Microplastics removal in wastewater treatment plants: a critical review†
Received
27th April 2020
, Accepted 5th August 2020
First published on 26th August 2020
Abstract
Wastewater treatment plants (WWTPs) are an important route for microplastics to enter aquatic environments. Microplastics have been recently identified in sewage samples in Russia, Sweden, France, Finland, USA, UK, Netherlands, Germany, Canada, Australia, Italy, Turkey, Denmark, Poland, China and South Korea. The aim of this study was to examine and quantify the removal efficacy of microplastics by WWTPs. Experimental methods employed in sampling, analysis and quantification of microplastics vary widely between studies. Microplastic removal rates in 21 studies were compared. Secondary and tertiary WWTPs removed an average of 88% and 94% of microplastics, respectively. The majority of microplastics, 72% on average, were removed during preliminary and primary treatment. Calculations of the settling/floating velocities of commonly used polymers indicate that primary sedimentation removes spherical particles >27–149 μm in diameter, depending on the polymer in question. Thus, the majority of microplastics removed during wastewater treatment are likely to be present in sewage sludge. Although the removal of microplastics is high, WWTPs are still an important entry point into aquatic and terrestrial systems, given the high volumes involved and the amount of sludge reused via land application. The major concerns are with small particles (especially <∼150 μm) in discharged wastewater effluent and the impact that particles which accumulate in sewage sludge may have on terrestrial ecosystems.
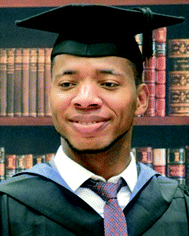 Paul Iyare | Paul Iyare is a Researcher, Environmental Engineer, and Environmental Professional-in-Training with ECO Canada. His research interests include environmental pollution and remediation, with particular emphasis on microplastics. He obtained an MSc in Water and Environmental Engineering from the University of Surrey and graduated with a Distinction. He also holds a bachelor's degree in Fisheries (first class honours), with multiple awards from the University of Benin. |
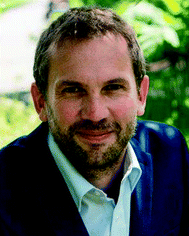 Tom Bond | Tom Bond is a Lecturer in the Department of Civil and Environmental Engineering at the University of Surrey and a Chartered Member of the Institution of Civil Engineers. His research concerns mitigating the impacts of hazardous aquatic pollutants. Prior to joining Surrey he held an Imperial College Research Fellowship and he has a first-class MSci in chemistry from Bristol University. |
Water impact
Secondary and tertiary wastewater treatment plants removed an average of 88% and 94% of microplastics, respectively; the majority during preliminary and primary treatment. Key impacts from microplastics in wastewater are predicted to be on terrestrial ecosystems, due to their accumulation in sewage sludge, and associated with small particles (especially < approximately 150 μm) in discharged wastewater effluent.
|
1. Introduction
The accumulation of plastic litter in aquatic ecosystems is now a well-known problem. Microplastics are typically characterised as plastic particles from 1 nm to <5 mm in size.1 They can either result from the degradation and weathering of larger plastic items (secondary microplastics), or from the direct discharge of materials originally manufactured at that size (primary microplastics), for example, microbeads used in facial scrub cleansers.2 The durability and resilience of plastics are major reasons why they pose a hazardous threat to the aquatic ecosystems.3
The global occurrence of microplastics in marine and freshwater environments has been affirmed by many research findings. Municipal wastewater treatment plant (WWTP) (or sewage treatment plant) effluents are an important route for microplastics to enter the aquatic environment4–20 and can include both primary and secondary microplastics. While WWTPs are not currently designed or optimised for the removal of microplastics, some studies indicate that advanced treatment technologies can improve their removal.11–13,16,21 It can be inferred that the removal efficacy of microplastics in WWTPs will vary depending on the treatment process and the physiochemical properties of the polymer in question (density, particle size, charge, hydrophobicity etc.).22 WWTPs receive wastewater from domestic, industrial and commercial sources, and sometimes also surface run-off. Depending on the country and location, effluent may be released directly into the ocean or into freshwater environments, typically rivers, from where it may be transported to the marine environment.23 Microplastics removed during sewage treatment may be retained in the sludge;5,24 which sometimes is treated and applied to land, such as for agricultural reuse.7,25
While there have been a number of recent reviews about plastics in wastewater treatment,26–29 none of these calculated removal efficiencies for microplastics for relevant treatment processes based on all available literature or settling/floating velocities for polymers commonly found in wastewater. The objective of this study was to analyse the fate and behaviour of microplastics during wastewater treatment, which included quantifying their removal efficiency by different processes and concentrations in sewage sludge.
2. Methods
A focussed literature search for papers on microplastics in WWTPs on scientific databases (specifically ScienceDirect, ACS publications and Google Scholar) was carried out for the terms “microplastic” and “wastewater”. The search yielded 740, 62 and 284 results respectively, out of which 21 research papers, which were considered relevant to the objectives of this study, were selected after reading abstracts and scanning through the contents of the papers. The removal rates, methods of sampling and analysis, and predominant type of detected microplastics from these 21 papers are summarised in Table 1. The percentage removal of different treatment stages (Table 3) and concentration of microplastics in sewage sludge (Table 4) were calculated. In order to make a preliminary assessment of settling/floating velocities of common polymer types during sewage treatment (Fig. 1), the approach of Dietrich30 as has been previously applied to calculate theoretical settling/floating velocities for microplastics in water was followed.31,32 In brief, the first step was to calculate the dimensionless particle diameter (D*) for spherical plastic particles, using: | 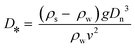 | (1) |
where:ps = polymer density, kg m−3 (see ESI† for values used)pw = density of water, (998.2 kg3 m−1 at 20 °C)33g = acceleration due to gravity (9.81 m s−2)Dn = spherical diameter of particle, (a value of 1.6 mm was used, the largest size of plastic fragments found after primary treatment).34
Table 1 Techniques utilised and microplastic influent/effluent concentrations in selected studies
Study |
Location |
Method of sampling |
Sampled particles size range (μm) |
Method of identification |
Major type of detected microplastics |
Influent microplastic concentration (MP per L) |
Effluent microplastic concentration (MP per L) |
Daily microplastic discharge (MP d−1) |
Primary effluent (in lieu of influent) concentration was used for estimation.
Concentration from three treatment technologies.NB: concentrations separated by commas represent different microplastic types whereas others separated by dashes represent a concentration range. |
35
|
Russia |
Filtration device |
20–300 |
Visual observation |
Fibres, synthetic particles |
467, 160 |
16, 7 |
NA |
5
|
Sweden |
Filtration |
>300 |
Visual observation & FT-IR spectroscopy |
Fibres, fragments |
15 |
8.3 |
3.6 × 104 |
36
|
France |
Automatic sampler |
NA |
Visual observation |
Fibres |
260–320 |
14–50 |
8.4 × 109 |
8
|
Finland |
Filtration device |
20–200 |
Visual observation |
Synthetic particle, textile fibres |
430, 180 |
9, 5 |
3.7 × 109 |
21
|
USA |
Sieving |
20–400 |
Visual observation & FT-IR spectroscopy |
Fibres (polyethylene) |
1 |
∼0.0007 |
9.3 × 105 |
9
|
Scotland |
Steel bucket and steel sieve |
>65 |
Visual observation & FT-IR spectroscopy |
Fibres (polyester, olyamide, acrylic) |
16 |
0.3 |
6.5 × 107 |
24
|
Netherlands |
Glass bottle |
10–5000 |
Visual observation & FT-IR spectroscopy |
Fibres |
68–910 |
52 |
7.5 × 108 − 4.3 × 1010 |
11
|
Germany |
Filtration device |
50–100 |
Visual observation & FPA-based micro-FT-IR imaging |
Fibres (polyethylene) |
ND |
ND |
4.2 × 104 − 1.2 × 107 |
12
|
Finland |
Filtration device |
NA |
Visual observation & FT-IR spectroscopy |
Fibres |
7 |
0.01 |
1.3 × 106 − 6.6 × 107 |
13
|
Australia |
Sampling device |
25–500 |
Visual observation & FT-IR spectroscopy |
Fibres (polyethylene) |
2a |
0.3, 0.2 |
3.6 × 106 − 1.0 × 107 |
14
|
Canada |
Glass jar |
1–65 |
Visual observation & FT-IR spectroscopy |
Fibres, fragments |
14 |
1 |
10.6 × 109 − 19.9 × 109 |
15
|
Turkey |
Sampling device |
1000–5000 |
Visual observation & μ-Raman spectroscopy |
Fibres (polyester) |
4.8 × 106 MP per day; 2.0 × 106 MP per day |
1.2 × 106 MP per day; 0.35 × 106 MP per day |
2.2 × 105 − 1.5 × 106 |
16
|
Finland |
Steel bucket |
250–5000 |
Visual observation, FT-IR & μ-Raman spectroscopy |
Fibres (polyesters, polyethylene, polyamide) |
57.6 |
1, 0.4 |
1.0 × 107 |
37
|
South Korea |
NA |
106–300 |
Visual observation & FT-IR spectroscopy |
Fibre, fragments |
30, 17, 14b |
0.4, 0.1, 0.3b |
NA |
38
|
Denmark |
Sampling device |
10–500 |
Visual observation & FPA-based FT-IR imaging |
Synthetic particle, fibres |
7216 |
54 |
NA |
39
|
Poland |
Plastic canisters |
109–>300 |
Visual observation |
Fibres |
19–552 |
0.028–0.96 |
NA |
17
|
USA |
Glass jar |
NA |
Visual observation & μFT-IR spectroscopy |
Fibres |
ND |
ND |
5.0 × 108 − 1.0 × 109 |
34
|
China |
Steel bucket |
20–4200 |
Visual observation & μ-Raman spectroscopy |
Fibres, fragments |
80 |
28 |
NA |
18
|
China |
Sampling device |
43–5000 |
Visual observation & FT-IR spectroscopy |
Fibres |
6.6 |
0.6 |
6.5 × 108 |
19
|
China |
Sampling device |
25–>500 |
Visual observation & FT-IR spectroscopy |
Fragments, fibres |
4 |
0.1, 0.1 |
6.5 × 106, 3.5 × 106 |
20
|
Italy |
Steel bucket and steel sieve |
10–5000 |
Visual observation & μFT-IR spectroscopy |
Fibres (polyesters, polyamide) |
3 |
0.4 |
1.6 × 108 |
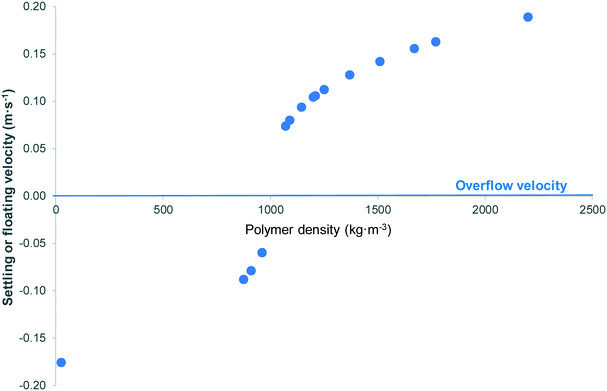 |
| Fig. 1 Theoretical settling or floating velocities of 1.6 mm diameter spherical particles of polymers commonly found in WWTPs (using density values based on Andrady,40 Hidalgo-Ruz et al.,41 and Duis and Coors42). Polymers with density ≤960 kg m−3 (expanded polystyrene, polypropylene, low-density and high-density polyethylene) are predicted to float; remaining polymers, all with density ≥1070 kg m−3, are predicted to be removed by sedimentation. | |
v = kinematic viscosity, (1.003 × 10−6 m2 s−1 °C)33
Subsequently, the dimensionless settling velocity (ω*) was calculated, using:
|  | (2) |
Or:
| log(ω*) = −3.7615 + 1.92944(log D*) − 0.09815(log D*)2 − 0.00575(log D*)3 + 0.00056(log D*)4 | (3) |
For 0.05 ≤
D* ≤ 5 × 10
9
Finally, the theoretical settling velocity (Vs) was calculated, using:
| 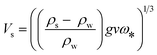 | (4) |
where the relevant terms are as defined above. Stokes' law can be used to directly calculate the terminal settling velocity of low-density spheres with diameter <200 μm.
31 Rearrangement of the version used by Metcalf and Eddy
33 was used for a preliminary estimation of the critical diameter above which spherical polymer particles are expected to be removed by density-driven clarification during primary sedimentation:
| 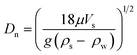 | (5) |
where
μ is the dynamic viscosity (1.003 × 10
−3 kg m
−1 s
−1 at 20 °C,
33) and the other terms are as defined above. When calculating the critical diameter, a settling/floating velocity of 4.6 × 10
−4 m s
−1 was used, which is equivalent to an overflow velocity (or surface loading rate) of 40 m
3 m
−2 per day, typical of primary sedimentation.
33 The surface loading rate is a key design parameter for clarification processes, as if the actual settling velocity of a particle is less than the overflow velocity then it will not be removed by sedimentation. Here it has been assumed that the noted overflow velocity applies to buoyant polymers (which float), as well as non-buoyant polymers (which settle). For calculations of settling/floating velocity it has been assumed that no interactions occur between plastics and other particles present. More experimental research into this topic may be fruitful, in order to validate or improve the approach followed here.
2.1 Observation and identification of microplastics
2.1.1 Visual identification.
Visual examination uses morphological characteristics such as size, shape and colour to identify and characterise microplastics, often with a dissecting microscope i.e. stereomicroscope.43–47 Particles were often classified into shapes (fibres, fragments, foils, foam, spheres, granules, sheets, pellets, etc.), colour (white, black, red, blue, etc.), as well as quantities. Results from four studies were based on visual identification only.8,35,36,39 Others included visual pre-selection of particles prior to spectroscopic identification (FT-IR or Raman spectroscopy). Visual examination prior to analysis represents an important step to isolate plastic particles from materials such as organic debris and other anthropogenic materials (tar, glass, metal paint coatings, etc.).41 However, analysis based on visual observation is prone to bias from overestimation or underestimation by misidentification of particles.41,48 It was difficult to differentiate between natural and synthetic fibres using visual identification alone.36,39 Sample preparation, involving digestion, staining and extraction could decrease the tendency for misidentification.49 The presence of synthetic fibres was reported in all 21 studies investigated. The following criteria was applied by two studies41,50 to visually distinguish synthetic from biological fibres:
i. The fibres had to be equally thick through their entire length.
ii. Fibres should not be entirely straight, which indicates a biological origin.
iii. No cellular or organic structures should be visible in microplastic fibres.
iv. Transparent fibres were examined with higher magnification to confirm their nature. Green fibres were also carefully observed because this colour is very widespread in natural particles.
Another problem associated with using visual identification only is size limitation, as smaller particles (especially 10–30 μm particle sizes) could not be sorted and identified easily even under microscopes and were referred to as “potential” microplastics.20,51 Thus, visual examination may include error rates ranging from 20%52 to 70%,41 which increased with decreasing size of particles.
2.1.2 Polymer identification.
Spectroscopic methods are required for reliable polymer identification in microplastic samples.11,53 FT-IR spectroscopy was the most frequently used technique in analysing microplastics from WWTPs: 11 studies applied FT-IR spectroscopy, 2 focal plane array (FPA)-based FT-IR imaging, 2 micro-FT-IR spectroscopy and 3 micro-Raman spectroscopy (Table 1).
FT-IR identification involves comparing characteristic peaks with those present in a reference spectrum. However, there are often discrepancies between samples and those in reference spectra, as commercially available spectral libraries represent ideal samples, which may not be representative of environmental samples.9 Comparison and identification of microplastics from environmental matrices can be improved by creating a more representative library of weathered/degraded plastics.9,11 Applying visual pre-selection steps prior to FT-IR analysis, using a set of qualitative characteristics, was considered as labour intensive and prone to error.54–58 In some cases, FT-IR was only used for fragments or sub-samples considered ambiguous (on the basis of visual identification).9,21,24,27 Despite comprehensive sample processing (including organic matter digestion, density separation and staining), 22–90% of suspected microplastics were identified as non-plastic particles, following FT-IR analysis.13 Fourier transform infrared microscopy (μFT-IR), a tool that combines FT-IR spectroscopy and microscopy, have been used by recent studies.17,20 μFT-IR gives improved spatial resolution59 relative to more standard types of FT-IR and requires little sample preparation, so can be used to directly identify microplastics which have been separated on membrane filters.58 The need for visual sorting of microplastics was eliminated by introducing a chemical mapping technique using reflectance micro-FT-IR spectroscopy for detecting microplastics in marine sediments.58 They also reported that focal plane array (FPA) detectors reduced the measurement time for complete filter analyses. Further, FPA-based micro-FT-IR has been suggested as a method for unbiased microplastic analysis in environmental samples, including wastewater.11,51,60 By simultaneously obtaining thousands of spectra within minutes, FPA detectors can provide fast identification of even smaller microplastic fragments, without compromising spatial resolution and eliminating the need for the visual pre-selection.51,60,61 This gives a clear advantage compared to analytical methods based on visual pre-selection. Attenuated total reflection FT-IR (ATR-FT-IR) and FPA-based transmission μFT-IR imaging, as well as an enzymatic–oxidative sample preparation were applied to identify polymers of all microplastics down to a size of 20 μm.11 However, the whole-filter analysis was time consuming, up to 10 hours per sample of treated wastewater. This may further lead to compromises like performing partial analysis of the samples in order to process the analysis in a reasonable amount of time.11
Raman spectroscopy has also been frequently used for identifying microplastics.15,16,34 Raman spectroscopy has a better size resolution than FT-IR (down to 1 μm – by automated single-particle exploration coupled to μ-Raman spectroscopy), but fluorescence often impairs the quality of Raman spectra of environmental microplastics. In contrast, FT-IR has a less precise size resolution (down to 10–20 μm – by μ-FTIR spectroscopy), and its spectral quality is not influenced by fluorescence but by the presence of water.62 FT-IR spectroscopy was reported to be faster than Raman spectroscopy,63 while the latter may suffer from interferences resulting from additives (e.g. colorants) or contaminants (including microalgae).63,64 Raman spectroscopy is a valuable alternative to FT-IR spectroscopy when the analysis of wet samples is required or when complementary spectral profiles are desired.60 Micro-Raman spectroscopy has been demonstrated as a reliable tool for polymer identification.65–71
3. Results and discussion
3.1 Overall removal of microplastics in wastewater treatment plants
While sewage treatment plants are not designed to remove microplastics,72 an average removal value of 88% for WWTPs applying preliminary/primary plus secondary treatment, and 94% for WWTPs applying preliminary/primary plus secondary plus tertiary treatment was calculated from the 21 studies summarised in Table 3. The bulk of the removal, an average of 72%, comes during preliminary and primary treatment. Although overall removal is high, the residual amount in treated effluent (∼10% of the microplastics in influent wastewater) nonetheless represents an important release of microplastics to the aquatic environment, given the large volumes of effluent involved. Data from Table 1 indicates that from 0.0–54 particles per litre (MP per L) microplastic particles were discharged into the receiving water body daily from treated wastewater. Corresponding influent concentrations of microplastics ranged from 1 to 7216 MP per L.
Note the high variability in both influent and effluent concentrations of microplastics. A large proportion of this can be explained by the variability in polymer sampling, isolation and detection methods, as discussed above. This is illustrated by the lower end of the size range of plastic particles sampled, isolated and identified in selected studies, which ranged from 1 to 1000 μm (Table 1). Due to the variability in the experimental methods deployed, comparing removal values for microplastics across multiple studies should be viewed as approximate. Nonetheless, the data presented in Table 3 does provide an important indication of the efficacy of WWTPs for the removal of microplastics.
Based on data provided by Talvitie et al.,8 Murphy et al.9 and Magni et al.,20 intra-study experimental variability in the number of microplastic particles, as quantified by the standard deviation of replicate samples, ranged from 12–33%, with a mean value of 25%. The authors of the current study are unaware of comparisons of variability in experimental microplastic measurements between multiple studies. However, they are presumably somewhat higher than the quoted intra-study values, given the current lack of standardised methods for sampling, sample processing and polymer identification. In the current study, percentage microplastic removal was selected as the principal means of quantifying the performance of wastewater treatment processes, but it should be kept in mind that direct comparison between different studies is problematic and that the removal values presented here are approximate.
3.1.1 Preliminary and primary treatment.
Preliminary treatment encompasses the removal of materials such as rags, sticks, floatables, grit and grease that may impair or inhibit the operation of downstream processes.33 Preliminary and primary treatment processes in the selected studies included coarse and fine screening, grit and grease removal, skimming and primary settlement (sedimentation) (Table 2). Screens of varying sizes – typically coarse (6 to 150 mm) and fine (less than 6 mm) – retain suspended and floating solids,33 so plastic particles larger than this are expected to be removed during preliminary treatment. This is consistent with data from Liu et al.,34 in which a screen mesh size of 6 mm was used, who reported that the largest size of plastic fragments after primary treatment was 1.6 mm.
Table 2 Wastewater treatment processes present in selected studies
Study |
Primary treatments |
Secondary treatment |
Tertiary treatment |
No secondary settling involved.MBR: membrane bioreactor; DF: disc-filter, RSF: rapid sand filter; DAF: dissolved air flotation. |
35
|
Screening, grit and grease removal, settling |
Activated sludge |
|
5
|
Screening, grit and grease removal, settling |
Activated sludge |
|
36
|
Screening, grit and grease removal, settling |
Biofiltrationa |
|
8
|
Screening, grit and grease removal, pre-aeration, settling |
Activated sludge |
Biological filtration |
21
|
Screening, grit and grease removal, skimming, settling |
Activated sludge |
Gravity filtration |
9
|
Screening, grit and grease removal, skimming, settling |
Activated sludge |
|
24
|
Screening, grit and grease removal, settling |
Activated sludge |
|
11
|
Screening, grit and grease removal, skimming, settling |
Activated sludge |
Gravity filtration |
12
|
Screening, grit and grease removal, skimming, settling |
Activated sludge |
DF, RSF, DAF, MBR |
13
|
Screening, grit and grease removal, skimming, settling |
Activated sludge |
Ultrafiltration, reverse osmosis |
14
|
Screening, grit and grease removal, settling |
Trickling filters, solids contact tanks |
|
15
|
Screening, grit and grease removal, settling |
Activated sludge |
|
16
|
Screening, grit and grease removal, settling |
Activated sludge |
MBR |
37
|
Screening, grit and grease removal, settling |
Activated sludge |
|
38
|
Screening, grit and grease removal, settling |
Activated sludge |
|
39
|
Screening, grit and grease removal, settling |
Activated sludge |
|
17
|
Screening, grit and grease removal, skimming, settling |
Activated sludge |
|
34
|
Screening, grit and grease removal, settling |
Activated sludge |
|
18
|
Screening, grit and grease removal, settling |
Activated sludge |
|
19
|
Screening, grit and grease removal, settling |
Activated sludge (oxidation ditch) |
MBR |
20
|
Screening, grit and grease removal, settling |
Activated sludge |
Gravity filtration |
From Table 3, an average of 72% (range 32–93%) of microplastic particles were removed during the preliminary and primary wastewater treatment. Data from WWTPs in Russia,35 Finland8 and Canada14 showed that preliminary and primary wastewater treatment removed 92–93% of fibres. Talvitie et al.8 found that fibres were largely removed during primary sedimentation, while removal during secondary sedimentation and biological filtration was insignificant in comparison. Further, Michielssen et al.73 reported that screening and primary sedimentation removed 84–88% of small anthropogenic litter. To illustrate this point further, analysis of microplastics retained by a secondary WWTP showed that the largest reduction in microplastics was during grit and grease removal treatment stage, 45%, followed by primary sedimentation, 34%.9 These data are consistent with the results of the settling/floating velocity calculations displayed in Fig. 1 and Table S1.† For polymers which have been commonly reported as components of the microplastics found in WWTPs, spherical particles of diameter 1.6 mm are predicted either to be completed removed by floatation (polymers of density ≤960 kg m−3, i.e. expanded polystyrene, polypropylene, low-density and high-density polyethylene) or sedimentation; (remaining polymers, all with density ≥1070 kg m−3). Based on an overflow velocity of 40 m3 m−2 per day, spherical polymer particles of diameter above 27–149 μm, depending on the particular polymer, are predicted to be removed during primary sedimentation (Table S1†). Note that these values are estimations, as they do not account for deviation from non-ideal behaviour of, for example, highly irregular particles or those fouled with biofilm, or of alternative removal mechanisms such as sorption. Nonetheless, they do emphasise the general point that larger particles, which in this context can be defined as those >∼150 μm, are well-removed by density-driven separation in preliminary and primary wastewater treatment. It is smaller particles which will comprise the bulk of microplastics entering subsequent treatment stages. A complicating factor is that smaller particles are also more difficult to isolate and analyse, so many will be missed during experimental surveys, even using state-of-the-art techniques which have a spatial resolution as low as 10–20 μm (see section 2.1.2).
Table 3 Removal of microplastics during wastewater treatment in selected studies
Study |
Primary |
Secondary |
Tertiary & advanced |
Primary + secondary |
Primary + secondary + tertiary |
Primary effluent (in lieu of influent) concentration was used for estimation.
|
35
|
87–93 |
4–9 |
|
96–97 |
|
5
|
|
|
|
99.9 |
|
36
|
69 |
19 |
|
88 |
|
8
|
32–92 |
0.2–52 |
5–14 |
|
97–98 |
21
|
|
|
|
|
99.9 |
9
|
78 |
20 |
|
98 |
|
24
|
|
|
|
72 |
|
11
|
|
|
|
|
95.5 |
12
|
|
|
|
|
99.9 |
13
|
|
|
|
|
90 |
14
|
92 |
7 |
|
98 |
|
15
|
|
|
|
76 |
|
16
|
|
|
|
98.3 |
99.4 |
37
|
|
|
|
98.5 |
|
38
|
|
|
|
99.3 |
|
39
|
|
|
|
98.2 |
|
17
|
|
|
|
91.4 |
|
34
|
41 |
24 |
|
64 |
|
18
|
|
|
|
90.5 |
|
19
|
|
|
|
53.6 |
82.1 |
20
|
NA |
NA |
20 |
|
84 |
Mean (n) |
72% (6) |
17% (6) |
15% (2) |
88% (15) |
94% (8) |
3.1.2 Secondary treatment.
Secondary treatment operations are typically biological processes which further reduce the residual suspended and dissolved solids remaining in wastewater after primary treatment.74 In 19 of the 21 studies, secondary treatment comprised variations of the activated sludge process (Table 2), whereas Dris et al.36 applied biofiltration and Gies et al.14 utilised trickling filters and solids contact tanks. Secondary treatment removes additional microplastics through entrapment in solid flocs,21 sedimentation in secondary clarifiers, or even by ingestion by microorganisms, e.g. protozoa or metazoan75,76 present. Ferric sulfate or other flocculants used in secondary sedimentation have been suspected to aid the removal of microplastics through aggregation of particulate matter into flocs.9 However, the mechanism of the interaction between microplastics and flocs, and the degree to which they aid microplastic removal has not, as far as we are aware, been determined. It is probable that microplastics trapped in unstable flocs may not be effectively removed, resulting in a dynamic redistribution of the particles and their subsequent escape during clarification.21 Lee and Kim37 suggested that smaller microplastics (106–300 μm) have a higher removal efficacy compared to larger microplastics (>300 μm), because they are not only retained in grit and grease removal stage, but also easily adsorbed to sticky media such as biofilm or floc. Liu et al.34 suggested that turbidity removal, from secondary treatment as well as primary sedimentation, had a closer relationship with microplastic removal than other parameters.
Overall, secondary treatment removed a further 16% of microplastics, on average, (0.2–52%) relative to preliminary and primary treatment (Table 3). The activated sludge process recorded an average removal rate of 16% (0.2–52%). Biofiltration was more effective for microplastic removal (19%) compared to trickling filters and solids contact tanks (7%). Talvitie et al.8 found that the removal of fibres was insignificant compared to the removal of synthetic particles during secondary sedimentation and the reverse was recorded during primary sedimentation. For example, 92% and 32% of textile fibres and synthetic particles were respectively removed by primary treatment, with equivalent values 0.2% and 52% during secondary treatment. HELCOM35 also recorded a higher removal of particles compared with textile fibres during secondary treatment.
Overall, preliminary/primary and secondary wastewater treatment removed an average of 88% of microplastics (Table 3), although far higher removals have been reported by individual WWTPs. Magnusson and Norén5 recorded a 99.9% removal rate for a relatively small (12
000 population equivalents) Swedish WWTP, based on one sampling event. Lee and Kim37 compared the microplastic removal efficacies of three different configurations of the activated sludge process: the anaerobic–anoxic–aerobic (A2O), sequence batch reactor (SBR), and media process (an anaerobic, anoxic, and aerobic basin that uses a filled carrier). All processes were found to have removal efficacies above 98%, with the SBR process recording the highest rate of 99.2%. Although, experiments from a lab-scale sequencing batch biological WWTP reported a low removal rate of 52%, and smaller particles (up to 60–70 μm) were retained in the activated sludge, while the larger particles were found in the effluent.77 However, the study focused on the fate of polyethylene microbeads and not a complex mixture of microplastics as found in authentic wastewater.
3.1.3 Tertiary and advanced treatment.
Tertiary treatment technologies are used to remove specific inorganic and organic pollutants beyond levels attainable by conventional secondary treatment processes, typically as required by a particular discharge consent or reuse requirement.33 The technologies used in the selected tertiary WWTPs included variations of filtration processes including depth, surface, biological and membrane filtration, as well as dissolved air flotation (DAF; Table 2). Tertiary treatment reduced the concentration of microplastics by a further 5–20%, relative to the level of removal achieved by secondary treatment. On average, tertiary WWTPs removed 94% (range 82–99%) of microplastics in sewage influent (Table 3). Talvitie et al.12 investigated the removal efficacies of microplastics (>20 μm) from tertiary treatment technologies commonly used in Finland: disc-filter (DF), rapid sand filter (RSF) and DAF treating secondary effluent, and MBR treating primary effluent. Samples were collected before and after the tertiary treatment steps. MBR produced the highest percentage removal (99.9%) whereas RSF, DAF and DF removed 97%, 95% and 40–98.5% respectively. Although the MBR was treating primary clarified effluent with a much higher microplastic concentration than the other processes, which received secondary effluent, this technology still gave the lowest concentration of microplastics in final effluent. The MBR filters had the finest pore size (0.4 μm) of all the filters in the study. Furthermore, Lares et al.16 used a pilot-scale MBR and reported 99.4% removal of microplastics. The plant consisted of an anaerobic tank, aerobic tank, as well as a membrane filtration tank with a submerged MBR unit with a pore size of 0.4 μm. Other studies have reported lower removal using MBRs as a tertiary treatment: Lv et al.19 reported a 99.5% removal based on plastic mass but 82.1% based on the number of microplastics. Removal efficacies based on microplastic particle numbers were negatively impacted by certain unit operation, e.g. grit removal, which resulted in microplastic fragmentation and an increase in particle numbers. Thus, these authors suggested that estimated that microplastic removal should be based on mass, as well as particle number. Ultrafiltration and reverse osmosis (RO) completely removed particles larger than 190 μm, whereas the smallest size fractions (<190 μm) were reported as the most abundant after tertiary treatment.13 Also, a post-filtration system of 12 rolling filters of pile fabric removed MP >500 μm completely but could only remove 93% of microplastics <500 μm in a German sewage treatment works.11
Conversely, while, Carr et al.21 recorded 99.9% removal rate across the whole WWTP, microplastics were mainly removed in the primary treatment stage, especially through solids skimming and sludge settling processes, and the effect of gravity filters was minimal. Biofiltration systems involving upstream denitrification (UD), downstream denitrification (DD) and combined upstream–downstream denitrification (U–DD) further removed 5% of textile fibres and 14% of synthetic particles, relative to the removal achieved by secondary treatment (Table 3).8 Liu et al.78 assessed the efficiency of a pilot-scale biofilter to remove microplastics from WWTP effluent. 79–89% of microplastics were removed and all microplastic particles larger than 100 μm were retained. These data are consistent with results from full-scale wastewater treatment plants (Table 3). Sand filtration decreased the microplastic concentration of secondary treated effluent by over 50%, from 0.9 ± 0.3 MPs per L to 0.4 ± 0.1 MPs per L.20 Compared to membrane filtration, this is a simple and cost-effective treatment method. Reliable estimation of microplastics removal efficacy by tertiary treatment processes require larger volumes of samples than for pre-treatment and secondary treatment processes.27 This is because microplastic concentrations from tertiary treatment units may be very low (<1 particle per L), leading to false zero results from limited sample volumes.
3.2 Microplastic concentrations in sewage sludge
The residual solids separated during various stages of wastewater treatment are known as sewage sludge. More than 10 million tonnes of sewage sludge were generated in WWTPs in the EU in 2010.79 The sludge produced is pumped to sludge treatment facilities such as thickening, dewatering and anaerobic digestion. Fat, oil and grease (FOG), typically separated by skimming during preliminary treatment, makes sludge more viscous and waxy, thus reducing its dewatering efficiency.80 Sludge which contains FOG is often incinerated or landfilled.81 As discussed above, it is likely that the majority of microplastics removed during wastewater treatment accumulate in sludge. This is illustrated by Magnusson and Norén5 who reported that the amount of microplastic particles in the wastewater effluent entering a Swedish WWTP with a load of 12
000 population equivalent (PE) was 15
000 microplastic particles per m3. More than 99% of this amount was retained in the sewage sludge while the concentration in the treated effluent was only 8 microplastics per m3. Li et al.82 suggested that laundry and plastic-industry wastewater may be the principal microplastic sources in sewage sludge. Consistent with this, plastic microfibres have been reported to dominate the composition of microplastics in sludge. Fibres comprised 90% of the total plastics reported by Corradini et al.,83 76% by Mahon et al.,84 70% by Magnusson and Norén5 and 63% by Li et al.82 Other items found included shafts, fragments, films, flakes and spheres. From Table 4, microplastic counts of 720–14
900 particles per kg (wet weight) have been reported from sewage sludge samples in various studies. Based on these numbers, an approximate estimation for the number of plastic particles present in the 10 million tonnes of sewage sludge generated each year in the EU is in the region of 7.2 × 1012 − 1.49 × 1014 per year. Similarly, Li et al.82 estimated that the average number of microplastic particles entering the natural environment in sewage sludge in China as 1.56 × 1014 particles per year.
Table 4 Microplastic concentrations in sewage sludge
Study |
Location |
Sampling point |
Microplastic size range (μm) |
Concentration (particle per kg) |
Wet weight.Note: concentrations based on dry weight of sludge. |
5
|
Sweden |
Slightly dewatered sludge |
300–5000 |
16 700 |
720a |
24
|
Netherlands |
Sewage sludge |
10–5000 |
510–760a |
11
|
Germany |
Sewage sludge |
<500 |
1000–24 000 |
14
|
Canada |
Primary sludge |
1–65 |
14 900a |
|
|
Secondary sludge |
|
4400a |
16
|
Finland |
Activated sludge |
250–1000 |
23 000 |
|
|
Digested sludge |
|
170 900 |
|
|
MBR sludge |
|
27 300 |
37
|
South Korea |
Anaerobic–anoxic–aerobic |
106–300 |
13 275 |
>300 |
1620 |
|
|
Sequence batch reactor |
106–300 |
7340 |
>300 |
2315 |
|
|
Media process |
106–300 |
10 615 |
>300 |
2585 |
39
|
Poland |
Sewage sludge |
109–5000 |
6700–62 600 |
34
|
China |
Dewatered sludge |
20–000 |
240 300 |
20
|
Italy |
Recycled activated sludge |
10–5000 |
113 000 |
On a dry weight basis, a higher microplastic sludge concentration range 1000–170
900 particles per kg has been reported in literature (Table 4). The sizes of microplastics retained in the sludge of a large secondary WWTP in Glasgow, with a load of 650
000 PE, were considerably larger than those obtained from various sampling points in the WWTP.9 This again emphasises that larger polymer particles are more easily removed through settlement operations in WWTPs. In investigating the effects of sludge treatment processes, Mahon et al.84 found that microplastics in smaller size classes were high in lime stabilisation samples (e.g. 0.2 g kg−1 in the <45 μm size fraction), suggesting that the treatment process shears microplastic particles. However, a lesser abundance of microplastics observed in anaerobic digestion samples (e.g. 0 g kg−1 in the <45 μm size fraction) suggested that the process may decrease microplastic abundances. Corradini et al.83 evaluated 31 agricultural fields in Chile with different sludge application records, covering a period of 10 years, and reported that successive sludge application on agricultural fields resulted in microplastic accumulation over time. Microplastic content in sludge of the agricultural fields ranged from 18
000 to 41
000 particle per kg. It is expected that microplastics in soil enter the aquatic environment through surface runoff, irrigation or wind.85,86
4. Conclusions
More accurate comparison of data across multiple studies would be facilitated by at least some standardisation of experimental methods for the extraction, isolation, and identification of microplastics in complex environmental matrices. Nonetheless, based on critical analysis of scientific literature on microplastics in WWTPs and sewage sludge, the following conclusions can be drawn:
• Preliminary and primary treatment, especially sedimentation and solids skimming, removed 72%, on average, of plastic particles present in sewage influent.
• Calculations of the settling/floating velocities of spherical polymer particles predict that those of diameter >27–149 μm, depending on the particular polymer, are removed during primary sedimentation. Thus, the majority of microplastics in sewage effluent discharged to the environment are likely to be smaller particles.
• Secondary and tertiary WWTPs removed respectively 88% and 94%, on average, of the number of microplastic particles present in sewage influent. Even though these values are high, the amount of sewage effluent discharged means that WWTPs still contribute significant amounts of microplastic pollution to the environment.
• Compared with other advanced (tertiary) treatment technologies, MBRs demonstrated a higher potential for more effective microplastic removal, from 82.1–99.9%.
• The majority of plastics removed during sewage treatment are retained in sewage sludge. A wide range of concentrations for microplastics in sewage sludge have been reported in literature: from 720–14
900 particles per kg (wet weight) and from 1000–170
900 particles per kg (dry weight). The majority, from 63–90%, of microplastics in sludge were fibres. Large numbers of plastic particles enter the terrestrial environment where sludge is reused for agriculture. More research is needed on the environmental fate and impact of plastics in sludge-amended soils, in particular where agricultural reuse of sewage sludge is common practice.
Conflicts of interest
There are no conflicts to declare.
Acknowledgements
Paul Iyare was supported by a Niger Delta Development Commission of Nigeria postgraduate scholarship. Tom Bond would like to acknowledge the Engineering and Physical Sciences Research Council (EPSRC) for supporting his New Investigator Award project on predicting the polymer-specific fate of aquatic plastic litter (EP/S029427/1).
References
-
GESAMP, Sources, fate and effects of microplastics in the marine environment: A global assessment, (IMO/FAO/UNESCO-IOC/UNIDO/WMO/IAEA/UN/UNEP/UNDP Joint Group of Experts on the Scientific Aspects of Marine Environmental Protection), Rep. Stud. GESAMP, London, 90, 2015 Search PubMed.
- M. Chang, Reducing microplastics from facial exfoliating cleansers in wastewater through treatment versus consumer product decisions, Mar. Pollut. Bull., 2015, 101(1), 330–333 CrossRef PubMed.
-
N. A. C. Welden, Microplastic pollution in the Clyde sea area: a study using the indicator species Nephrops norvegicus, PhD. Thesis, University of Glasgow, 2015 Search PubMed.
- M. Cole, P. Lindeque, C. Halsband and T. S. Galloway, Microplastics as contaminants in the marine environment: A review, Mar. Pollut. Bull., 2011, 62(12), 2588–2597 CrossRef PubMed.
-
K. Magnusson and F. Norén, Screening of microplastic particles in and down-stream a wastewater treatment plant, IVL Swedish Environmental Research Institute, Stockholm, 2014 Search PubMed.
- M. Wagner, C. Scherer, D. Alvarez-Muñoz, N. Brennholt, X. Bourrain, S. Buchinger, E. Fries, C. Grosbois, J. Klasmeier and T. Marti, Microplastics in freshwater ecosystems: what we know and what we need to know, Environ. Sci. Eur., 2014, 26(1), 12 CrossRef PubMed.
- D. Eerkes-Medrano, R. C. Thompson and D. C. Aldridge, Microplastics in freshwater systems: a review of the emerging threats, identification of knowledge gaps and prioritisation of research needs, Water Res., 2015, 75, 63–82 CrossRef CAS PubMed.
- J. Talvitie, M. Heinonen, J. P. Paakkonen, E. Vahtera, A. Mikola, O. Setala and R. Vahala, Do wastewater treatment plants act as a potential point source of microplastics? Preliminary study in the coastal Gulf of Finland, Baltic Sea, Water Sci. Technol., 2015, 72(9), 1495–1504 CrossRef CAS PubMed.
- F. Murphy, C. Ewins, F. Carbonnier and B. Quinn, Wastewater Treatment Works (WwTW) as a Source of Microplastics in the Aquatic Environment, Environ. Sci. Technol., 2016, 50(11), 5800–5888 CrossRef CAS PubMed.
- S. Ziajahromi, P. A. Neale and F. D. Leusch, Wastewater treatment plant effluent as a source of microplastics: review of the fate, chemical interactions and potential risks to aquatic organisms, Water Sci. Technol., 2016, 74(10), 2253–2269 CrossRef CAS PubMed.
- S. M. Mintenig, I. Int-Veen, M. G. J. Löder, S. Primpke and G. Gerdts, Identification of microplastic in effluents of waste water treatment plants using focal plane array-based micro-Fourier-transform infrared imaging, Water Res., 2017, 108, 365–372 CrossRef CAS PubMed.
- J. Talvitie, A. Mikola, A. Koistinen and O. Setälä, Solutions to microplastic pollution–Removal of microplastics from wastewater effluent with advanced wastewater treatment technologies, Water Res., 2017, 123, 401–407 CrossRef CAS.
- S. Ziajahromi, P. A. Neale, L. Rintoul and F. D. L. Leusch, Wastewater treatment plants as a pathway for microplastics: Development of a new approach to sample wastewater-based microplastics, Water Res., 2017, 112, 93–99 CrossRef CAS.
- E. A. Gies, J. L. LeNoble, M. Noël, A. Etemadifar, F. Bishay, E. R. Hall and P. S. Ross, Retention of microplastics in a major secondary wastewater treatment plant in Vancouver, Canada, Mar. Pollut. Bull., 2018, 133, 553–561 CrossRef CAS.
- S. Gündoğdu, C. Çevik, E. Güzel and S. Kilercioğlu, Microplastics in municipal wastewater treatment plants in Turkey: a comparison of the influent and secondary effluent concentrations, Environ. Monit. Assess., 2018, 190(11), 626 CrossRef.
- M. Lares, M. C. Ncibi, M. Sillanpää and M. Sillanpää, Occurrence, identification and removal of microplastic particles and fibers in conventional activated sludge process and advanced MBR technology, Water Res., 2018, 133, 236–246 CrossRef PubMed.
- K. Conley, A. Clum, J. Deepe, H. Lane and B. Beckingham, Wastewater treatment plants as a source of microplastics to an urban estuary: Removal efficiencies and loading per capita over one year, Water Research X, 2019, 3, 100030 CrossRef PubMed.
- Z. Long, Z. Pan, W. Wang, J. Ren, X. Yu, L. Lin, H. Lin, H. Chen and X. Jin, Microplastic abundance, characteristics, and removal in wastewater treatment plants in a coastal city of China, Water Res., 2019, 155, 255–265 CrossRef PubMed.
- X. Lv, Q. Dong, Z. Zuo, Y. Liu, X. Huang and W.-M. Wu, Microplastics in a municipal wastewater treatment plant: Fate, dynamic distribution, removal efficiencies, and control strategies, J. Cleaner Prod., 2019, 225, 579–586 CrossRef.
- S. Magni, A. Binelli, L. Pittura, C. G. Avio, C. Della Torre, C. C. Parenti, S. Gorbi and F. Regoli, The fate of microplastics in an Italian Wastewater Treatment Plant, Sci. Total Environ., 2019, 652, 602–610 CrossRef PubMed.
- S. A. Carr, J. Liu and A. G. Tesoro, Transport and fate of microplastic particles in wastewater treatment plants, Water Res., 2016, 91, 174–182 CrossRef CAS PubMed.
- T. Bond, V. Ferrandiz-Mas, M. Felipe-Sotelo and E. van Sebille, The occurrence and degradation of aquatic plastic litter based on polymer physicochemical properties: A review, Crit. Rev. Environ. Sci. Technol., 2018, 48(7–9), 685–722 CrossRef.
- A. McCormick, T. J. Hoellein, S. A. Mason, J. Schluep and J. J. Kelly, Microplastic is an Abundant and Distinct Microbial Habitat in an Urban River, Environ. Sci. Technol., 2014, 48(20), 11863–11871 CrossRef CAS.
- H. A. Leslie, S. H. Brandsma, M. J. van Velzen and A. D. Vethaak, Microplastics en route: Field measurements in the Dutch river delta and Amsterdam canals, wastewater treatment plants, North Sea sediments and biota, Environ. Int., 2017, 101, 133–142 CrossRef CAS.
- M. C. Rillig, Microplastic in terrestrial ecosystems and the soil?, Environ. Sci. Technol., 2012, 46(12), 6453–6454 CrossRef CAS.
- P. L. Ngo, B. K. Pramanik, K. Shah and R. Roychand, Pathway, classification and removal efficiency of microplastics in wastewater treatment plants, Environ. Pollut., 2019, 255, 113326 CrossRef CAS PubMed.
- J. Sun, X. Dai, Q. Wang, M. C. van Loosdrecht and B.-J. Ni, Microplastics in wastewater treatment plants: Detection, occurrence and removal, Water Res., 2019, 152, 21–37 CrossRef CAS.
- X. Zhang, J. Chen and J. Li, The removal of microplastics in the wastewater treatment process and their potential impact on anaerobic digestion due to pollutants association, Chemosphere, 2020, 126360 CrossRef CAS.
- Z. Zhang and Y. Chen, Effects of microplastics on wastewater and sewage sludge treatment and their removal: a review, Chem. Eng. J., 2020, 382, 122955 CrossRef.
- W. E. Dietrich, Settling velocity of natural particles, Water Resour. Res., 1982, 18(6), 1615–1626 CrossRef.
- N. Kowalski, A. M. Reichardt and J. J. Waniek, Sinking rates of microplastics and potential implications of their alteration by physical, biological, and chemical factors, Mar. Pollut. Bull., 2016, 109(1), 310–319 CrossRef CAS PubMed.
- M. Kooi, E. H. V. Nes, M. Scheffer and A. A. Koelmans, Ups and downs in the ocean: effects of biofouling on vertical transport of microplastics, Environ. Sci. Technol., 2017, 51(14), 7963–7971 CrossRef CAS PubMed.
-
Metcalf and Eddy Inc., G. Tchobanoglous, H. D. Stensel, R. Tsuchihashi and F. L. Burton, Wastewater engineering: treatment and resource recovery, McGraw-Hill Higher Education, New York, 2014 Search PubMed.
- X. Liu, W. Yuan, M. Di, Z. Li and J. Wang, Transfer and fate of microplastics during the conventional activated sludge process in one wastewater treatment plant of China, Chem. Eng. J., 2019, 362, 176–182 CrossRef CAS.
-
J. Talvitie and M. Heinonen, HELCOM BASE project 2012–2014: Preliminary study on synthetic microfibers and particles at a municipal waste water treatment plant, Baltic Marine Environment Protection Commission (HELCOM), Helsinki, 2014 Search PubMed.
- R. Dris, J. Gasperi, V. Rocher, M. Saad, N. Renault and B. Tassin, Microplastic contamination in an urban area: a case study in Greater Paris, Environ. Chem., 2015, 12(5), 592–599 CrossRef CAS.
- H. Lee and Y. Kim, Treatment characteristics of microplastics at biological sewage treatment facilities in Korea, Mar. Pollut. Bull., 2018, 137, 1–8 CrossRef CAS PubMed.
- M. Simon, N. van Alst and J. Vollertsen, Quantification of microplastic mass and removal rates at wastewater treatment plants applying Focal Plane Array (FPA)-based Fourier Transform Infrared (FT-IR) imaging, Water Res., 2018, 142, 1–9 CrossRef CAS.
- E. Wiśniowskaa, K. Moraczewska-Majkutb and W. Nocońb, Efficiency of microplastics removal in selected wastewater treatment plants–preliminary studies, Desalin. Water Treat., 2018, 134, 316–323 CrossRef.
- A. L. Andrady, Microplastics in the marine environment, Mar. Pollut. Bull., 2011, 62(8), 1596–1605 CrossRef CAS.
- V. Hidalgo-Ruz, L. Gutow, R. C. Thompson and M. Thiel, Microplastics in the marine environment: a review of the methods used for identification and quantification, Environ. Sci. Technol., 2012, 46(6), 3060–3075 CrossRef CAS.
- K. Duis and A. Coors, Microplastics in the aquatic and terrestrial environment: sources (with a specific focus on personal care products), fate and effects, Environ. Sci. Eur., 2016, 28(1), 2 CrossRef.
- D. G. Shaw and R. H. Day, Colour-and form-dependent loss of plastic micro-debris from the North Pacific Ocean, Mar. Pollut. Bull., 1994, 28(1), 39–43 CrossRef.
- C. J. Moore, S. L. Moore, S. B. Weisberg, G. L. Lattin and A. F. Zellers, A comparison of neustonic plastic and zooplankton abundance in southern California's coastal waters, Mar. Pollut. Bull., 2002, 44(10), 1035–1038 CrossRef CAS.
- S. Morét-Ferguson, K. L. Law, G. Proskurowski, E. K. Murphy, E. E. Peacock and C. M. Reddy, The size, mass, and composition of plastic debris in the western North Atlantic Ocean, Mar. Pollut. Bull., 2010, 60(10), 1873–1878 CrossRef PubMed.
- L. M. Rios, P. R. Jones, C. Moore and U. V. Narayan, Quantitation of persistent organic pollutants adsorbed on plastic debris from the Northern Pacific Gyre's “eastern garbage patch”, J. Environ. Monit., 2010, 12(12), 2226–2236 RSC.
- M. J. Doyle, W. Watson, N. M. Bowlin and S. B. Sheavly, Plastic particles in coastal pelagic ecosystems of the Northeast Pacific ocean, Mar. Environ. Res., 2011, 71(1), 41–52 CrossRef CAS PubMed.
-
M. G. Löder and G. Gerdts, in Marine anthropogenic litter, Springer, 2015, pp. 201–227 Search PubMed.
- S. A. Mason, D. Garneau, R. Sutton, Y. Chu, K. Ehmann, J. Barnes, P. Fink, D. Papazissimos and D. L. Rogers, Microplastic pollution is widely detected in US municipal wastewater treatment plant effluent, Environ. Pollut., 2016, 218, 1045–1054 CrossRef CAS.
-
Sweden KIMO, Small plastic particles in Coastal Swedish waters, N-Research report, commissioned by KIMO Sweden (Submitted to BDC), Sweden, 2007 Search PubMed.
- M. G. J. Löder, M. Kuczera, S. Mintenig, C. Lorenz and G. Gerdts, Focal plane array detector-based micro-Fourier-transform infrared imaging for the analysis of microplastics in environmental samples, Environ. Chem., 2015, 12(5), 563–581 CrossRef.
- M. Eriksen, S. Mason, S. Wilson, C. Box, A. Zellers, W. Edwards, H. Farley and S. Amato, Microplastic pollution in the surface waters of the Laurentian Great Lakes, Mar. Pollut. Bull., 2013, 77(1–2), 177–182 CrossRef CAS PubMed.
- T. Rocha-Santos and A. C. Duarte, A critical overview of the analytical approaches to the occurrence, the fate and the behavior of microplastics in the environment, TrAC, Trends Anal. Chem., 2015, 65, 47–53 CrossRef CAS.
- R. C. Thompson, Y. Olsen, R. P. Mitchell, A. Davis, S. J. Rowland, A. W. John, D. McGonigle and A. E. Russell, Lost at sea: where is all the plastic?, Science, 2004, 304(5672), 838 CrossRef CAS.
- K. Ng and J. Obbard, Prevalence of microplastics in Singapore's coastal marine environment, Mar. Pollut. Bull., 2006, 52(7), 761–767 CrossRef CAS PubMed.
- M. S. Reddy, S. Basha, S. Adimurthy and G. Ramachandraiah, Description of the small plastics fragments in marine sediments along the Alang-Sosiya ship-breaking yard, India, Estuarine, Coastal Shelf Sci., 2006, 68(3–4), 656–660 CrossRef.
- P. L. Corcoran, M. C. Biesinger and M. Grifi, Plastics and beaches: a degrading relationship, Mar. Pollut. Bull., 2009, 58(1), 80–84 CrossRef CAS PubMed.
- J. P. Harrison, J. J. Ojeda and M. E. Romero-González, The applicability of reflectance micro-Fourier-transform infrared spectroscopy for the detection of synthetic microplastics in marine sediments, Sci. Total Environ., 2012, 416, 455–463 CrossRef CAS PubMed.
- J. L. Koenig, S.-Q. Wang and R. Bhargava, FTIR images, Anal. Chem., 2001, 73(13), 361A–369A CrossRef.
- A. S. Tagg, M. Sapp, J. P. Harrison and J. J. Ojeda, Identification and Quantification of Microplastics in Wastewater Using Focal Plane Array-Based Reflectance Micro-FT-IR Imaging, Anal. Chem., 2015, 87(12), 6032–6040 CrossRef CAS.
-
R. Bhargava, S.-Q. Wang and J. L. Koenig, Liquid Chromatography/FTIR Microspectroscopy/Microwave Assisted Synthesis, Springer, Berlin, Heidelberg, 2003, pp. 137–191 Search PubMed.
- L. Cabernard, L. Roscher, C. Lorenz, G. Gerdts and S. Primpke, Comparison of Raman and Fourier transform infrared spectroscopy for the quantification of microplastics in the aquatic environment, Environ. Sci. Technol., 2018, 52(22), 13279–13288 CrossRef CAS.
- M. Cole, H. Webb, P. K. Lindeque, E. S. Fileman, C. Halsband and T. S. Galloway, Isolation of microplastics in biota-rich seawater samples and marine organisms, Sci. Rep., 2014, 4, 4528 CrossRef.
- L. Van Cauwenberghe, A. Vanreusel, J. Mees and C. R. Janssen, Microplastic pollution in deep-sea sediments, Environ. Pollut., 2013, 182, 495–499 CrossRef CAS.
- F. Collard, B. Gilbert, G. Eppe, E. Parmentier and K. Das, Detection of anthropogenic particles in fish stomachs: an isolation method adapted to identification by Raman spectroscopy, Arch. Environ. Contam. Toxicol., 2015, 69(3), 331–339 CrossRef CAS.
- K. Enders, R. Lenz, C. A. Stedmon and T. G. Nielsen, Abundance, size and polymer composition of marine microplastics≥ 10 μm in the Atlantic Ocean and their modelled vertical distribution, Mar. Pollut. Bull., 2015, 100(1), 70–81 CrossRef CAS PubMed.
- L. Frère, I. Paul-Pont, J. Moreau, P. Soudant, C. Lambert, A. Huvet and E. Rinnert, A semi-automated Raman micro-spectroscopy method for morphological and chemical characterizations of microplastic litter, Mar. Pollut. Bull., 2016, 113(1–2), 461–468 Search PubMed.
- A. Käppler, F. Windrich, M. G. Löder, M. Malanin, D. Fischer, M. Labrenz, K.-J. Eichhorn and B. Voit, Identification of microplastics by FTIR and Raman microscopy: a novel silicon filter substrate opens the important spectral range below 1300 cm− 1 for FTIR transmission measurements, Anal. Bioanal. Chem., 2015, 407(22), 6791–6801 CrossRef PubMed.
- B. E. Oßmann, G. Sarau, S. W. Schmitt, H. Holtmannspötter, S. H. Christiansen and W. Dicke, Development of an optimal filter substrate for the identification of small microplastic particles in food by micro-Raman spectroscopy, Anal. Bioanal. Chem., 2017, 409(16), 4099–4109 CrossRef PubMed.
- R. Lenz, K. Enders, C. A. Stedmon, D. M. Mackenzie and T. G. Nielsen, A critical assessment
of visual identification of marine microplastic using Raman spectroscopy for analysis improvement, Mar. Pollut. Bull., 2015, 100(1), 82–91 CrossRef CAS PubMed.
- S. Zhao, M. Danley, J. E. Ward, D. Li and T. J. Mincer, An approach for extraction, characterization and quantitation of microplastic in natural marine snow using Raman microscopy, Anal. Methods, 2017, 9(9), 1470–1478 RSC.
-
E. Schneiderman, Discharging Microbeads to Our Waters: An Examination of Wastewater Treatment Plants in New York, 2015 Search PubMed.
- M. R. Michielssen, E. R. Michielssen, J. Ni and M. B. Duhaime, Fate of microplastics and other small anthropogenic litter (SAL) in wastewater treatment plants depends on unit processes employed, Environ. Sci.: Water Res. Technol., 2016, 2(6), 1064–1073 RSC.
-
H. Patel and R. T. Vashi, in Characterization and Treatment of Textile Wastewater, ed. H. Patel and R. T. Vashi, Elsevier, Boston, 2015, pp. 1–20 Search PubMed.
- C.-B. Jeong, E.-J. Won, H.-M. Kang, M.-C. Lee, D.-S. Hwang, U.-K. Hwang, B. Zhou, S. Souissi, S.-J. Lee and J.-S. Lee, Microplastic Size-Dependent Toxicity, Oxidative Stress Induction, and p-JNK and p-p38 Activation in the Monogonont Rotifer (Brachionus koreanus), Environ. Sci. Technol., 2016, 50(16), 8849–8857 CrossRef CAS PubMed.
-
C. Scherer, A. Weber, S. Lambert and M. Wagner, in Freshwater Microplastics, Springer, Cham, 2018, pp. 153–180 Search PubMed.
- G. Kalčíková, B. Alič, T. Skalar, M. Bundschuh and A. Ž. Gotvajn, Wastewater treatment plant effluents as source of cosmetic polyethylene microbeads to freshwater, Chemosphere, 2017, 188, 25–31 CrossRef.
- F. Liu, N. B. Nord, K. Bester and J. Vollertsen, Microplastics Removal from Treated Wastewater by a Biofilter, Water, 2020, 12(4), 1085 CrossRef.
- Eurostat, Sewage sludge production and disposal, 2014 [cited 2019 June]; Available from: http://appsso.eurostat.ec.europa.eu/nui/show.do?dataset=env_ww_spd&lang=en.
- A. T. Jameel, S. A. Muyubi, M. I. A. Karim and M. Z. Alam, Removal of oil and grease as emerging pollutants of concern (EPC) in wastewater stream, IIUM Engineering Journal, 2011, 12(4), 161–169 Search PubMed.
-
D. H. Liu and B. G. Liptak, Environmental Engineers' Handbook Second Edition, CRC Press LLC, Florida, 1999 Search PubMed.
- X. Li, L. Chen, Q. Mei, B. Dong, X. Dai, G. Ding and E. Y. Zeng, Microplastics in sewage sludge from the wastewater treatment plants in China, Water Res., 2018, 142, 75–85 CrossRef CAS PubMed.
- F. Corradini, P. Meza, R. Eguiluz, F. Casado, E. Huerta-Lwanga and V. Geissen, Evidence of microplastic accumulation in agricultural soils from sewage sludge disposal, Sci. Total Environ., 2019, 671, 411–420 CrossRef CAS PubMed.
- A. M. Mahon, B. O'Connell, M. G. Healy, I. O'Connor, R. Officer, R. Nash and L. Morrison, Microplastics in Sewage Sludge: Effects of Treatment, Environ. Sci. Technol., 2017, 51(2), 810–818 CrossRef CAS PubMed.
-
J. Hammer, M. H. Kraak and J. R. Parsons, in Reviews of environmental contamination and toxicology, Springer, 2012, pp. 1–44 Search PubMed.
- C. M. Rochman, S. M. Kross, J. B. Armstrong, M. T. Bogan, E. S. Darling, S. J. Green, A. R. Smyth and D. Veríssimo, Scientific evidence supports a ban on microbeads, Environ. Sci. Technol., 2015, 49(18), 10759–10761 CrossRef CAS PubMed.
Footnote |
† Electronic supplementary information (ESI) available. See DOI: 10.1039/d0ew00397b |
|
This journal is © The Royal Society of Chemistry 2020 |
Click here to see how this site uses Cookies. View our privacy policy here.