0.0001 vs. sham group, *P < 0.05, **P < 0.01 vs. model group. | |
The suspension experiment (Fig. 1B) showed that compared with the sham-operation group, the suspension time of the model group was significantly shortened on the 4th day (24.62 ± 41.35 s, P < 0.001), on the 7th day (28.63 ± 38.57 s, P < 0.01), and on the 10th day (30.33 ± 44.24 s, P < 0.001), which means that the modeling is successful. Compared with the model group, the suspension time of the mice in the Rg1, H, M, and L groups was prolonged, and that of the H group was extended to 90.77 ± 69.39 s (P < 0.05) on the 4th day, 101.97 ± 79.39 s (P < 0.05) on the 7th day, and 111.78 ± 82.05 s (P < 0.01) on the 10th day. An improving trend can be observed in the MO groups during 4 to 10 days of treatment.
The hot plate experiment (Fig. 1C) showed that compared with the sham-operation group, the time the mice licked their back foot for the first time in the model group was significantly prolonged, the time on the 4th day was 113.11 ± 23.71 s (P < 0.01), 108.39 ± 47.77 s on the 7th day (P < 0.0001), and 96.82 ± 52.13 s on the 10th day (P < 0.001), which means that the modeling is successful. Compared with the model group, the time in the Rg1, H, M, and L groups was shortened, and that in the H group was shortened to 85.44 ± 15.92 s on the 4th day (P < 0.01), 70.42 ± 31.73 s on the 7th day (P < 0.05), and 44.6 ± 39.77 s on the 10th day (P < 0.01). Mice receiving MO exhibited significant improvements in the hot plate experiment during 4 to 10 days of treatment.
3.2 Effect of MO on the pathology of the brain cortex and SGZ of mice
After treatment with the water extract of MO, the morphology of neurons in the brain cortex (Fig. 2A) and SGZ (Fig. 2B) of mice with ischemia–reperfusion injury was significantly improved. In the sham-operation group, the number of neurons was normal, the cell body was full, the nucleolus was clear, and the shape was regular. The neurons in the corresponding parts of the model group were sparsely arranged, the cell bodies of individual neurons were shrunken, and the structure of some neurons was unclear. The neuron morphology of the Rg1, H, M, and L groups had different degrees of improvement compared the model group.
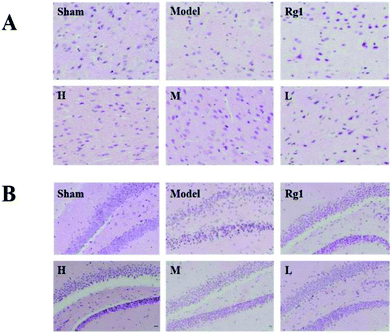 |
| Fig. 2 Effect of MO on the pathology of the brain cortex and SGZ of mice. (A) Neuron state of the brain cortex (400×) and (B) neuron state of the SGZ (400×). Compared with the sham-operation group, the neurons in the model group were sparsely and disorderly arranged, cell bodies were shrunken, some neurons became triangular, and some neuronal structures were unclear. In all MO groups and Rg1 group, the neuron morphology had different degrees of improvement compared to the model group. All treatment groups: H – 5.10 g kg−1 d−1; M – 1.7 g kg−1 d−1; L – 0.57 g kg−1 d−1; and Rg1 – 0.03 g kg−1 d−1. | |
3.3 Effect of MO on the nestin protein in the brain cortex and SGZ of mice
Mice with ischemia–reperfusion injury that were treated with the water extract of MO for 10 days showed a significantly higher content of the nestin protein in the brain cortex (Fig. 3A) and SGZ (Fig. 3B). The cells with brownish yellow cytoplasm were nestin positive cells. Compared with the sham-operation group, the number of nestin positive cells in the model group in the brain cortex was increased (P < 0.05). The number of nestin positive cells in the brain cortex of the H, M and L groups was significantly higher than that of the model group (P < 0.05; P < 0.01), and was the same as that of the Rg1 group (P < 0.05) (Fig. 3C). Compared with the sham-operation group, the number of nestin positive cells in the model group in the SGZ was increased (P < 0.01). The number of nestin positive cells in the SGZ of the Rg1, H, M and L groups was significantly higher than that of the model group (P < 0.05) (Fig. 3D).
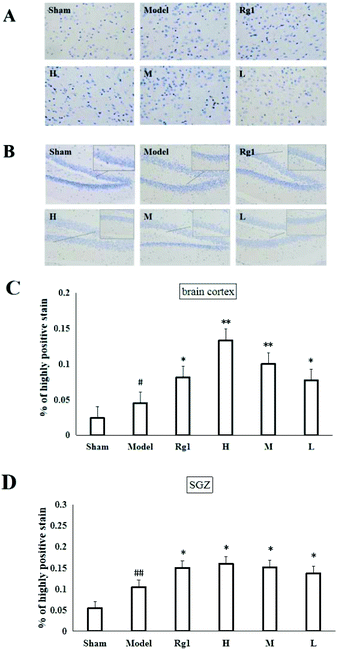 |
| Fig. 3 Effect of MO on the nestin protein in the brain cortex and SGZ of mice. (A) Nestin protein content in the brain cortex (200×) and (B) nestin protein content in the SGZ (200×). Semi-quantitative analysis of the expression of nestin in the brain cortex (C) and SGZ (D). Compared with the sham-operation group, the model group showed increased number of nestin positive cells. Compared with the model group, the number of nestin positive cells in the brain cortex and SGZ of mice increased significantly in all MO groups and Rg1 group. All treatment groups: H – 5.10 g kg−1 d−1; M – 1.7 g kg−1 d−1; L – 0.57 g kg−1 d−1; and Rg1 – 0.03 g kg−1 d−1. | |
3.4 Effect of MO on the proliferation of NSCs
Treatment with the water extract of MO for 48 h significantly promoted neural stem cell proliferation in the MO groups (Fig. 4). NSCs were identified by the expression of nestin (Fig. 4B), and the NSCs were in a healthy condition (Fig. 4A). The effect of the water extract of MO on NSC number is shown in Fig. 4C and D. Five visual fields (400×) were randomly selected in each group, the number of cells in each visual field was recorded, and the average number of cells was calculated. The number of cells was 277.5 ± 59.36 in the normal group, but only 34.25 ± 20.75 in the model group (P < 0.001). However, compared with the model group, the number of cells in the Rg1 and MO groups was higher (Fig. 4D). The cell viability was 100% in the normal group, but only 50.00% ± 14.85% in the model group. The cell viability of the cells treated with the water extract of MO was increased. The cell viability was 80.67%±30.35% in the H group and 67.17% ± 12.42% in the Rg1 group (Fig. 4E).
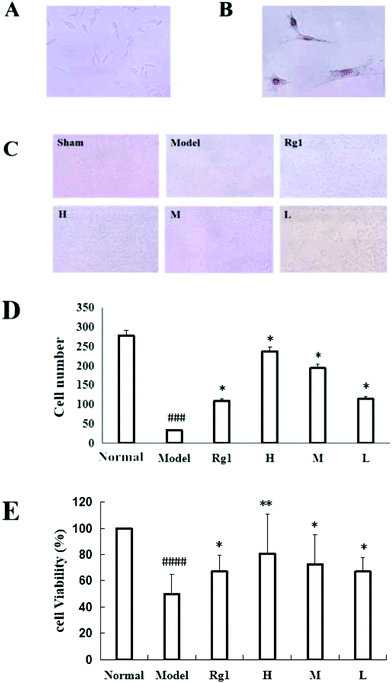 |
| Fig. 4 Effect of MO on the proliferation of NSCs. (A) C17.2 cell growth state (400×). (B) Immunocytochemistry identified C17.2 cells as neural stem cells (400×). (C, D) The number of cells administered with MO for 48 h after OGD/R. (E) The viability of cells administered with MO for 48 h after OGD/R. The number of cells in the model group was significantly lower than that in the normal group. All MO groups showed significant cell proliferation. The cells also proliferated significantly upon treatment with Rg1 for 48 h compared with the model group. All treatment groups: H – 1.6 mg mL−1; M – 0.53 mg mL−1; L – 0.18 mg mL−1; and Rg1 – 0.01 mg mL−1. Data are expressed as mean ± SD. ####P < 0.0001 vs. normal group, *P < 0.05, **P < 0.01 vs. model group (n = 6). | |
EdU staining showed similar results. The number of cells in the treated groups was decreased compared with the normal group because of OGD/R. However, the number of neoproliferative cells in the MO groups was more than that in the model group (P < 0.05; Fig. 5A and B), compared with the model group, the percentage of EdU positive cells increased significantly in the MO groups, and in the Rg1 group. Taken together, the results suggested that MO can promote NSC proliferation.
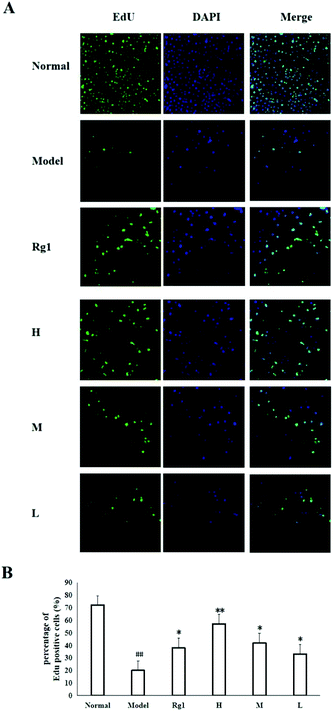 |
| Fig. 5 Effect of MO on the EdU positive cells. (A and B) MO significantly increased EdU positive cells. Cells were treated with 10 μM EdU for 2 hours. (A) Representative images showing that the cells treated with MO show more numbers of EdU positive cells than the model group. EdU (blue) and DAPI (green). (B) Quantitative data showing that MO decreases the numbers of EdU-labeled cells. All treatment groups: H – 1.6 mg mL−1; M – 0.53 mg mL−1; L – 0.18 mg mL−1; and Rg1 – 0.01 mg mL−1. Data are expressed as mean ± SD. ##P < 0.01 vs. normal group, *P < 0.05, **P < 0.01 vs. model group (n = 6). | |
3.5 Effect of MO on the expression of β-catenin and CyclinD1 in NSCs
Treating C17.2 cells with the water extract of MO for 48 h significantly increased the expressions of β-catenin and CyclinD1 (Fig. 6A and B). Compared with the normal group, the β-catenin protein content in the model group was significantly higher (0.35 ± 0.13 vs. 0.51 ± 0.03, P < 0.05), and the β-catenin protein content in the Rg1, H, M and L groups was also significantly higher than that of the model group. The β-catenin protein content in the H group increased to 1.07 ± 0.45 (Fig. 6A and C) compared with that in the normal group, the CyclinD1 protein content in the model group was significantly higher (0.15 ± 0.02 vs. 0.22 ± 0.02, P < 0.05), and the CyclinD1 protein content in the Rg1, H, M, and L groups was also significantly increased compared to that in the model group. The CyclinD1 protein content in the H group increased to 1.09 ± 0.70.
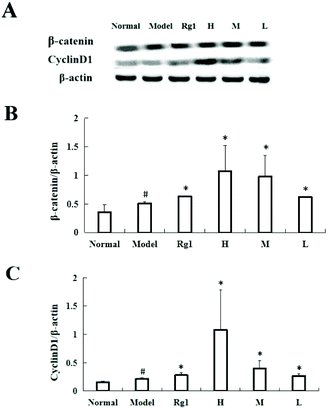 |
| Fig. 6 Effect of MO on the expression of β-catenin and CyclinD1 in NSCs. (A and B) The protein of β-catenin in the MO groups increased significantly, and also in the Rg1 group. The histogram represented the ratio of the β-catenin protein content to β-actin. Data were expressed as mean ± SD. #P < 0.05 vs. normal group, *P < 0.05 vs. model group (n = 6). (A and C) The CyclinD1 protein in the MO groups increased significantly, and also increased significantly in the Rg1 group. The histogram showed the ratio of the CyclinD1 protein content to β-actin. Data were expressed as mean ± SD. #P < 0.05 vs. normal group, *P < 0.05 vs. model group (n = 5). All treatment groups: H – 1.6 mg mL−1; M – 0.53 mg mL−1; L – 0.18 mg mL−1; and Rg1 – 0.01 mg mL−1. | |
4. Discussion
In this study, we investigated the therapeutic effects of the water extract of MO on mice with ischemia–reperfusion injury. The experimental results showed that the water extract of MO can significantly improve the motor function, neurosensory ability, balance, and grasp ability of the mice with ischemia–reperfusion injury. At the same time, the water extract of MO restored the damaged neurons and increased the nestin protein content in the cerebral cortex and SGZ. We also explored the effect of the water extract of MO on the proliferation of NGD/R model NSCs. The CCK-8 technique was used to detect the activity of NSCs after treatment with the water extract of MO for 48 h. The activity of NSCs in the model group was significantly lower than that in the MO groups. Cell proliferation was detected by EdU staining, and MO increased the number of neoproliferative cells after anoxic-reoxygenation. The protein levels of the key proliferative genes β-catenin and CyclinD1 were detected by western blotting, and the protein level of the MO groups was found to be significantly increased. The results showed that MO improved the nerve damage of the ischemic brain tissue and promoted the proliferation of NSCs through the Wnt/β-catenin signaling pathway.
In recent years, natural products and functional foods have aroused great interest in the treatment of ischemic nerve damage. As a kind of tea of the people of Hainan, MO had a significant curative effect on the treatment of ischemic nerve injury, which was also in line with the theory of “medicine and food homology” in traditional Chinese medicine.
The aim of the present study was to explore the molecular mechanism of MO in treating ischemic nerve injury. Neuronal death was one of the most important features of ischemic nerve injury. Therefore, supplementation of missing neurons was one of the effective strategies for the treatment of ischemic nerve injury.26–28 In fact, when cerebral ischemia leads to nerve damage, orthotopic NSCs in the brain can participate in nerve regeneration through proliferation and further differentiation into neurons and thus restore brain tissue function.29 Therefore, understanding how MO promotes the specific pathway of NSC proliferation is the key to understanding how MO ameliorates ischemic nerve injury.
The proliferation of NSCs is an extremely complex process that is regulated by various factors and signaling pathways. Among them, it was found that the Wnt/β-catenin signaling pathway was the most classical signaling pathway and played an important role in regulating the proliferation of NSCs.30 After the transmembrane receptor Frizzled was activated by Wnt, Dvl and Axin were formed in its intracellular segment in the cytoplasm. Activated Dvl becomes an inhibitor of the GSK-3β–Axin–APC complex to prevent the degradation of β-catenin.31 β-catenin accumulates in the cytoplasm and then translocates into the nucleus and binds to the cAMP response element binding protein (CREB) and TCF (binding factor), positively regulating the transcription of target genes that promote the proliferation of NSCs. On the other hand, β-catenin bound to P300 (cAMP response element binding protein homolog) and TCF (binding factor) positively regulated the transcription of target genes that promote NSC differentiation. One is stronger and the other is weaker, the stronger determines whether NSCs are proliferating or differentiating.32 β-Catenin was a core factor in the Wnt/β-catenin signaling pathway and played an important role in determining whether a cell was in a proliferative state.33 β-Catenin used Axin or APC as a carrier to transport to the nucleus from the cytoplasm by active transport, and played a decisive role in the expression of downstream proliferating genes by regulating its quantity and state in the cytoplasm.34 CyclinD1 is a downstream proliferating gene of the Wnt/β-catenin signaling pathway. Cyclin has a positive regulatory effect on cyclin-dependent kinase (CDK) and a negative regulatory effect on the cyclin-dependent kinase inhibitor (CKI). CyclinD1 binds to CDK4 or CDK6 to allow the cells to enter the S phase, promoting cell division and proliferation35–37 (Fig. 7).
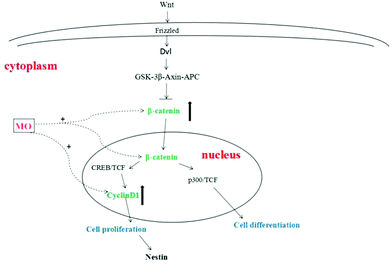 |
| Fig. 7 Network of the signaling pathway in NSC proliferation. The Wnt/β-catenin signaling pathway was the key pathway for NSC proliferation, and the key genes were upregulated by treatment with the water extract of MO. β-Catenin accumulated in the cytoplasm, translocated to the nucleus and then regulated the expression of proliferating genes. CyclinD1 was a proliferating gene in the middle and lower reach of the Wnt/β-catenin signaling pathway. CyclinD1 combined with CDK4 or CDK6 to make the cells enter the S phase and promote cell proliferation. | |
The therapeutic effect of MO on ischemic nerve injury may also be the result of comprehensive regulation of multiple signaling pathways. The concatenation of the Wnt signaling pathway with other signaling pathways warrants further investigation. In addition, according to research studies, MO contains not only ellagic acid, but also epigallocatechin, epigallocatechin gallate, epicatechin gallate, tea polyphenols and so on.2,9,38 Many active ingredients such as ellagic acid, epigallocatechin-3-gallate, and tea polyphenols can promote NSC proliferation.3,39,40 The therapeutic effect of MO on ischemic nerve injury may also be the result of comprehensive regulation of multiple active components. These multiple components and multiple mechanisms may also be the characteristics and advantages of food therapy.
In summary, we found that Hainan folk tea MO had the potential to improve ischemic nerve injury. MO could activate the Wnt/β-catenin signaling pathway to promote the proliferation of orthotopic NSCs in the brain and thus restore the neurological function of the ischemic brain tissue through nerve regeneration. These findings suggest that MO can ameliorate ischemic nerve injury by promoting the proliferation of orthotopic NSCs in the brain by activating the Wnt/β-catenin signaling pathway.
5. Conclusions
This study demonstrated for the first time that MO promoted the proliferation of orthotopic NSCs in the brain by regulating the Wnt/β-catenin signaling pathway to ameliorate ischemic nerve injury. This study provided new insights into how MO plays a neuroprotective role. The results of the study indicate that MO is a kind of potential functional food or drug for the treatment of ischemic nerve injury.
Abbreviations
APC | Adenomatous polyposis coil |
CREB | cAMP Reaction element binding protein |
Dvl | Dishevelled |
EdU | 5-Ethynyl-2′-deoxyuridine |
GSK-3β | Glycogen synthase kinase 3 times |
MO |
Mallotus oblongifolius
|
NSCs | Neural stem cells |
p300 | cAMP Reaction element binding protein homologue |
SGZ | Subgranular zone |
TCF | Transcription factors |
Conflicts of interest
The authors declare no conflict of interest.
Acknowledgements
This work was supported by the Natural Science Foundation of China (81774006 and 81803810); the Key Research and Development Projects of the Ministry of Science and Technology (2017YFC1704000); the Independent Research Project for Doctoral Students of Minzu University of China (BBZZKY-2019090); and Fund of Xizang Minzu University (324011809906).
References
- G. M. Liu, J. L. Li, X. J. Wang and Q. X. He, Studies on the ethnobotany of Hainan mallotus oblongifolius, J. Hainan Norm. Univ., Nat. Sci., 2007, 20, 167–172 CrossRef.
- C. N. Mao, W. L. Yang, W. Y. Lai and J. Q. Zhang, Determination of gallic acid in shankucha of Li nationaly medicinal material by HPLC, China Pharm., 2009, 20, 198–199 CAS.
- Q. S. Liu, S. R. Li, K. Q. Li, X. Y. Yin and Z. R. Pang, Ellagic acid improves endogenous neural stem cells proliferation and neurorestoration through Wnt/β-catenin signaling in vivo and in vitro, Mol. Nutr. Food Res., 2017, 61, 1600587 CrossRef.
- Y. L. Liu and L. B. Lin, Effects of extract of hainan mountain bitter tea on learning and memory ability of aging mice, Chin. J. Trop. Med., 2012, 12, 21–23 Search PubMed.
- S. X. Zhang, W. Tian, Y. D. Ren, M. Y. Wang and S. M. Liu, Application and research of traditional Chinese medicine in neurodegenerative diseases, J. Tradit. Chin. Med., 2015, 43, 126–129 Search PubMed.
- J. Liu, K. Solway, R.O Messing and F.R Sharp, Increased neurogenesis in the dentate gyrus after transient global ischemia in gerbils, J. Neurosci., 1998, 18, 7768–7778 CrossRef CAS.
- Q. Jiang, L. Chen, K. Huang, R. Wang and Z. H. Wu, Effect of Notchsignaling pathway on proliferation and differentiation of neural stem cells after cerebral ischemia preconditioning in rats, Guangdong Med. J., 2008, 39, 2718–2722 Search PubMed.
- X. H. Zhang, J. D. Zhu, T. T. Long, J. J. Wang and C. S. Kang, Effects of Wnt/β-catenin signal pathway on hippocampal neural stem cells in mice, J. Anhui Med. Univ., 2019, 54, 44–49 Search PubMed.
- J. Song and Z. Y. Zhang, Research progress on chemical composition and pharmacological action of shankucha, Chin. J. Hosp. Pharm., 2016, 36, 152–156 CAS.
- W. P. Yan and J. L. Li, Research progress of mallotus oblongifolius in Hainan, Chin. Agric. Sci. Bull., 2016, 32, 200–204 Search PubMed.
- W. L. Gu, L. H. Tan, C. Y. Hao, H. Wang and H. Y. Zhu, Research progress and development and utilization status of mallotus oblongifolius, Sci.Trop. Agric., 2015, 35, 28–35 CrossRef.
-
S. tu Z. Qiang, Cell Culture [M], World Book Publishing House, Beijing, 2nd edn, 2007 Search PubMed.
- R. Cai, B. Liu, Q. H. Gong, B. Huang, J. S. Shi and F. Li, Icariin improves the learning and memory function of rats with bilateral common carotid artery ligation by promoting angiogenesis, Chin. J. New Drugs Clin. Med., 2016, 35, 287–293 CAS.
- X. X. Zhao, Z. S. Li and Y. Wang, Comparison of behavioral test methods in mice with focal cerebral ischemia, Chin. J. Rehabil. Med., 2016, 31, 821–824 Search PubMed.
-
X. Y. Niu, Behavioral pharmacological experimental methods. Pharmacological Experimental Methodology [M], People's Health Publishing House, Beijing, 2nd edn, 1994, pp. 641–650 Search PubMed.
- K. Y. Fan, L. N. Zhu, W. L. Zhang, H. R. Zhang, Y. Zhang and L. Ma, Comparison of subacute and chronic models of Parkinson's disease induced by MPTP in mice, Chin. J. Gerontol., 2017, 37, 4–6 Search PubMed.
- M. R. Sapio, M. J. Iadarola, D. M. LaPaglia, T. Lehky, A. E. Thurm, K. M. Danley, S. R. Fuhr, M. D. Lee, A. E. Huey, S. J. Sharp, J. M. Tsao, J. A. Yanovski, A. J. Mannes and J. C. Han, Haploinsufficiency of the brain-derived neurotrophic factor gene is associated with reduced pain sensitivity, Pain, 2019, 160, 1070–1081 CrossRef CAS.
- S. C. Li, L. N. Ma, F. J. Li, W. Hao, L. Zhao and Y. K. Li, Comparison of anesthetic effects of different concentrations of chloral hydrate on rats, China Pharm., 2014, 23, 22–23 Search PubMed.
- C. Q. Qu, W. Zou and Y. J. Zhang, A method for making paraffin sections of high quality animal tissues, Lab. Sci., 2018, 21, 75–78 Search PubMed.
- S. K. Mohamed, A. A. E. Ahmed, E. M. El Morsy and S. Nofal, The protective effect of zeranol in cerebral ischemia reperfusion via p-CREB overexpression, Life Sci., 2019, 217, 212–221 CrossRef CAS.
- W. W. Wang, J. H. Han, L. Wang and T. H. Bao, Scutellarin may alleviate cognitive deficits in a mouse model of hypoxia by promoting proliferation and neuronal differentiation of neural stem cells, Iran. J. Basic Med. Sci., 2017, 20, 272–279 Search PubMed.
- T. Adell, S. Barberán, M. Sureda-Gómez, M. Almuedo-Castillo, N. de Sousa and F. Cebrià, Immunohistochemistry on Paraffin-Embedded Planarian Tissue Sections, Methods Mol. Biol., 2018, 1774, 367–378 CrossRef CAS.
- M. Lazzari, S. Bettini and V. Franceschini, Immunocytochemical characterisation of ensheathing glia in the olfactory and vomeronasal systems of Ambystoma mexicanum (Caudata: Ambystomatidae), Brain Struct. Funct., 2016, 221, 955–967 CrossRef CAS.
- F. Li, J. Liang and D. F. Tang, Brahma-related gene 1 ameliorates the neuronal apoptosis and oxidative stress induced by oxygen-glucose deprivation/reoxygenation through activation of Nrf2/HO-1 signaling, Biomed. Pharmacother., 2018, 108, 1216–1224 CrossRef CAS.
- S. M. Davis, L. A. Collier, E. A. Foran, C. C. Leonardo, C. T. Ajmo Jr. and K. R. Pennypacker, Neuroprotective activity of leukemia inhibitory factor is relayed through myeloid zinc finger-1 in a rat model of stroke, Metab. Brain Dis., 2019, 34, 631–640 CrossRef CAS.
- C. W. Qiu, Z. Y. Liu, F. L. Zhang, L. Zhang, F. Li, S. Y. Liu, J. Y. He and Z. C. Xiao, Post-stroke gastrodin treatment ameliorates ischemic injury and increases neurogenesis and restores the Wnt/β-Catenin signaling in focal cerebral ischemia in mice, Brain Res., 2019, 1712, 7–15 CrossRef CAS.
- S. J. Zhang, R. L. Wang, H. P. Zhao, Z. Tao, J. C. Li, F. Ju, Z. P. Han, Q. F. Ma, P. Liu, S. B. Ma, G. D. Cao and Y. M. Luo, MEPO promotes neurogenesis and angiogenesis but suppresses gliogenesis in mice with acute ischemic stroke, Eur. J. Pharmacol., 2019, 849, 1–10 CrossRef CAS.
- Q. S. Liu, R. Deng, S. Li, X. Li, K. Q. Li, G. Kebaituli, X. Li and R. Liu, Ellagic acid protects against neuron damage in ischemic stroke through regulating the ratio of Bcl-2/Bax expression, Appl. Physiol., Nutr., Metab., 2017, 42, 855–860 CrossRef CAS.
- Q. Jiang, L. Chen, K. Huang, R. Wang and Z. H. Wu, Effect of Notch signaling pathway on proliferation and differentiation of neural stem cells after cerebral ischemia preconditioning in rats, Guangdong Med. J., 2008, 39, 2718–2722 Search PubMed.
- B. Y. Sheng, Y. J. Jiang, Y. Li, X. M. Ren, W. Jiao, Z. M. Wang, J. Y. Tian, J. H. Wu and J. Wang, Effects of Wnt/β-catenin signal regulation on damage of neural stem cells induced by Aβ1-42 in the hippocampus, Heilongjiang Med. Sci., 2017, 40, 101–103 Search PubMed.
- J. F. Zheng and X. F. Fu, Research status of main physiological and pathological effects of WNT signaling pathway, J. Neuroanat., 2014, 30, 490–494 CAS.
- S. R. Li, R. Deng and Q. S. Liu, Research progress on Wnt signaling pathway of neural stem cell proliferation and intervention of traditional Chinese medicine, Int. J. Pharm. Res., 2017, 44, 45–48 Search PubMed.
- Y. Zhang, S. Chen, C. Wei, G. O. Rankin, X. Ye and Y. C. Chen, Dietary compound proanthocyanidins from Chinese bayberry (Myrica rubraSieb. et Zucc.) leaves attenuate chemotherapy-resistant ovarian cancer stem cell traits via targeting the Wnt/β-catenin signaling pathway and inducing G1 cell cycle arrest, Food Funct., 2018, 9, 525–533 RSC.
- C. X. Zhang, Y. J. Guan, Y. C. Chen, L. Yu and X. J. Li, Research progress on the relationship between classical Wnt signaling pathway and neurodegenerative diseases, Adv. Anat. Sci., 2013, 19, 96 CAS.
- P. T. Chen, X. Q. Zhou, W. F. Liu, Z. B. Cao and Y. W. Chen, Effect of Danlong Xingnao recipe on proliferation and Frizzled1, Dvl1, CyclinD1 expression of neural stem cells in hippocampus of rats with cerebral ischemia-reperfusion, Pharmacol. Clin. Pract. Tradit. Chin. Med., 2016, 32, 120–124 Search PubMed.
- S. Afrin, F. Giampieri, M. Gasparrini, T. Y. Forbes-Hernández, D. Cianciosi, P. Reboredo-Rodriguez, A. Amici, J. L. Quilesc and M. Battino, The inhibitory effect of Manuka honey on human colon cancer HCT-116 and LoVo cell growth. Part 1: the suppression of cell proliferation, promotion of apoptosis and arrest of the cell cycle, Food Funct., 2018, 9, 2145–2157 RSC.
- X. Wu, M. Y. Song, P. J. Qiu, F. Li, M. Q. Wang, J. K. Zheng, Q. Wang, F. Xua and H. Xiao, A metabolite of nobiletin, 4′-demethylnobiletin and atorvastatin synergistically inhibits human colon cancer cell growth by inducing G0/G1 cell cycle arrest and apoptosis, Food Funct., 2018, 9, 87–95 RSC.
- S. R. Li, R. Deng, Q. S. Liu and Y. Cheng, Simultaneous determination of three active components in compound Zhegu oral liquid of Li nationality by HPLC, Chin. Med., 2019, 30, 1113–1115 Search PubMed.
- T. Itoh, M. Imano, S. Nishida, M. Tsubaki, N. Mizuguchi, S. Hashimoto, A. Ito and T. Satou, (-)-Epigallocatechin-3-gallate increases the number of neural stem cells around the damaged area after rat traumatic brain injury, J. Neural Transm., 2012, 119, 877–890 CrossRef CAS.
- K. J. Seong, H. G. Lee, M. S. Kook, H. M. Ko, J. Y. Jung and W. J. Kim, Epigallocatechin-3-gallate rescues LPS-impaired adult hippocampal neurogenesis through suppressing the TLR4-NF-κB signaling pathway in mice, Korean J. Physiol. Pharmacol., 2016, 20, 41–51 CrossRef CAS.
Footnote |
† These authors contributed equally to this work. |
|
This journal is © The Royal Society of Chemistry 2020 |