DOI:
10.1039/C9FO01570A
(Paper)
Food Funct., 2020,
11, 977-991
The regulatory effects of phytosterol esters (PSEs) on gut flora and faecal metabolites in rats with NAFLD†
Received
17th July 2019
, Accepted 11th November 2019
First published on 14th November 2019
Abstract
Recent studies have shown that the occurrence and progression of nonalcoholic fatty liver disease (NAFLD) can be aggravated by dysregulation of intestinal flora. We previously found that phytosterol esters (PSEs) could effectively prevent the progression of NAFLD. Here, we further investigated the regulatory effect that PSEs have on gut flora and faecal metabolites in rats with NAFLD. Adult SD (Sprague Dawley) rats were randomized into four groups: the normal chow diet (NC), high-fat diet (HFD), low-dose PSE (0.05 g per 100 g BW, PSEL) and high-dose PSE (0.10 g per 100 g BW, PSEH) groups. PSEs were intragastrically administered once a day for 12 consecutive weeks. Our work indicated that high-dose PSE treatment effectively inhibited the increase in liver and abdominal fat indexes (P < 0.01) and hepatic lipids (P < 0.01); a high dose PSE treatment effectively corrected the HFD-induced intestinal flora imbalance by changing the diversity. The relative abundances of the four phyla (Firmicutes, Proteobacteria, Actinobacteria and Verrucomicrobia) and partial bacteria at the genus level (Faecalibacterium, Akkermansia, etc.) in the PSEH group were closer to those in the NC group. High-dose PSE intervention significantly increased the relative abundance of Bacteroidetes and Anaerostipes. Compared with the HFD, PSEH treatment significantly decreased the ionic strengths of bile acid metabolism products (P < 0.05), which were positively correlated with hepatic steatosis. In conclusion, PSE treatment exerts a beneficial effect on NAFLD that is associated with its regulatory action on intestinal flora and faecal metabolites, which might present a new opportunity to develop effective and safe preventive strategies against NAFLD.
1 Introduction
With the increasing prevalence of metabolic syndrome and obesity, nonalcoholic fatty liver disease (NAFLD) has become the most common cause of chronic liver disease worldwide that threatens human health.1 The prevalence of NAFLD is approximately 30% in Western countries and 12–24% in Asian countries.2 NAFLD, defined as a spectrum of liver pathology, is initiated by simple steatosis and may progress to nonalcoholic steatohepatitis (NASH), cirrhosis and eventually hepatocellular carcinoma.3 Therefore, understanding the disease pathology mechanisms and putting forward practical preventive and therapeutic strategies for NAFLD are important.
Recent studies have shown that in addition to the genetic background, intestinal microbiota dysbiosis is one of the critical factors in the development of NAFLD since these intestinal bacteria play important roles in regulating energy balance and fat deposition.4 The dysregulation of intestinal flora may aggravate the occurrence and progression of NAFLD via abnormal levels of intestinal flora metabolites and their negative effect on intestinal permeability.5,6 Therefore, restoring (or maintaining) gut homeostasis with functional components in food may present an effective and safe preventive or therapeutic strategy for NAFLD patients.
Phytosterols or plant sterols are active ingredients that are widely found in a variety of vegetable oils, nuts and plant seeds. The esterified form of phytosterols, phytosterol esters (PSEs), exhibits good solubility and bioavailability. Studies have shown that phytosterols have regulatory effects on blood lipids and anticancer effects.7 Our previous animal experiment found that PSE treatment could significantly reduce the levels of serum low-density lipoprotein cholesterol (LDL-C) and hepatic triglyceride (TG), total cholesterol (TC), and free fatty acids (FFAs) as well as the levels of some cytokines, including TGF-β, IL-6, IL-10 and C-reactive protein (CRP). Furthermore, oxidative stress was ameliorated by PSE treatment by increasing superoxide dismutase (SOD) activity and decreasing xanthine oxidase (XOD) activity and malondialdehyde (MDA) levels. PSE intervention could effectively ameliorate the “two-hit” pathological process of NAFLD partly due to the upregulation of hepatic PPAR-α and PPAR-γ mRNA levels and LXR-α and ELOVL-2 expression.8 In the present study, the influence of PSEs on the intestinal microbiota and faecal metabolites was explored to further elucidate their mechanisms related to the prevention of NAFLD.
2 Materials and methods
2.1 Chemical composition of phytosterol esters
The PSE product was supplied by BASF China Ltd (Shanghai, China). PSEs (total PSE and phytosterol content ≥97%: PSE content ≥91% and free phytosterol content ≤6%) had the following chemical composition: β-sitosterol: 42.0–55.0%, campesterol: 20.0–29.0%, stigmasterol: 12.0–23.0%, brassicasterol ≤6%, D5-oats sterol ≤4%, D7-stigmasterol ≤2%, D7-oats sterol ≤2%, cholesterol ≤2%, and other ≤5%.
2.2 Experimental animals and treatment
Six-week-old male Sprague Dawley (SD) rats (170 ± 10 g) were purchased from the Slack Laboratory Animal Co., Ltd [SCXK(Hu)2012-0002, Shanghai, China] and maintained in a ventilated rack system. Food and water were provided ad libitum throughout the entire study. After a week of adaptive feeding, the rats were randomly allocated into the following four groups containing animals with similar mean body weights (BWs): a normal chow diet control group (NC, n = 6), a high-fat diet group (HFD, n = 6), a low-dose PSE treatment group (PSEL, n = 6) and a high-dose PSE treatment group (PSEH, n = 6). The rats in the NC group were fed a standard diet, and those in the other three groups were fed a HFD. At the same time, SD rats in the two PSE treatment groups were orally administered PSE-fortified skimmed milk (1 mL per 100 g BW) once a day for 12 consecutive weeks. The rats in the PSEH and PSEL groups were administered 0.05 g per 100 g BW and 0.10 g per 100 g BW PSE, respectively (the PSE intervention dose was the equivalent of 3 g d−1 and 6 g d−1, respectively, in humans). As a control, regular skimmed milk was given to the NC and HFD groups.
The normal diet contained crude protein (≧20%), crude fibre (≦5%), crude fat (≧4%), moisture (≦10%), ash (≦8%), calcium (1–1.8%) and phosphorus (0.6–1.2%). The HFD was purchased from FBSH Biotechnology Co. Ltd and was prepared by mixing the normal diet (52.65%) with 21% lard (wt/wt), 10% sucrose (wt/wt), 10% casein, 2.7% maltodextrin, 1.9% premix compound, 1.25% cholesterol and 0.5% cholic acid.
All rats were inspected daily, and the BWs of all animals were recorded once a week. The experimental conditions and procedures were approved by the Shanghai Jiao Tong University Institutional Animal Care and Use Committee (Shanghai, China) and were consistent with the National Institutes of Health Guide for the Care and Use of Laboratory Animals (GB/T 35892-2018).
2.3 Sample collection
After 12 weeks of the intervention, the rats were fasted overnight, weighed, and anaesthetized with 2% sodium pentobarbital (0.2 ml per 100 g) according to the recommendations for experimental animals. Blood samples were drawn from the abdominal aorta, and serum was prepared by low-speed centrifugation (3509g, 10 min, 4 °C) to separate the serum. Livers and bilateral perirenal lipids were rapidly removed and weighed. The fresh liver samples from the same lobe and a similar site in each rat were immediately fixed in 4% paraformaldehyde, and the other tissues were flash-frozen in liquid nitrogen. Faecal samples were collected from all rats every morning for one week before the end of the experiment, and snap frozen in liquid nitrogen within minutes. All of the samples were kept at −80 °C until analysis.
2.4 Determination of the liver index and abdominal fat index
The rats were weighed before sacrifice. The liver and bilateral perirenal fat were carefully removed at the time of sacrifice and washed with normal saline. The excess water was absorbed with clean filter paper, and the mass of tissues and organs was accurately weighed using a balance. The organ index was calculated using the following formulas: liver index (mg g−1) = liver weight/rat weight; and abdominal fat index (mg g−1) = bilateral perirenal fat weight/rat weight.
2.5 Analysis of the hepatic lipid content
The hepatic lipid content of each rat was analysed according to9 Folch J's method with partial modification. Briefly, 10 ml chloroform/methanol (2
:
1) was added into 1 g of liver tissue. The mixture was then homogenized, sonicated at 400 W for 20 min, and centrifuged at 2000 rpm for 5 min. The supernatant was then evaporated in a 70 °C water bath until it was dry. Then, the sample was oven heated to a constant weight, and the total mass of fat in 1 g of liver was assessed by weighing.
2.6 Histological analysis
The livers were fixed in 4% paraformaldehyde, processed, and embedded in paraffin for haematoxylin-eosin (H&E) staining. The microscope slides were analysed using Image and Microsuite (Olympus Soft Imaging Solutions GmbH, Muenster, Germany).
2.7 Bacterial genomic DNA preparation and 16S rRNA sequencing
Total bacterial genomic DNA was extracted using a Fast DNA SPIN Extraction Kit (MP Biomedicals, CA, USA), and the quality of the DNA was assessed using a NanoDrop ND-1000 spectrophotometer (Thermo Fisher Scientific, Waltham, MA, USA) and agarose gel electrophoresis. The V3–V4 hypervariable regions of the 16S rRNA genes were amplified with polymerase chain reaction (PCR) using a forward primer (5′-ACTCCTACGGGAGGCAGCA-3′) and a reverse primer (5′-GGACTACHVGGGTWTCTAAT-3′). Gel electrophoresis and an AxyPrep DNA Gel Extraction Kit (AP-GX-500; Axygen) were used for the purification of the PCR products. The DNA library was constructed using a TruSeq Nano DNA LT Library Prep Kit (FC-121-4001; Illumina, San Diego, USA). A Quant-iTPicoGreen dsDNA Assay Kit was used to quantify the library on a Promega QuantiFluor System. The optimized library was tested using an Agilent High Sensitivity DNA Kit (5067-4626; Agilent, California, USA) and subsequently sequenced on the Illumina MiSeq Platform.
2.8 Bioinformatics analysis
Sequence data were analysed using quantitative insights into microbial ecology (QIIME) and R software packages (v3.2.0). Sequences with a similarity greater than 97% were defined as an operational taxonomic unit (OTU), and the most abundant sequence within each OTU was taken as the representative sequence. Each representative sequence was compared with the template sequence in the Silva database, and then the taxonomic information for each OTU was obtained. The sampling depth was analysed using the rarefaction curve generated by OTU clustering, and alpha diversity indexes, including the Chao 1, ACE, Shannon and Simpson indexes, were calculated to compare the richness and diversity among the samples. Beta diversity analysis was performed to identify structural variations in the microbial communities of the various samples using partial least squares discriminant analysis (PLS-DA) with the R software package (v2.15.3).
2.9 UPLC-Q-TOF-MSE analysis of faecal metabolites
2.9.1 Faecal sample preparation.
A total of 0.1 g of each faecal sample was mixed with 600 μl of methanol. The mixture was vortexed, mixed, left to sit at 4 °C for 1 h (the sample was vortexed for 15 min each time), and centrifuged (12
000 rpm, 25 min). Then, the supernatant was transferred to an autosampler vial for analysis.
2.9.2 Metabolomics discovery.
From each supernatant sample, one microliter aliquot was injected into a UPLC-ESI-QTOFMS system (ACQUITY UPLC I-Class, Waters) with a C18 column (100 × 2.1 mm, 1.7 μm, Waters ACQUITY UPLC BEH C18). The gradient mobile phase consisted of 0.1% formic acid in water (A) and 0.1% formic acid in acetonitrile (B). The gradient elution procedure was as follows: B: 5–20%, 1 min; 20–40%, 1.5 min; 40–100%, 6.5 min; 100%, 3 min; 100–5%, 0.5 min; and 5%, 2 min. The flow rate was 0.4 ml min−1. The column temperature was maintained at 45 °C. A Vion IMS QTOF mass spectrometer (Waters) was operated in both positive and negative modes and scanned at 50–1000 amu at a rate of 0.2 s per scan; the capillary voltages were 2 kV (positive mode) and 1 kV (negative mode). Other instrument conditions were as follows: source temperature: 115 °C, sampling cove: 40 V, and desolvation gas flow: 900 L h−1 at 450 °C.
2.10 Measurement of faecal short-chain fatty acids (SCFAs)
Briefly, 0.4 g of fresh faecal sample from each rat was placed in a weighing bottle to determine the water content. Another 0.4 g of the faecal sample was added to 1.6 mL of ultra-pure water and vortexed until it was mixed evenly. The sample was then centrifuged at 5000 rpm for 20 min, 0.8 mL of the supernatant was pipetted, and 0.2 mL of 50% H2SO4 and 1 mL of an ether solution containing the internal standard 2-methylpentanoic acid (50 μg mL−1) were added. The mixture was vortexed until it was evenly distributed. Then, the mixture was centrifuged at 12
000 rpm for 10 min and placed in a refrigerator (4 °C) for 30 min. After stratification, the upper diethyl ether phase was analysed using gas chromatography (GC-2010, SHIMADZU, Japan) (DB-FFAP capillary column, 30 m × 0.25 mm × 0.25 μm, Agilent Technologies) under the following conditions: the initial oven temperature (100 °C) was maintained for 1 min; then, the temperature was raised to 170 °C at 5 °C min−1 and then to 230 °C at 30 °C min−1, where it was maintained for 2 min. The temperatures of the injection port and flame ionization detector (FID) were 220 °C and 230 °C, respectively. The split ratio was 5
:
1. The internal standard curve method was used for quantification. Short-chain fatty acid standard references (SCFAs, >99.5%) were obtained from Aladdin Biochemical Technology Co., Ltd, Shanghai.
2.11 RNA preparation and quantitative RT-PCR analysis
Total RNA of colon tissues (50 mg) from each rat was extracted using a phenol-based method according to the manufacturer's instructions (TRIzol® Reagent, Takara, Dalian, China). cDNA was synthesized promptly using SuperScript III reverse transcriptase (Invitrogen, USA) after total RNA extraction. The mRNA expression levels of colonic occludin and claudin-1 were detected using an SYBR Premix Ex Taq™ QPCR kit (RR420A, Takara). Target gene expression (2−ΔΔCt) was normalized to endogenous beta-actin expression. The primers used are listed in the ESI (Table S1†).
2.12 Data processing and statistical analysis
Chromatographic and MS data were deconvoluted using Progenesis QI v2.3 (Waters). A multivariate data matrix containing information on sample identity, ion identity (retention time and m/z), and ion abundance was generated through centroiding, deisotoping, filtering, peak recognition, and integrating. UNIFI software and an online database [HMDB (http://www.hmdb.ca/) and lipidmaps (http://www.lipidmaps.org/tools/)] were used to analyse and identify the different metabolites in each group. Partial least squares discriminant analysis (PLS-DA) was performed using SIMCA-P software (Umetrics, Kinnelon, NJ). The differential metabolites were searched for based on the variable weight values (VIP > 1), the smallest coefficient of variation (min CV% < 30%), and the P-value from the Student-t test (p < 0.05).
All parameters were expressed as the mean ± SD. The results were statistically analysed by one-way ANOVA followed by a least significant difference (LSD) multiple comparison test. The criterion for significance was p < 0.05. Spearman correlation analyses of the abundance of bacterial genera, faecal differential metabolites and hepatic steatosis degree (described as the liver lipid content) were carried out (SPSS 19.0 software package, USA).
3 Results
3.1 Effects of PSEs on organ indexes of NAFLD rats
The main manifestation of the metabolic syndrome is the accumulation of fat in the liver and abdominal cavity. As shown in Fig. 1, the HFD alone significantly increased the liver index (P < 0.01) and abdominal fat index (P < 0.05) of the rats in the HFD group, whereas treatment with the two doses of PSEs (0.05 g per 100 g and 0.1 g per 100 g) significantly inhibited the increase in these two indexes (P < 0.05 and P < 0.01, respectively), even though the food intake was not significantly different between the rats in the PSE treatment groups and those in the HFD group (data not shown here).
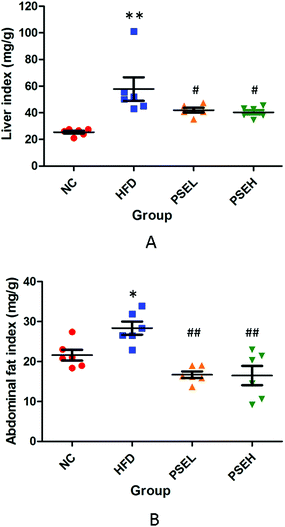 |
| Fig. 1 Effects of PSEs on the liver and abdominal fat indexes of rats in each group. A. Liver index; and B. abdominal fat index. NC: normal chow diet control group; HFD: high-fat diet group; PSEL: low-dose PSE treatment group (0.05 g per 100 g BW); and PSEH: high-dose PSE treatment group (0.10 g per 100 g BW). *P < 0.05, **P < 0.01 vs. NC, #P < 0.05, ##P < 0.01 vs. HFD. Data are presented as the average values ± SD of the samples in each group. | |
3.2 Effects of PSEs on the hepatic histology and hepatic lipid content of NAFLD rats
To further verify the degree of hepatic steatosis, histological examinations were carried out. Fig. 2A–D show representative hepatic pathological images of each group following H&E staining. The hepatic histology of the HFD group rats exhibited significant hepatocyte swelling and degeneration, balloon-like changes and fatty changes. In comparison with the HFD group, the PSE groups showed substantial improvements. A hepatic lipid content assay was performed to verify the above pathological changes, and the results are shown in Fig. 2E. After 12 weeks of HFD consumption, the hepatic lipid levels in the two PSE groups were significantly lower than those in the HFD group (P < 0.01, P < 0.05).
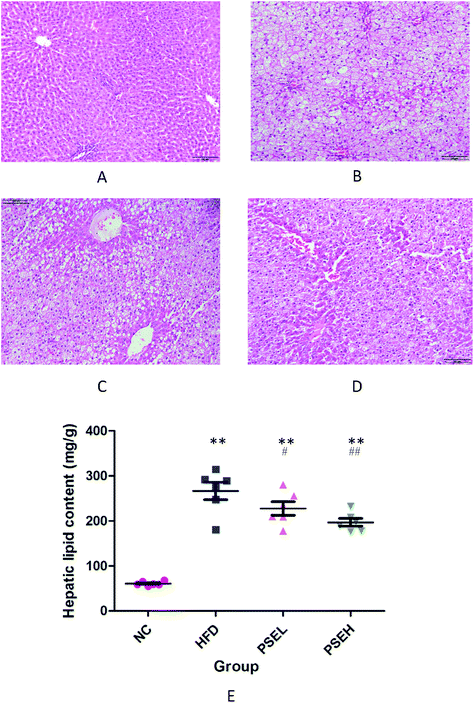 |
| Fig. 2 Representative images and the hepatic lipid content of the rats in the different groups. A. NC group. B. HFD group. C. PSEL group. D. PSEH group. E. Hepatic lipid content in each group. NC: normal chow diet control group; HFD: high-fat diet group; PSEL: low-dose PSE treatment group (0.05 g per 100 g BW); PSEH: high-dose PSE treatment group (0.10 g per 100 g BW). *P < 0.05, **P < 0.01 vs. NC, #P < 0.05, ##P < 0.01 vs. HFD. Data are presented as the average values ± SD of the samples in each group. | |
3.3 Effects of PSEs on the intestinal microbial community profile
The effects of PSEs on the diversity and composition of the gut microbiota were investigated using high-throughput sequencing. The results of the sequencing and calculated microbial community richness and alpha diversity indexes are shown in Fig. 3A, B and Table 1. From the richness and alpha diversity analysis, it was found that the Chao1 and ACE indexes of the HFD, PSEL and PSEH groups were significantly higher than those of the NC group (P < 0.01, P < 0.05), but the Shannon and Simpson indexes of the PSEH groups were similar to those of the NC group, and significantly higher than those of the HFD group (P < 0.05). Our findings indicate that HFD consumption led to an increase in richness and a decrease in the diversity of the gut microbiota, while a high dose of PSEs could partially correct the disturbance induced by the HFD.
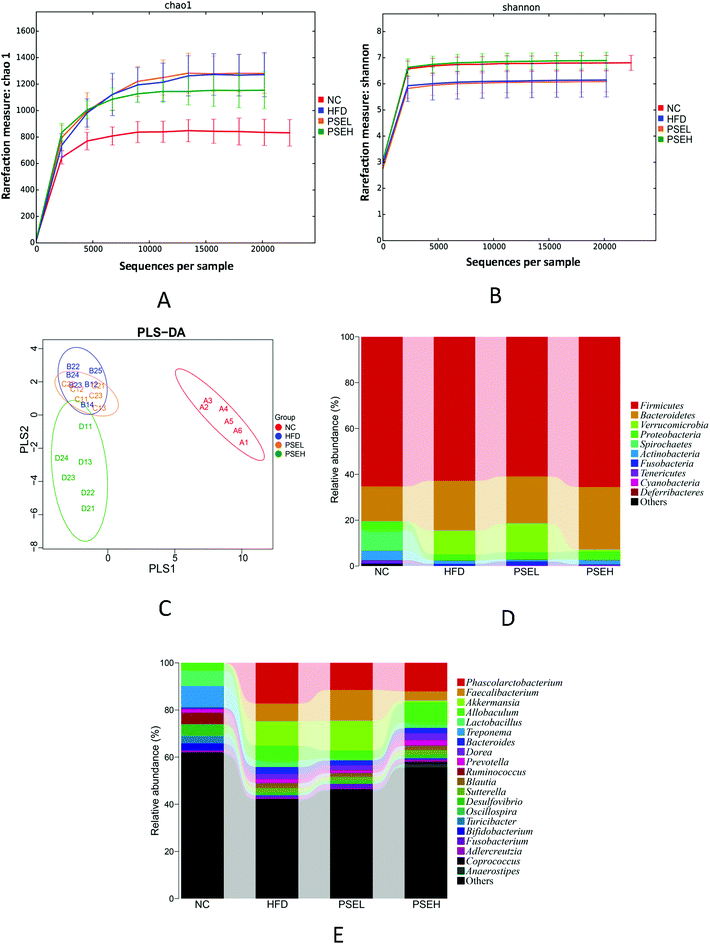 |
| Fig. 3 Effects of PSEs on intestinal flora diversity and the relative abundance of major microbial phyla and genera in NAFLD rats. A. Chao1 curves of different groups. B. Shannon curves of different groups. The Chao1 and Shannon curves both reached a plateau, indicating that the sequencing results are sufficient to reflect the abundance and diversity of the current sample. C. PLS-DA of the most important differences between the samples. D. and E. Relative abundance of major microbial phyla and genera in the different groups of rats. NC: normal chow diet control group; HFD: high-fat diet group; PSEL: low-dose PSE treatment group (0.05 g per 100 g BW); and PSEH: high-dose PSE treatment group (0.10 g per 100 g BW). Data are presented as the average values of the samples in each group. | |
Table 1 The diversity indexes of different groups
|
Chao1 |
ACE |
Simpson |
Shannon |
**P < 0.01 vs. NC, *P < 0.05 vs. NC, #P < 0.05 vs. HFD, ##P < 0.01 vs. HFD. |
NC |
832.04 ± 115.78 |
838.44 ± 120.13 |
0.97 ± 0.01 |
6.80 ± 0.33 |
HFD |
1105.63 ± 189.12** |
1179.67 ± 193.72** |
0.80 ± 0.04* |
5.28 ± 0.82* |
PSEL |
1274.26 ± 165.12** |
1365.67 ± 177.88** |
0.92 ± 0.03 |
6.09 ± 0.44 |
PSEH |
1157.15 ± 162.73* |
1175.15 ± 172.70* |
0.97 ± 0.01# |
6.89 ± 0.35# |
The differences in the gut microbiota components of the rats in each group were identified by PLS-DA (Fig. 3C). The data are presented as a 2D plot to illustrate the relationship. The gut microbial composition of the rats in the HFD group changed significantly since most of the dots in the HFD group were distinct from those of the NC group. The low-dose PSE group was similar to the HFD group. However, the high-dose PSE treatment notably changed the components of the microbial community, which were well separated from those of the HFD group. These results indicate that a high dose of PSEs can form distinct bacterial communities from those formed by a HFD or a normal diet.
Specifically, at the phylum level, the relative abundances of Firmicutes and Proteobacteria were 65.53 ± 6.46% and 3.47 ± 2.06% in the PSEH group, which were similar to those in the NC groups (65.30 ± 8.92% and 3.68 ± 3.55%, respectively). These values were slightly higher than those of the HFD and PSEL groups (62.83 ± 8.31% and 2.56 ± 1.97%; 60.92 ± 7.98% and 3.08 ± 1.69%, respectively). The relative abundance of Actinobacteria in the NC group was 4.25 ± 4.28%, which was significantly higher than those in the HFD group (1.55 ± 0.95%) and PSEL group (0.93 ± 0.31%) (P < 0.05). In addition, the relative abundances of Verrucomicrobia in the NC and PSEH groups were 0.00 ± 0.00% and 0.73 ± 1.33%, respectively, which were lower than those of the NC and PSEL groups (10.38 ± 15.03% and 12.58 ± 15.61%, respectively), but no significant difference was observed because of the high individual difference. The relative abundances of the above four phyla exhibited similarities between the NC and PSEH groups but differences between the HFD and PSEL groups. However, the high-dose PSE intervention significantly increased the level of Bacteroidetes (30.75 ± 6.26%) compared with the control condition (17.20 ± 4.88%) (P < 0.05) and was slightly higher than that induced by the HFD (20.44 ± 6.45%) and PSEL treatment (20.52 ± 8.72%) (Fig. 3D).
At the genus level, the relative abundances of Phascolarctobacterium in the NC, PSEL and PSEH groups were 0.02 ± 0.00%, 11.49 ± 8.89% and 12.08 ± 7.66%, respectively, which were lower than that of the HFD group (17.31 ± 3.89%); the relative abundances of Faecalibacterium in the NC and PSEH groups were 0.00 ± 0.00% and 3.95 ± 3.20%, respectively, which were significantly lower than those of the PSEL (13.09 ± 5.85%) and HFD (7.49 ± 4.37%) groups (P < 0.05); and the relative abundances of Akkermansia in the NC and PSEH groups were 0.00 ± 0.00% and 0.75 ± 1.33%, respectively, which were slightly lower than those of the HFD (10.39 ± 15.03%) and PSEL (12.58 ± 15.62%) groups. The total relative abundances of Adlercreutzia in the NC and PSEH groups were 0.87 ± 0.50% and 0.91 ± 0.71%, respectively, and its abundance in the PSEH group was significantly higher than those in the HFD (0.42 ± 0.29%) and PSEL (0.39 ± 0.17%) groups (P < 0.05). Additionally, we found that a high dose of PSE could significantly increase the relative abundance of Anaerostipes compared with the control condition (P < 0.05), HFD (P < 0.01) and PSEL treatment (P < 0.01).
The relative abundances of Treponema, Blautia, Fusobacterium, Bifidobacterium, Prevotella, Clostridiu, SMB 53, and Bilophila in the PSEH group exhibited trends more similar to those in the NC group than to those in the HFD and PSEL groups (Fig. 3E), but no significant differences were observed.
3.4 Effect of PSEs on faecal metabolites
Considering the changes in intestinal flora, the faecal metabolites were analysed by UPLC-Q-TOF/MS, and PCA was employed to determine the metabolic distinction among the four groups. Score plots of the PCA model are shown in Fig. 4A and B. The NC, HFD and PSEH groups were clearly classified in both the ESI+ and ESI− modes. The metabolic profile of rats in the high-dose PSE group differed greatly from that of the rats in the HFD group, suggesting that the deviations induced by a HFD were significantly regulated after the high-dose PSE treatment. These results were in accordance with the results of the intestinal flora profile to a certain degree.
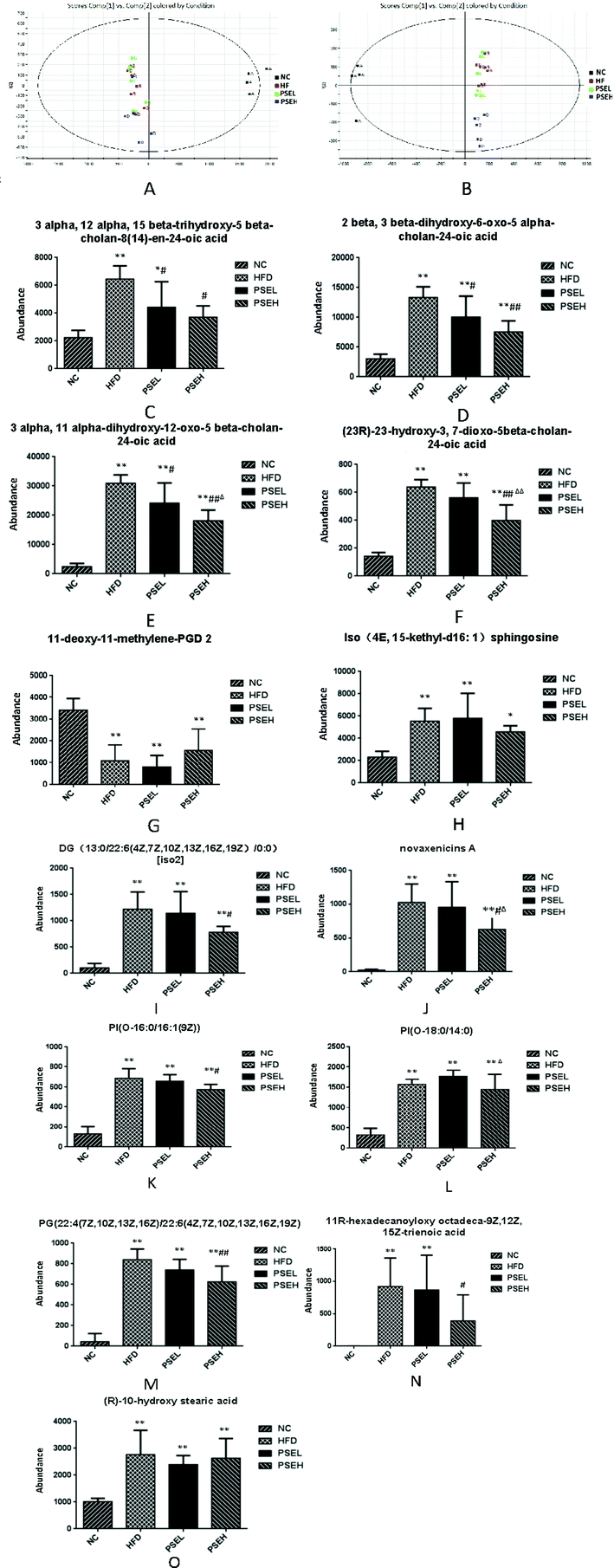 |
| Fig. 4 Effects of PSEs on faecal metabolites in NAFLD rats. (A and B) Score plots of the PCA model. (C—O) Comparison of the ionic strengths of the identified differential faecal metabolites. NC: normal chow diet control group; HFD: high-fat diet group; PSEL: low-dose PSE treatment group (0.05 g per 100 g BW); and PSEH: high-dose PSE treatment group (0.10 g per 100 g BW). *P < 0.05, **P < 0.01 vs. NC, #P < 0.05, ##P < 0.01 vs. HFD, ΔP < 0.05, ΔΔP < 0.01 vs. PSEL. Data are presented as the average values ± SD of the samples in each group. | |
Among the ions, 13 differential metabolites were identified by searching the human metabolome database (HMDB) using accurate mass with variable importance in projection (VIP) values greater than 1, P-values from Student's t test below 0.05 and min CV% < 30%. These differential metabolites can be divided into bile acid metabolic products [3-alpha,12-alpha,15-beta-trihydroxy-5-beta-cholan-8(14)-en-24-oic acid; 2-beta,3-beta-dihydroxy-6-oxo-5-alpha-cholan-24-oic acid; 3-alpha,11-alpha-dihydroxy-12-oxo-5beta-cholan-24-oic acid; and (23R)-23-hydroxy-3,7-dioxo-5beta-cholan-24-oic acid], lipids [11-deoxy-11-methylene-PGD 2, iso (4E, 15-methyl-d16:1) sphingosine; diglycerides DG(13:0/22:6(4Z,7Z,10Z,13Z,16Z,19Z)/0:0)], phospholipids [PI(O-16:0/16:1(9Z)), PI(O-18:0/14:0), PG(22:4(7Z,10Z,13Z,16Z)/22:6(4Z,7Z,10Z,13Z,16Z,19Z))], fatty acids [11R-hexadecanoyloxy octadeca-9Z,12Z,15Z-trienoic acid and (R)-10-hydroxy stearic acid] and novaxenicins A. Except for 11-deoxy-11-methylene-PGD2, 12 differential metabolites were positively correlated with the degree of hepatic steatosis (quantitative description with the hepatic lipid content) (Table 2). Fig. 4 (C–O) shows comparisons of the ionic strengths of the differential metabolites in each group of rats. The ionic strengths of the 12 differential metabolites in the HFD group were significantly higher than those in the NC group (except 11-deoxy-11-methylene-PGD 2) (P < 0.05). Compared with the HFD group, the high-dose PSE intervention significantly decreased the four bile acid metabolism products, DGs, novaxenicins A, PI(O-16:0/16:1(9Z)), PG(22:4(7Z,10Z,13Z,16Z)/22:6(4Z,7Z,10Z,13Z,16Z,19Z)) and 11R-hexadecanoyloxy octadeca-9Z,12Z,15Z-trienoic acid (P < 0.05).
Table 2 Effect of PSE interventions on differential metabolites in faeces of NAFLD rats
Differential metabolites |
UPLC-MS identification information |
Change trends |
Correlation analysis vs. liver lipid content |
Compound ID |
Observed m/z |
Observed RT (min) |
Adducts |
NC/HFD |
PSEL/HFD |
PSEH/HFD |
Correlation coefficient |
P-Value |
For Spearman correlation analysis results, the smaller the P-value, the more accurate the correlation analysis result. |
3alpha,12alpha,15beta-trihydroxy-5beta-cholan-8(14)-en-24-oic acid |
LMST 04010358 |
407.2797 |
3.51 |
+H |
↓ |
↓ |
↓ |
0.628 |
0.0002 |
2beta,3beta-dihydroxy-6-oxo-5alpha-cholan-24-oic acid |
LMST 04010172 |
405.2645 |
3.77 |
−H |
↓ |
↓ |
↓ |
0.746 |
0.0000 |
3alpha,11alpha-dihydroxy-12-oxo-5beta-cholan-24-oic acid |
LMST 04010337 |
405.2643 |
4.04 |
−H |
↓ |
↓ |
↓ |
0.832 |
0.0000 |
(23R)-23-Hydroxy-3,7-dioxo-5beta-cholan-24-oic acid |
LMST 04010346 |
403.2486 |
4.2 |
−H |
↓ |
↓ |
↓ |
0.817 |
0.0000 |
11-Deoxy-11-methylene-PGD2 |
LMFA 03010103 |
351.2505 |
5.95 |
+H |
↑ |
↓ |
↑ |
−0.620 |
0.0003 |
Iso (4E,15-methyl-d16:1) sphingosine |
LMSP 01080005 |
286.2752 |
5.55 |
+H, M − H2O + H |
↓ |
↑ |
↓ |
0.510 |
0.0040 |
DG(13:0/22:6(4Z,7Z,10Z,13Z,16Z,19Z)/0:0)[iso2] |
LMGL 02010377 |
597.4495 |
9.85 |
−H |
↓ |
↑ |
↓ |
0.670 |
0.0001 |
Novaxenicins A |
LMPR 0104420001 |
347.1862 |
7.13 |
−H |
↑ |
↑ |
↓ |
0.704 |
0.0000 |
PI(O-16:0/16:1(9Z)) |
LMGP 06020008 |
793.5212 |
4.33 |
−H |
↓ |
↓ |
↓ |
0.833 |
0.0000 |
PI(O-18:0/14:0) |
LMGP 06020021 |
795.5372 |
7.43 |
−H |
↓ |
↑ |
↓ |
0.779 |
0.0000 |
PG(22:4(7Z,10Z,13Z,16Z)/22:6(4Z,7Z,10Z,13Z,16Z,19Z)) |
LMGP 04010978 |
869.5333 |
3.38 |
−H |
↓ |
↓ |
↓ |
0.904 |
0.0000 |
11R-Hexadecanoyloxy octadeca-9Z,12Z,15Z-trienoic acid |
LMFA 07010015 |
531.4407 |
10.37 |
−H |
↓ |
↑ |
↓ |
0.489 |
0.0061 |
(R)-10-Hydroxy stearic acid |
LMFA 02000237 |
299.2593 |
8.93 |
−H |
↓ |
↓ |
↓ |
0.417 |
0.0220 |
3.5 Spearman correlation analysis between faecal differential metabolites and the components of the intestinal flora at the genus level
Considering the differences in the above 13 metabolites among the four groups, the correlations between the ionic strengths of these compounds and the components of the intestinal flora at the genus level were analysed using Spearman correlation analysis. The result was exhibited with a clustered heatmap that illustrated the relative increasing (red) or decreasing (blue) trend, as shown in Fig. 5. Phascolarctobacterium, Faecalibacterium, and Akkermansia, which were lower in the PSEH and NC groups than in the HFD group, were positively correlated with 12 differential faecal metabolites. Adlercreutzia and Anaerostipes, which were higher in the PSEH and NC groups than in the HFD group, were negatively correlated with 12 differential metabolites (except for 11-deoxy-11-methylene-PGD2, which exhibited the opposite trend).
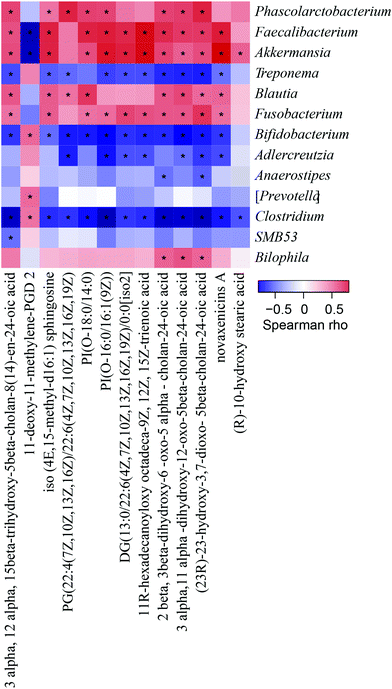 |
| Fig. 5 Results of the Spearman correlation analysis between 13 differential faecal metabolites and corresponding gut microflora components at the genus level. The heatmap diagram illustrates the correlation analysis conducted among 13 differential faecal metabolites and the bacterial genera. If the correlation between two components is positive, it is displayed in red and if it is negative, it is displayed in blue. The heatmap is filtered and displayed with a significance level of P < 0.05, and significance is noted as “*” in the square. | |
3.6 The effects of PSEs on faecal SCFA concentration
Except the metabolites mentioned above, the present study investigated the faecal SCFA levels of the rats in each group. As shown in Fig. 6A–E, a HFD alone led to an obvious decrease in SCFAs in the present study, but the treatment with PSEs could partially inhibit this decrease. Specifically, the concentrations of acetic acid (P < 0.01), butyric acid (P < 0.01), and isobutyric acid (P < 0.05) were significantly lower in the HFD group than in the NC group. The isobutyric acid (P < 0.05), valeric acid (P < 0.01) and isovaleric acid (P < 0.05) concentrations were significantly higher (P < 0.05) in the PSEH group than in the HFD group.
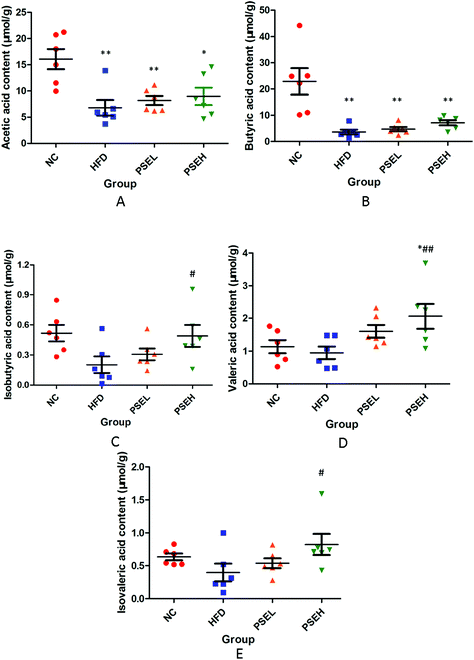 |
| Fig. 6 Effects of PSEs on faecal SCFAs in NAFLD rats. A. Acetic acid; B. butyric acid; C. isobutyric acid; D. valeric acid; and E. isovaleric acid. NC: normal chow diet control group; HFD: high-fat diet group; PSEL: low-dose PSE treatment group (0.05 g per 100 g BW); and PSEH: high-dose PSE treatment group (0.10 g per 100 g BW). *P < 0.05, **P < 0.01 vs. NC, #P < 0.05, ##P < 0.01 vs. HFD, ΔP < 0.05, ΔΔP < 0.01 vs. PSEL. Data are presented as the average values ± SD of the samples in each group. | |
3.7 The effects of PSEs on the mRNA expression of tight junction proteins
Tight junctions (TJs) play an important role in maintaining intestinal barrier integrity, and are composed of a variety of tight junction proteins (transmembrane proteins), such as ZO-1, Occludin and Claudin. Studies have demonstrated that the gut microbiota imbalance and small intestinal mucosal permeability increase in NAFLD.10 Hence, the mRNA expression levels of colonic tight junction proteins were quantified in our study. The results showed that the mRNA expression levels of colonic Occludin exhibited decreasing trends in the HFD group, while the mRNA expression levels of colonic Claudin-1 and Occludin in the high-dose PSE group were significantly higher than those in the HFD group (Fig. 7) (P < 0.05). This result suggested that treatment with PSEs may improve the intestinal mechanical barrier.
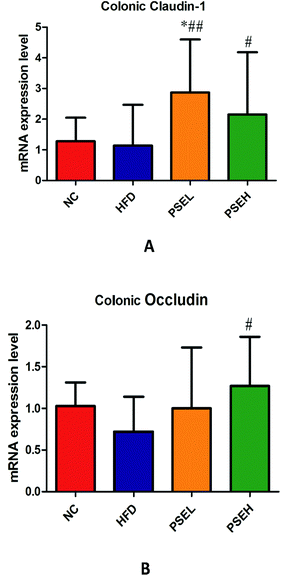 |
| Fig. 7 The effects of PSEs on the mRNA expression levels of Claudin-1 and Occludin in colon tissues. A. Claudin-1; and B. Occludin. NC: normal chow diet control group; HFD: high-fat diet group; PSEL: low-dose PSE treatment group (0.05 g per 100 g BW); and PSEH: high-dose PSE treatment group (0.10 g per 100 g BW). *P < 0.05, **P < 0.01 vs. NC, #P < 0.05, ##P < 0.01 vs. HFD, ΔP < 0.05, ΔΔP < 0.01 vs. PSEL. | |
4 Discussion
The components of the intestinal flora in healthy people are formed in a certain ratio. The bacteria are mutually restricted and interdependent and establish a balance in their quantity and variety. Once this balance is broken, the occurrence and progression of certain diseases can be exacerbated due to the effects of the intestinal flora metabolites and intestinal permeability.11 Increasing evidence has shown that gut microbiota alteration and barrier function defects are closely related to the “second strike” of the liver and are important pathological factors leading to the development of NAFLD through the “intestinal-hepatic axis”.11,12 Therefore, the regulation of intestinal flora dysbiosis through a diet that includes food containing functional phytochemicals may provide a practical strategy for preventing or treating nonalcoholic liver disease.
In our previous study, we found that PSEs could alleviate hepatic steatosis in NAFLD rats, in addition to their regulatory role in cholesterol metabolism.8 In this study, the effect of PSEs on intestinal flora and faecal metabolites of NAFLD rats was further explored.
The results showed that a HFD alone increased the richness of intestinal flora but that the diversity was lower compared with that in the normal control group. This finding further proved that the HFD resulted in dysbiosis of the gut microbiota, whereas a high-dose PSE treatment could increase both the richness and diversity of intestinal flora and form bacterial communities distinct from those induced by a HFD or normal diet.
Specifically, at the phylum level, the relative abundances of Firmicutes, Proteobacteria and Actinobacteria in the PSEH group were close to those in the NC group. However, the relative abundance of Bacteroidetes in the PSEH group was significantly higher than those in the NC, HFD and PSEL groups. Kyung-Ah Kim et al.12 found that HFD consumption led to an increase in Firmicutes and a reduction in Bacteriodetes and Proteobacteria abundances, which is partially consistent with our results. Firmicutes and Bacteroidetes are the dominant bacteria that occupy approximately 95% of human intestinal flora.13 Studies have reported a higher proportion of Firmicutes and a lower amount of Bacteroidetes in obese NAFLD patients.14,15 Another study showed that the frequency of increased Firmicutes species coincides with decreased Bacteroidetes in the guts of obese mice by increasing the host's capacity for energy harvesting from foods.16 Our present experimental results implied that supplementation with PSEs (0.1 g per 100 g BW) may prevent hepatic steatosis induced by a HFD partially by increasing the amount of Bacteroidetes.
At the genus level, a HFD significantly increased the relative abundances of Phascolarctobacterium, Faecalibacterium and Akkermansia and decreased the relative abundances of Adlercreutzia and Anaerostipes. However, a high dose of PSEs could partially reverse these changes, resulting in relative abundances that are closer to those observed in the NC condition. Importantly, a high dose of a PSE could significantly increase the relative abundances of Adlercreutzia and Anaerostipes. Adlercreutzia are short-chain fatty acid-producing bacteria and play a beneficial role in immune regulation and anti-inflammation.17 Studies have shown that Anaerostipes are butyrate-producing colon bacteria that produce butyric acid via the fermentation of acetic acid and lactic acid.18 Therefore, the increasing abundances of Adlercreutzia and Anaerostipes induced by PSEs probably contribute to the improvement of the intestinal microenvironment (the increase in isobutyric acid, valeric acid and isovaleric acid in this study) and the inflammatory state.
Moreover, Akkermansia is a representative bacterium of Verrucomicrobia found in the intestine. A study showed that Akkermansia is associated with a range of metabolic diseases, including obesity and type 2 diabetes.19 Oral administration of Akkermansia can improve alcohol-induced liver disease.20 However, the effect of Akkermansia on the body is perhaps not always positive. Other studies have found that colonization of Akkermansia in sterile mice can aggravate Salmonella-induced intestinal inflammation by interfering with the mucus balance of the host.21 In addition, high-dose intake of haem iron significantly increased the abundance of A. muciniphila in the intestines of mice while destroying the intestinal mucus layer.22
Faecalibacterium is one of the most common anaerobic bacteria in the human gut. Studies have found that it is associated with chronic metabolic diseases such as diabetes, obesity and host digestive diseases (e.g. inflammatory bowel), which suggests that this intestinal bacteria may affect multiple metabolic pathways in the host.23,24
The intestine is a complex system in which the growth of bacteria is related to the interaction between bacteria. Therefore, the metabolic characteristics of Akkermansia and Faecalibacterium and their physiological role related to high-dose PSE preventive effects on NAFLD in the present study still require further investigation.
Changes in the intestinal flora will inevitably lead to changes in faecal metabolites with many different bioactive properties (e.g., regulation of inflammatory and metabolic responses). Therefore, this study also examined the effects of PSEs on faecal metabolites and SCFAs. Thirteen differential metabolites were identified, including bile acid metabolism products and lipids (DGs, phosphatidylglycerol, and phosphatidylinositol). The high-dose PSE group showed significantly decreased ionic strengths of bile acid metabolism-related products compared with the HFD group. Studies have shown that bile acids play a critical role in maintaining lipid and glucose metabolism and insulin sensitivity through the farnesoid X receptor (FXR) and the G protein-coupled receptor TGR5.25,26 Zampa et al.27 found that the faecal bile acid content significantly decreased after volunteers were supplemented with Bifidobacterium and Lactobacillus, which is similar to our findings. In addition, some researchers have confirmed that probiotics can reduce the conversion of primary bile acids into secondary bile acids in the intestine, which may inhibit the development of malignant tumours.28 The above evidence implied that PSEs may serve as “prebiotics” that promote the growth of probiotics (e.g., Adlercreutzia and Anaerostipes, SCFA-producing bacteria), and may contribute to the decrease in bile acid metabolism products.
In addition, a high-dose PSE intervention could significantly decrease the ionic strengths of DGs, phosphatidylinositol, and phosphatidylglycerol compared with those induced by a HFD. DGs are precursors of triglycerides and can aggravate NAFLD in two ways: they directly activate protein kinase C (PKC), and PKC can reduce the tyrosine phosphorylation of insulin receptor substrates, resulting in additional obstacles in related signalling pathways and the promotion of insulin resistance (IR);29 DGs can also directly damage liver cells, leading to excessive reactive oxygen species (ROS) production and aggravation of lipid peroxidation and oxidative stress, which can aggravate liver damage.30 The above results provide another clue for the preventive effect of high-dose PSE treatment on the progression of NAFLD pathology.
To investigate the causes underlying the production of these metabolites, we further analysed the correlation of the above differential metabolites with the high relative abundance of bacteria at the genus level. Notably, the high dose of PSEs significantly reduced the relative abundance of Faecalibacterium, which was positively correlated with the ionic strengths of the above metabolites (except 11-deoxy-11-methylene-PGD 2), and significantly increased the relative abundances of Adlercreutzia and Anaerostipes, which were negatively correlated with the ionic strengths of the above metabolites (except 11-deoxy-11-methylene-PGD 2). These components of the intestinal flora are likely some of the key regulatory targets for the ameliorative effect of PSEs on the degree of hepatic steatosis. However, the role of these bile acid metabolism products and related signalling pathways remains to be further investigated.
Moreover, among the variety of gut microbiota metabolites, SCFAs have received the most attention because of their function in maintaining the haemostasis of hosts and promoting recovery from diseases. Hence, we explored the changes in faecal SCFAs and found that the contents of isobutyric acid, valeric acid and isovaleric acid were significantly higher in the PSEH group than in the HFD group. This finding may be attributed to the promotion effects of PSEH on the relative abundances of Adlercreutzia and Anaerostipes, SCFA-producing bacteria. Previous studies have shown that SCFAs (mainly acetate, propionate, butyrate, iso-butyrate, valerate, iso-valerate, and hexanoate) have the biological functions of increasing insulin sensitivity and energy expenditure, reversing the production of ROS, inhibiting the production of proinflammatory cytokines and directly serving as important energy resources via their action on certain molecular targets, such as G protein-coupled receptor 43 (GPR43)/free fatty acid receptor 2 (FFAR2), GPR41/FFAR3, GPCR109A/hydroxycarboxylic acid receptor 2 (HCA2), GPCR81/HCA1, histone deacetylase 1 (HDAC1), and HDAC3.31–33 The present study showed that the induction of SCFA components by PSEs is another pathway related to the amelioration of the progression of NAFLD. Notably, however, different intervention strategies lead to increases in different types of SCFAs.34 Therefore, the roles of isobutyric acid, valeric acid and isovaleric acid in the prevention of PSEs on NAFLD still require further exploration.
The intestinal barrier includes mechanical barriers, immune barriers, microbial barriers, and chemical barriers. Among the four barriers, mechanical barriers are the most important. Tight junctions are the most important structures constituting the mechanical barrier of the intestinal mucosa. They are composed of claudin, occludin, ZOs and other structural proteins and various connexin molecules. There is a close relationship between the intestinal mucosal barrier and the intestinal microecological balance. A study showed that the intestinal mucosal permeability changed significantly in NAFLD patients who consumed a high-fat and high-sugar diet.35 Another in vitro study demonstrated that a HFD can increase intestinal permeability and reduce the expression of tight junction proteins in intestinal epithelial cells.36 Increased intestinal permeability and bacterial flora translocation lead to intestinal bacterial toxins entering the liver through the portal vein, which causes abnormal bile secretion, the expression of hepatic tight junction proteins and liver damage. Our results showed that a HFD alone led to a decreasing trend of colonic Occludin at the mRNA level. While high-dose PSE treatment significantly increased the mRNA expression levels of Colonic Claudin-1 and Occludin in the PSEH group compared with those in the HFD group. The upregulation of the mRNA expression of these two tight junction proteins may be another reason for its correction of bile acid metabolism disturbance and its third pathway, resulting in the prevention of NAFLD progression.
In summary, the PSE intervention doses that we used in the present study were equivalent to 3 g d−1 and 6 g d−1, respectively, in humans. A high dose of PSEs could regulate intestinal flora dysbiosis induced by a HFD by increasing the relative abundance and diversity. Accordingly, this increase influences some of the faecal metabolites related to bile acid and lipid metabolism; furthermore, a high dose of a PSE could increase the level of partial SCFA components and the mRNA expression levels of partial tight junction proteins (claudin and occludin). However, the definite mechanism of some bacteria and their related metabolites in the preventive effect of PSEs on NAFLD (including SCFAs) still requires further investigation.
Conflicts of interest
The authors declare no conflict of interest.
Acknowledgements
This work was supported by grants from the Newtrition™ Asia Research Grant by BASF (SA 1500053), the National Natural Science Foundation of China (Grant No. 81472770, 81672350, and 81872225), and the Shanghai Municipal Planning Commission of Science and Research Fund (201640256).
References
- G. Vernon, A. Baranova and Z. M. Younossi, Systematic review: the epidemiology and natural history of non-alcoholic fatty liver disease and non-alcoholic steatohepatitis in adults, Aliment. Pharmacol. Ther., 2011, 34, 274–285 CrossRef CAS.
- G. Bedogni, L. Miglioli, F. Masutti, C. Tiribelli, G. Marchesini and S. Bellentani, Prevalence of and risk factors for nonalcoholic fatty liver disease: the Dionysos nutrition and liver study, Hepatology, 2005, 42, 44–52 CrossRef.
- D. G. Tiniakos, M. B. Vos and E. M. Brunt, Nonalcoholic fatty liver disease: pathology and pathogenesis, Annu. Rev. Pathol., 2010, 5, 145–171 CrossRef CAS.
- Q. M. Anstee, A. K. Daly and C. P. Day, Genetics of alcoholic and nonalcoholic fatty liver disease, Semin. Liver Dis., 2011, 31, 128–146 CrossRef CAS PubMed.
- B. Schnabl and D. A. Brenner, Interactions between the intestinal microbiome and liver diseases, Gastroenterology, 2014, 146, 1513–1524 CrossRef CAS.
- M. L. Balmer, E. Slack, A. de Gottardi, M. A. Lawson, S. Hapfelmeier, L. Miele, A. Grieco, H. Van Vlierberghe, R. Fahrner, N. Patuto, C. Bernsmeier, F. Ronchi, M. Wyss, D. Stroka, N. Dickgreber, M. H. Heim, K. D. McCoy and A. J. Macpherson, The liver may act as a firewall mediating mutualism between the host and its gut commensal microbiota, Sci. Transl. Med., 2014, 6, 237ra266 Search PubMed.
- X. Luo, P. Su and W. Zhang, Advances in Microalgae-Derived Phytosterols for Functional Food and Pharmaceutical Applications, Mar. Drugs, 2015, 13, 4231–4254 CrossRef CAS.
- L. Song, D. Qu, Q. Zhang, J. Jiang, H. Zhou, R. Jiang, Y. Li, Y. Zhang and H. Yan, Phytosterol esters attenuate hepatic steatosis in rats with non-alcoholic fatty liver disease rats fed a high-fat diet, Sci. Rep., 2017, 7, 41604 CrossRef CAS.
- J. Folch, M. Lees and G. H. Sloane Stanley, A simple method for the isolation and purification of total lipids from animal tissues, J. Biol. Chem., 1957, 226, 497–509 CAS.
- L. Miele, V. Valenza, G. La Torre, M. Montalto, G. Cammarota, R. Ricci, R. Masciana, A. Forgione, M. L. Gabrieli, G. Perotti, F. M. Vecchio, G. Rapaccini, G. Gasbarrini, C. P. Day and A. Grieco, Increased intestinal permeability and tight junction alterations in nonalcoholic fatty liver disease, Hepatology, 2009, 49, 1877–1887 CrossRef CAS.
- A. Goel, M. Gupta and R. Aggarwal, Gut microbiota and liver disease, J. Gastroenterol. Hepatol., 2014, 29, 1139–1148 CrossRef.
- K. A. Kim, W. Gu, I. A. Lee, E. H. Joh and D. H. Kim, High fat diet-induced gut microbiota exacerbates inflammation and obesity in mice via the TLR4 signaling pathway, PLoS One, 2012, 7, e47713 CrossRef CAS.
- M. Li, B. Wang, M. Zhang, M. Rantalainen, S. Wang, H. Zhou, Y. Zhang, J. Shen, X. Pang, M. Zhang, H. Wei, Y. Chen, H. Lu, J. Zuo, M. Su, Y. Qiu, W. Jia, C. Xiao, L. M. Smith, S. Yang, E. Holmes, H. Tang, G. Zhao, J. K. Nicholson, L. Li and L. Zhao, Symbiotic gut microbes modulate human metabolic phenotypes, Proc. Natl. Acad. Sci. U. S. A., 2008, 105, 2117–2122 CrossRef CAS.
- L. Zhu, S. S. Baker, C. Gill, W. Liu, R. Alkhouri, R. D. Baker and S. R. Gill, Characterization of gut microbiomes in nonalcoholic steatohepatitis (NASH) patients: a connection between endogenous alcohol and NASH, Hepatology, 2013, 57, 601–609 CrossRef CAS.
- M. Doulberis, G. Kotronis, D. Gialamprinou, J. Kountouras and P. Katsinelos, Non-alcoholic fatty liver disease: An update with special focus on the role of gut microbiota, Metabolism, 2017, 71, 182–197 CrossRef CAS.
- P. J. Turnbaugh, R. E. Ley, M. A. Mahowald, V. Magrini, E. R. Mardis and J. I. Gordon, An obesity-associated gut microbiome with increased capacity for energy harvest, Nature, 2006, 444, 1027–1031 CrossRef.
- B. Lamas, M. L. Richard, V. Leducq, H. P. Pham, M. L. Michel, G. Da Costa, C. Bridonneau, S. Jegou, T. W. Hoffmann, J. M. Natividad, L. Brot, S. Taleb, A. Couturier-Maillard, I. Nion-Larmurier, F. Merabtene, P. Seksik, A. Bourrier, J. Cosnes, B. Ryffel, L. Beaugerie, J. M. Launay, P. Langella, R. J. Xavier and H. Sokol, CARD9 impacts colitis by altering gut microbiota metabolism of tryptophan into aryl hydrocarbon receptor ligands, Nat. Med., 2016, 22, 598–605 CrossRef CAS.
- A. Riviere, M. Selak, D. Lantin, F. Leroy and L. De Vuyst, Bifidobacteria and Butyrate-Producing Colon Bacteria: Importance and Strategies for Their Stimulation in the Human Gut, Front. Microbiol., 2016, 7, 979 Search PubMed.
- M. Yassour, M. Y. Lim, H. S. Yun, T. L. Tickle, J. Sung, Y. M. Song, K. Lee, E. A. Franzosa, X. C. Morgan, D. Gevers, E. S. Lander, R. J. Xavier, B. W. Birren, G. Ko and C. Huttenhower, Sub-clinical detection of gut microbial biomarkers of obesity and type 2 diabetes, Genome Med., 2016, 8, 17 CrossRef.
- C. Grander, T. E. Adolph, V. Wieser, P. Lowe, L. Wrzosek, B. Gyongyosi, D. V. Ward, F. Grabherr, R. R. Gerner, A. Pfister, B. Enrich, D. Ciocan, S. Macheiner, L. Mayr, M. Drach, P. Moser, A. R. Moschen, G. Perlemuter, G. Szabo, A. M. Cassard and H. Tilg, Recovery of ethanol-induced Akkermansia muciniphila depletion ameliorates alcoholic liver disease, Gut, 2018, 67, 891–901 CrossRef.
- B. P. Ganesh, R. Klopfleisch, G. Loh and M. Blaut, Commensal Akkermansia muciniphila exacerbates gut inflammation in Salmonella Typhimurium-infected gnotobiotic mice, PLoS One, 2013, 8, e74963 CrossRef CAS.
- J. E. van Hylckama Vlieg, P. Veiga, C. Zhang, M. Derrien and L. Zhao, Impact of microbial transformation of food on health - from fermented foods to fermentation in the gastro-intestinal tract, Curr. Opin. Biotechnol, 2011, 22, 211–219 CrossRef CAS.
- J. Feng, H. Tang, M. Li, X. Pang, L. Wang, M. Zhang, Y. Zhao, X. Zhang and J. Shen, The abundance of fecal Faecalibacterium prausnitzii in relation to obesity and gender in Chinese adults, Arch. Microbiol., 2014, 196, 73–77 CrossRef CAS.
- J. Gerritsen, H. Smidt, G. T. Rijkers and W. M. de Vos, Intestinal microbiota in human health and disease: the impact of probiotics, Genes Nutr., 2011, 6, 209–240 CrossRef.
- J. Y. Chiang, Bile acids: regulation of synthesis, J. Lipid Res., 2009, 50, 1955–1966 CrossRef CAS.
- C. Thomas, A. Gioiello, L. Noriega, A. Strehle, J. Oury, G. Rizzo, A. Macchiarulo, H. Yamamoto, C. Mataki, M. Pruzanski, R. Pellicciari, J. Auwerx and K. Schoonjans, TGR5-mediated bile acid sensing controls glucose homeostasis, Cell Metab., 2009, 10, 167–177 CrossRef CAS.
- A. Zampa, S. Silvi, R. Fabiani, G. Morozzi, C. Orpianesi and A. Cresci, Effects of different digestible carbohydrates on bile acid metabolism and SCFA production by human gut micro-flora grown in an in vitro semi-continuous culture, Anaerobe, 2004, 10, 19–26 CrossRef CAS.
- S. Sato, H. Nagai and Y. Igarashi, Effect of probiotics on serum bile acids in patients with ulcerative colitis, Hepatogastroenterology, 2012, 59, 1804–1808 Search PubMed.
- V. T. Samuel, Z. X. Liu, A. Wang, S. A. Beddow, J. G. Geisler, M. Kahn, X. M. Zhang, B. P. Monia, S. Bhanot and G. I. Shulman, Inhibition of protein kinase Cepsilon prevents hepatic insulin resistance in nonalcoholic fatty liver disease, J. Clin. Invest., 2007, 117, 739–745 CrossRef CAS.
- N. Kumashiro, D. M. Erion, D. Zhang, M. Kahn, S. A. Beddow, X. Chu, C. D. Still, G. S. Gerhard, X. Han, J. Dziura, K. F. Petersen, V. T. Samuel and G. I. Shulman, Cellular mechanism of insulin resistance in nonalcoholic fatty liver disease, Proc. Natl. Acad. Sci. U. S. A., 2011, 108, 16381–16385 CrossRef CAS.
- W. Huang, H. L. Guo, X. Deng, T. T. Zhu, J. F. Xiong, Y. H. Xu and Y. Xu, Short-Chain Fatty Acids Inhibit Oxidative Stress and Inflammation in Mesangial Cells Induced by High Glucose and Lipopolysaccharide, Exp. Clin. Endocrinol. Diabetes, 2017, 125, 98–105 CrossRef CAS.
- P. Vernocchi, F. Del Chierico and L. Putignani, Gut Microbiota Profiling: Metabolomics Based Approach to Unravel Compounds Affecting Human Health, Front. Microbiol., 2016, 7, 1144 Search PubMed.
- J. Tan, C. McKenzie, M. Potamitis, A. N. Thorburn, C. R. Mackay and L. Macia, The role of short-chain fatty acids in health and disease, Adv. Immunol., 2014, 121, 91–119 CAS.
- J. H. Tao, J. A. Duan, S. Jiang, J. M. Guo, Y. Y. Qian and D. W. Qian, Simultaneous determination of six short-chain fatty acids in colonic contents of colitis mice after oral administration of polysaccharides from Chrysanthemum morifolium Ramat by gas chromatography with flame ionization detector, J. Chromatogr. B: Anal. Technol. Biomed. Life Sci., 2016, 1029–1030, 88–94 CrossRef CAS.
- V. Volynets, M. A. Kuper, S. Strahl, I. B. Maier, A. Spruss, S. Wagnerberger, A. Konigsrainer, S. C. Bischoff and I. Bergheim, Nutrition, intestinal permeability, and blood ethanol levels are altered in patients with nonalcoholic fatty liver disease (NAFLD), Dig. Dis Sci., 2012, 57, 1932–1941 CrossRef CAS.
- P. D. Cani, R. Bibiloni, C. Knauf, A. Waget, A. M. Neyrinck, N. M. Delzenne and R. Burcelin, Changes in gut microbiota control metabolic endotoxemia-induced inflammation in high-fat diet-induced obesity and diabetes in mice, Diabetes, 2008, 57, 1470–1481 CrossRef CAS.
Footnotes |
† Electronic supplementary information (ESI) available. See DOI: 10.1039/c9fo01570a |
‡ Co-first author. |
|
This journal is © The Royal Society of Chemistry 2020 |