Real-time monitoring of ciprofloxacin degradation in an electro-Fenton-like system using electrochemical-mass spectrometry†
Received
29th September 2019
, Accepted 12th November 2019
First published on 13th November 2019
Abstract
Electro-Fenton-like processes are widely used in removal of antibiotics in sewage owing to their excellent degradation efficiency for organics. However, the degradation mechanisms of antibiotics in electro-Fenton-like systems are still unclear caused by the non-real time detection of current methods. An electrochemical-mass spectrometry (EC-MS) method was developed for in situ analysis and real-time monitoring of electrochemical degradation of antibiotics in this study. In EC-MS, ITO glass was used as an electrochemical reactor and counter electrode, which is positioned in front of the MS inlet. A platinum slice as a working electrode was placed over the ITO glass. When different high voltages were applied on the electrodes, the electrochemical degradation of organics started and a charged spray was generated for MS detection. To enhance the electrochemical reaction efficiency, the voltage difference was optimized. As a proof-of-concept, the degradation of ciprofloxacin (CIP) in an electro-Fenton-like system was monitored, and five intermediates (m/z 330, 304, 348, 346 and 362) different from those obtained in other removal processes were detected and verified. Furthermore, six new characteristic intermediates (m/z 364, 305, 293, 277, 275 and 280) were captured. Meanwhile, the compounds were identified by high-resolution mass spectrometry and tandem mass spectrometry. Extracted ion chromatography (EIC) of several characteristic intermediates was performed for further discussion of the degradation pathway. Based on the data acquired, the degradation mechanism of CIP by an electro-Fenton-like process was proposed for the first time. This work provided a useful tool for mechanism research in electrochemical advanced oxidation processes.
Water impact
The concentrations of antibiotics, such as ciprofloxacin and norfloxacin, were up to ng L−1 or even μg L−1 levels in both influent and effluent of wastewater treatment plants (WWTPs). Advanced oxidation processes (AOPs), such as electro-Fenton and EF-like processes, were commonly used for the removal of antibiotics in WWTPs. However, the reported mechanisms of antibiotic removal in EF or EF-like systems are incomplete or unclear caused by the non-real time detection of current methods. It is significant to study the entire mechanism of pollutant degradation, which could improve degradation efficiency and prevent secondary pollution. Hence, we describe a simple but effective electrochemical-mass spectrometry method which could achieve in situ, real-time monitoring of the removal of contaminants in EF or EF-like systems. The device has a short dead time (in the order of subseconds), which greatly facilitates reaction monitoring. During monitoring of the degradation of ciprofloxacin in the EF-like process, five intermediates different from those in other removal processes were verified and six new characteristic intermediates were captured and identified which contribute to a feasible degradation mechanism.
|
Introduction
The increasing amount of antibiotics in sewage1–3 has drawn much attention in recent years. The concentrations of several drugs, such as ciprofloxacin and norfloxacin, were up to ng L−1 or even μg L−1 levels in both influent and effluent of wastewater treatment plants (WWTPs).4 Advanced oxidation processes (AOPs),5–7 such as electro-Fenton8–11 and electro-Fenton-like12–16 processes, were commonly used for the removal of antibiotics in WWTPs. Different from the electro-Fenton process, the SO4˙− radical generated in an electro-Fenton-like process has an excellent oxidizing ability and enhances the degradation efficiency of targets. Moreover, the use of an electric field decreases the dosage of Fe2+, and thus the iron-containing sludge,17 which largely increases the SO4˙− generation efficiency. In addition, the individual electrochemical could activate persulfate as well.18 These merits make electro-Fenton-like processes more and more popular in removal of organic pollutants from wastewater. It has been reported that electro-Fenton-like processes could provide a satisfactory efficient way for antibiotic removal.19
To study the degradation mechanisms of electro-Fenton or electro-Fenton-like processes, several methods have been developed.8,20–22 Liquid chromatography coupled with mass spectrometry (LC-MS) was the mainly used technique. MS could give the accurate molecular weight and structural information of analytes, which is commonly used in the mechanism study of chemical reactions. With the good separation ability of LC, LC-MS makes sure of the accuracy of intermediates and products detected.21,23,24 However, this method usually takes a dead time, in which some significant intermediates or products may undergo further degradation during the transmission process. Although this method could give the rough reaction mechanisms of organics in electro-Fenton and electro-Fenton-like processes, the non-real time (NRT) detection may miss the important intermediates and result in the incomplete mechanisms. Therefore, developing new methods to achieve the real-time monitoring of electro-Fenton-like processes is still desired.
Ambient ionization (AI) technologies could achieve chemical reactions and desorption/ionization of targets at the same time. Some of them are widely used for real-time monitoring of chemical reactions, such as direct analysis in real time (DART),25 desorption electrospray ionization (DESI),26 and electrospray ionization with a pressurized photoreactor.27 However, few of these technologies are suitable for the real-time monitoring of electrochemical reactions. Recently, we have developed electrochemical-mass spectrometry (EC-MS)28 based on droplet spray ionization (DSI).29 Two platinum electrodes were placed over a cover slip in front of the mass spectrometer. The voltage difference between the two platinum electrodes ensures the occurrence of electrochemical reactions. This method achieved the real-time monitoring of the electrochemical reaction of aniline28 and DMA,30 in which the radicals were successfully captured.
In this study, a new EC-MS system was developed by applying conductive indium tin oxide (ITO) glass as a liquid carrier rather than a cover slip. The ITO glass was used as an electrochemical reactor as well as a counter electrode. One platinum electrode as a working electrode (WE) was positioned over the ITO glass. The change of the electrode increased the working area, and thus enhanced the electrochemical reaction efficiency. In addition, the setup was further simplified. The performance of this new EC-MS system was evaluated by real-time monitoring the degradation process of ciprofloxacin (CIP) in an electro-Fenton-like system. The reaction intermediates were identified by high-resolution mass spectrometry and tandem mass spectrometry (MS/MS). Moreover, the degradation mechanism of CIP by the electro-Fenton-like process was proposed for the first time.
Experimental section
Chemicals and reagents
Methanol of MS grade was purchased from Sigma-Aldrich (Darmstadt, Germany). Ciprofloxacin and potassium persulfate with purities of more than 98% were purchased from J&K Scientific Co. (Beijing, China). Hydrated ferrous sulfate (FeSO4·7H2O) was purchased from J&K Scientific Co. (Beijing, China). The ciprofloxacin standard solution was prepared with methanol, while the potassium persulfate and ferrous sulfate solutions were prepared with ultrapure water (18.2 MΩ cm) obtained from a Milli-Q water purification system (Milford, MA). The reaction solutions of ciprofloxacin and potassium persulfate were prepared by diluting the standard solutions with methanol to 5 × 10−5 mol L−1 and 3 × 10−3 mol L−1, respectively. The reaction solution of ferrous sulfate was prepared by diluting the standard solution with water to 3 × 10−3 mol L−1. The ITO glass (20 × 20 × 0.15 mm) was purchased from Zono Science and Technology Ltd. (Hunan, China).
Mass spectrometry experiments
All the experiments were implemented on an LTQ/Orbitrap mass spectrometer (Thermo Fisher Scientific, Waltham, MA). The resolution was as high as 100
000. Full-scan spectra were obtained in positive ion mode with an m/z range from 100 to 500, and the maximum ion injection time was set at 500 ms. Mass spectra were recorded and analyzed using the Xcalibur® software (version 2.0.7, Thermo Fisher Scientific, US). For MS/MS experiments, the collision-induced dissociation (CID) voltage was adjusted to 18–25 V, and the maximum ion injection time was set at 100 ms. The tube lens voltage and capillary voltage were set at 110 V and 35 V, respectively. Other instrumental parameters were 1 microscan and 275 °C capillary temperature.
Electrochemical-mass spectrometry (EC-MS)
As the counter electrode (CE) and the substrate for loading the reaction solutions, the ITO glass is positioned in front of the MS inlet (Fig. 1). Meanwhile, a Pt plate (Fig. 1b, bottom 5 × 5 mm, side 5 × 10 mm) as the WE is placed over the corner of the ITO glass, and the distance between the Pt electrode and ITO glass is approximately 0.7 mm. Notably, only the bottom of the Pt electrode was immersed in the reaction solution during the reaction. The voltages are HV and HV + ΔV for the ITO glass and Pt electrode, respectively. The HV was set as high as 4800 V to induce the charged spray, and the ΔV was adjusted between 0 and 20 V. Before the detection, the CIP solution was loaded onto the corner of the ITO glass and the Pt electrode was submerged in the solution. After that, high voltages were applied to the electrodes to ionize analytes for MS detection, and the mass spectra were acquired. Then, potassium persulfate solution and ferrous sulfate solution were successively added into the CIP solution and the degradation of CIP by the electro-Fenton-like process started. Data were recorded continuously during the whole step. Notably, the high voltage applied was demonstrated to have no influence on the reaction system.28
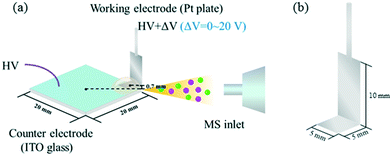 |
| Fig. 1 Schematic of the EC-MS system (a) and the size of the Pt plate (b). | |
Results and discussion
Voltage difference optimization
To evaluate the stability of EC-MS, the total ion chromatogram (TIC) of the mixture of CIP, Fe2+ and S2O82− was acquired and is given in Fig. 2a, illustrating a steady ion current for reaction monitoring. Prior to the in situ and real-time monitoring, the voltage difference (ΔV) between the ITO glass and Pt electrode was optimized from 0 V to 20 V (Fig. 2b). Notably, the addition of Fe2+ and S2O82− could lead to ion suppression of CIP in the beginning, which contributes to an uncontrollable intensity reduction of CIP. Therefore, the intensity of the main degradation product (m/z 263.0825) after 2 min reaction was used to investigate the CIP degradation efficiency with the increase of the voltage difference. When the voltage difference was set at 0 V, the signal of m/z 263.0825 at a reaction time of 2 min was absent because of the low degradation rate of CIP. A higher potential leads to a greater electron density, and thus a higher electronic transmission efficiency. As a result, the reaction rate accelerated and thus the signal intensity of m/z 263.0825 increased with increasing voltage difference. Therefore, 20 V was used as the voltage difference between the ITO glass and Pt electrode in the following experiments.
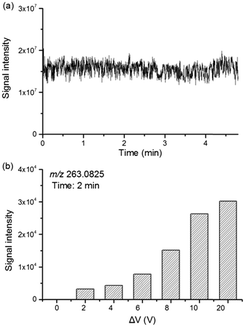 |
| Fig. 2 (a) TIC of the mixture of CIP, Fe2+ and S2O82− and (b) effect of the voltage difference on the intensity of the main product (m/z 263.0825). The signal intensities were obtained at a reaction time of 2 min. The concentrations of CIP, Fe2+ and S2O82− were 5 × 10−5 mol L−1, 3 × 10−3 mol L−1 and 3 × 10−3 mol L−1, respectively. The total volume of the reaction solution was 100 μL, and the proportion of methanol/water was 9 : 1. The HV was 4800 V. | |
Merit of EC-MS
The merit of EC-MS was evaluated by monitoring the degradation of CIP in the electro-Fenton-like system. In detail, the experiments were conducted under methanol and water (9
:
1) mixture conditions. Notably, excess Fe2+ contributes to the removal of SO4˙− (ref. 31) and ˙OH,32 which would finally influence the degradation efficiency. Therefore, the proportion of Fe2+/S2O82− was chosen to be 1
:
2 to decrease the dosage of Fe2+.
The real-time mass spectra were obtained during the electrochemical degradation reaction process (Fig. 3). In Fig. 3a, the spectrum was dominated by the protonated CIP (m/z 332). However, the basic peak shifted to m/z 291 after 5 min reaction, which is assumed to be one of the intermediates in pathway I (Fig. 6). Intermediates reported in other AOP methods based on electro-Fenton and persulfate systems, such as m/z 279,21 334, 306, 263 and 291 (ref. 33), were detected in the electro-Fenton-like system (Fig. 3b). Aside from high-resolution mass spectrometry, MS/MS was used for verifying the structural formula of these intermediates (Fig. S1†). The same intermediates illustrated that there are several similarities in degradation of CIP even by different methods, and the inferred mechanism in this study contributes significantly to the degradation of CIP by other methods.
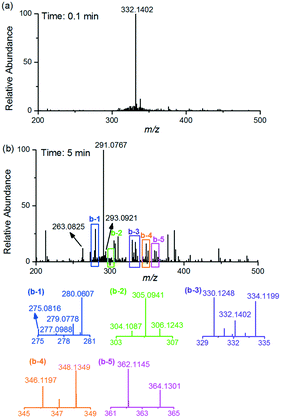 |
| Fig. 3 Mass spectra of CIP degradation in the electro-Fenton-like system using EC-MS at different times: (a) 0.1 min and (b) 5 min. The concentrations of CIP, Fe2+ and S2O82− were 5 × 10−5 mol L−1, 3 × 10−3 mol L−1 and 3 × 10−3 mol L−1, respectively. The total volume of the reaction solution was 100 μL, and the proportion of methanol/water was 9 : 1. The HV and ΔV were 4800 V and 20 V, respectively. All the deviations of mass-to-charge ratios range from −3.7857 to 2.9650 ppm. | |
Furthermore, five intermediates (m/z 330, 304, 348, 346 and 362), which have been reported in an Fe(II)–persulfate system or other AOP methods, were found to be different structures in the electro-Fenton-like process based on the high-resolution mass spectra and tandem mass spectra. Notably, the exact mass-to-charge ratio of 330.1248 demonstrated that the intermediate with m/z 330 was generated by elimination reaction, which was different from that generated by substituting fluorine with hydroxyl reported in other systems such as Fe(II)–persulfate33 and MPUV/PMS systems.34 The exact mass-to-charge ratio of 304.1087 indicated the existence of fluorine in m/z 304. To further verify the chemical construction of m/z 330 and 304, MS/MS experiments were conducted, and the mass spectra are shown in Fig. S2.† The structure of m/z 348 was demonstrated by the MS/MS experiment (Fig. 4a) as well, and the fragment peak with m/z 316 might be due to the loss of CH3OH. Moreover, MS/MS CID of m/z 346 lead to a 32 Da loss (Fig. 4b). These results indicated the potential existence of –CHOH in m/z 348 and 346, which were different from the construction obtained in the Fe(II)–persulfate system.33 The proposed structure of m/z 362 was supported by the MS/MS mass spectrum (Fig. 4c). Note that two of the fragment ions (m/z 330 and 300) indicated the potential existence of –CH2CH2NH2. In addition, six characteristic intermediates (m/z 364, 305, 293, 277, 275 and 280) were newly found by real-time monitoring using EC-MS. The exact mass-to-charge ratios with deviations at a range of −3.7857–2.9650 ppm are given in Fig. 6, and the MS/MS mass spectra are shown in Fig. S3† to deduce the structures of these six intermediates.
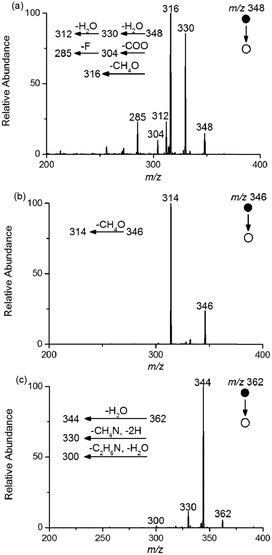 |
| Fig. 4 MS/MS mass spectra of (a) m/z 348, (b) 346 and (c) 362. | |
The intensity change of CIP and some key intermediates during the degradation process is shown in Fig. 5. The intensities of intermediates increased as the intensity of m/z 332 decreased. The m/z 330 was promptly generated at the beginning of the reaction, which illustrates that m/z 330 is the first intermediate of the removal of CIP in the electro-Fenton-like process. Afterwards, m/z 346 appeared at 0.25 min, and m/z 348 appeared at 0.5 min. The appearance order of m/z 346 and 348 indicates that m/z 348 was not the only precursor of m/z 346, which was different from the removal of CIP in the Fe(II)–persulfate system.33 Other key intermediates appeared at 0.75 min (m/z 334, 291 and 263), 1 min (m/z 279) and 1.25 min (m/z 280), respectively. The extracted ion currents (EICs) are shown in Fig. S4.† The intensities of CIP and these intermediates detected were reduced in the later reaction process, which indicates that CIP was finally degraded into short chain carboxylic acids.35
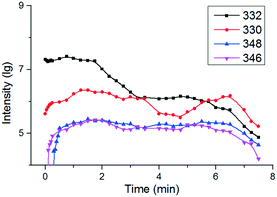 |
| Fig. 5 EIC of m/z 332, 330, 348 and 346. The concentrations of CIP, Fe2+ and S2O82− were 5 × 10−5 mol L−1, 3 × 10−3 mol L−1 and 3 × 10−3 mol L−1, respectively. The total volume of the reaction solution was 100 μL, and the proportion of methanol/water was 9 : 1. The ΔV was 20 V. | |
Mechanism of CIP removal by the electro-Fenton-like process
According to the analysis of the spectra, the degradation process of CIP is likely to occur via three major pathways (I–III) (Fig. 6). Different from the electro-Fenton process in which the hydroxyl radicals play an important role,36,37 the substitution of H2O2 by S2O82− generates large amounts of SO4˙− (ref. 38) in the reaction system. It was reported that there are both SO4˙− and ˙OH in the system used,39 and the sulfate radical is predominant at acidic pH (ref. 40–42) (the initial pH = 3.73 for the reaction system in this work). The potential mechanism of the main free radical attack is also given in the ESI† (Fig. S5).
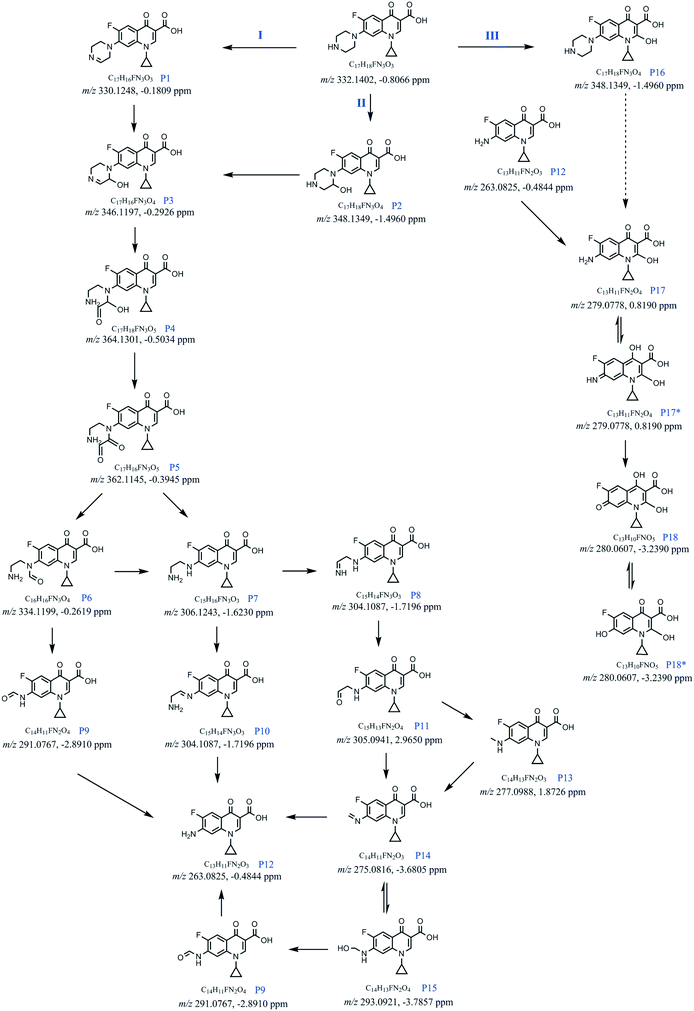 |
| Fig. 6 Proposed pathways for CIP degradation in the electro-Fenton-like system. The dotted line indicates that there might be intermediates undiscovered. All the deviations of mass-to-charge ratios range from −3.7857 to 2.9650 ppm. | |
All three pathways are initiated by the multiple attacks of free radicals to the heterocyclic ring. In pathway I, CIP is firstly oxidized to P1 (m/z 330.1248), which is then oxidatively hydroxylated to P3 (m/z 346.1197). Meanwhile, CIP can be oxidized and hydroxylated to P2 (m/z 348.1349), which can also be transformed into P3 by oxidative dehydrogenation. After that, hydrolysis of –C
N– of P3 leads to generation of P4 (m/z 364.1301), which can be further oxidized to P5 (m/z 362.1145). Subsequently, radical hydrogen atom abstraction (HAA) and radical decarbonylation of P5 finally generate P6 (m/z 334.1199) and P7 (m/z 306.1243). Moreover, both P6 and P7 can be further degraded to P12 (m/z 263.0825). Notably, P7 can generate P8 (m/z 304.1087) via further oxidation. Hydrolysis of P8 can generate P11 (m/z 305.0941). Nevertheless, radical decarbonylation affords radical intermediates. Further oxidative dehydrogenation and hydrogen atom transfer produce P14 and P13, respectively. P13 can be further oxidized to P14. P14 undergoes nucleophilic addition by H2O to afford P15. P15 can contribute to the generation of P9 through the oxidation, which can undergo radical decarbonylation to generate P12. P12 can also be generated via direct hydrolysis of P13.
In pathway III, oxidative hydroxylation of the alkenyl C–H bond produces P16 (m/z 348.1349), in which the piperazine ring is subsequently oxidized and cleaved to afford P17. Tautomerism of P17 affords P17* (m/z 279.0778), which can be hydrolyzed to P18 and/or P18*. Notably, the P12 generated in pathway I can also produce P17 and/or P17* by substitution reaction. The further degradation at the aromatic ring contributes to the formation of P18 and/or P18*.
Conclusions
In this study, a simple and effective EC-MS method was developed for in situ and real-time monitoring of electrochemical degradation of antibiotics. It was achieved by placing a platinum slice over conductive ITO glass which was positioned in front of the MS inlet. Different high voltages were applied on two electrodes to simultaneously generate the electrochemical reaction and the charged spray for MS detection. As a proof-of-concept, the removal of CIP in the electro-Fenton-like system was monitored and the transformation products were identified by high-resolution mass spectrometry and MS/MS spectrometry. Five intermediates (m/z 330, 304, 348, 346 and 362) different from those in other removal processes were detected and verified. Furthermore, six new characteristic intermediates (m/z 364, 305, 293, 277, 275 and 280) were captured and identified. Meanwhile, a feasible degradation mechanism of CIP removal by the electro-Fenton-like process was proposed for the first time. These results indicate that EC-MS has advantages of ease of setup, high sensitivity and high reaction rates. It provided a potential tool for in situ and real-time monitoring of organics degradation by electrochemical advanced oxidation processes, which is important for mechanism research and the improvement of electrochemical advanced oxidation processes.
Conflicts of interest
There are no conflicts to declare.
Acknowledgements
This work was supported by the National Key R&D Program of China (No. 2016YFF0103801), the National Natural Science Foundation of China (No. 21705030 and No. 21804027), the Shandong Provincial Key R&D Program (No. 2016YYSP013) and the Shandong Provincial Natural Science Foundation, China (Grant No. ZR2018PB016).
Notes and references
- N. Unceta, M. Carmen Sampedro, N. K. Abu Bakar, A. Gomez-Caballero, M. Aranzazu Goicolea and R. J. Barrio, Multi-residue analysis of pharmaceutical compounds in wastewaters by dual solid-phase microextraction coupled to liquid chromatography electrospray ionization ion trap mass spectrometry, J. Chromatogr. A, 2010, 1217, 3392–3399 CrossRef CAS PubMed.
- G. McEneff, L. Barron, B. Kelleher, B. Paull and B. Quinn, A year-long study of the spatial occurrence and relative distribution of pharmaceutical residues in sewage effluent, receiving marine waters and marine bivalves, Sci. Total Environ., 2014, 476, 317–326 CrossRef PubMed.
- L. G. Chaves-Barquero, K. H. Luong, C. J. Mundy, C. W. Knapp, M. L. Hanson and C. S. Wong, The release of wastewater contaminants in the Arctic: A case study from Cambridge Bay, Nunavut, Canada, Environ. Pollut., 2016, 218, 542–550 CrossRef CAS PubMed.
- W. Ben, B. Zhu, X. Yuan, Y. Zhang, M. Yang and Z. Qiang, Occurrence, removal and risk of organic micropollutants in wastewater treatment plants across China: Comparison of wastewater treatment processes, Water Res., 2018, 130, 38–46 CrossRef CAS PubMed.
- T. An, H. Yang, G. Li, W. Song, W. J. Cooper and X. Nie, Kinetics and mechanism of advanced oxidation processes (AOPs) in degradation of ciprofloxacin in water, Appl. Catal., B, 2010, 94, 288–294 CrossRef CAS.
- H. Ou, J. Ye, S. Ma, C. Wei, N. Gao and J. He, Degradation of ciprofloxacin by UV and UV/H2O2 via multiple-wavelength ultraviolet light-emitting diodes: Effectiveness, intermediates and antibacterial activity, Chem. Eng. J., 2016, 289, 391–401 CrossRef CAS.
- H. Pourzamani, N. Mengelizadeh, H. Mohammadi, N. Niknam, B. Neamati and R. Rahimi, Comparison of electrochemical advanced oxidation processes for removal of ciprofloxacin from aqueous solutions, Desalin. Water Treat., 2018, 113, 307–318 CrossRef CAS.
- A. Ozcan, A. A. Ozcan and Y. Demirci, Evaluation of mineralization kinetics and pathway of norfloxacin removal from water by electro-Fenton treatment, Chem. Eng. J., 2016, 304, 518–526 CrossRef CAS.
- S. Ahmadzadeh and M. Dolatabadi, Removal of acetaminophen from hospital wastewater using electro-Fenton process, Environ. Earth Sci., 2018, 77, 53 CrossRef.
- G. Divyapriya, I. Nambi and J. Senthilnathan, Ferrocene functionalized graphene based electrode for the electro-Fenton oxidation of ciprofloxacin, Chemosphere, 2018, 209, 113–123 CrossRef CAS PubMed.
- G. Divyapriya, R. Srinivasan, I. M. Nambi and J. Senthilnathan, Highly active and stable ferrocene functionalized graphene encapsulated carbon felt array - A novel rotating disc electrode for electro-Fenton oxidation of pharmaceutical compounds, Electrochim. Acta, 2018, 283, 858–870 CrossRef CAS.
- B. Balci, M. A. Oturan, N. Oturan and I. Sires, Decontamination of Aqueous Glyphosate, (Aminomethyl) phosphonic Acid, and Glufosinate Solutions by Electro-Fenton-like Process with Mn2+ as the Catalyst, J. Agric. Food Chem., 2009, 57, 4888–4894 CrossRef CAS PubMed.
- N. Oturan, M. Zhou and M. A. Oturan, Metomyl Degradation by Electro-Fenton and Electro-Fenton-Like Processes: A Kinetics Study of the Effect of the Nature and Concentration of Some Transition Metal Ions As Catalyst, J. Phys. Chem. A, 2010, 114, 10605–10611 CrossRef CAS PubMed.
- E. Alfaya, O. Iglesias, M. Pazos and M. Angeles Sanroman, Environmental application of an industrial waste as catalyst for the electro-Fenton-like treatment of organic pollutants, RSC Adv., 2015, 5, 14416–14424 RSC.
- A. Long and H. Zhang, Selective oxidative degradation of toluene for the recovery of surfactant by an electro/Fe2+/persulfate process, Environ. Sci. Pollut. Res., 2015, 22, 11606–11616 CrossRef CAS PubMed.
- B. Kamarehie, J. Mohamadian, S. A. Mousavi, G. Asgari and Y. Dadban Shahamat, Aniline degradation from aqueous solution using electro/Fe2+/peroxydisulfate process, Desalin. Water Treat., 2017, 80, 337–343 CrossRef CAS.
- Y. R. Wang and W. Chu, Degradation of 2,4,5-trichlorophenoxyacetic acid by a novel Electro-Fe(II)/Oxone process
using iron sheet as the sacrificial anode, Water Res., 2011, 45, 3883–3889 CrossRef CAS PubMed.
- W.-S. Chen and C.-P. Huang, Mineralization of aniline in aqueous solution by electrochemical activation of persulfate, Chemosphere, 2015, 125, 175–181 CrossRef CAS PubMed.
- Y. Sun, Y. Li, X. Mi, S. Zhan and W. Hu, Evaluation of ciprofloxacin destruction between ordered mesoporous and bulk NiMn2O4/CF cathode: efficient mineralization in a heterogeneous electro-Fenton-like process, Environ. Sci.: Nano, 2019, 6, 661–671 RSC.
- M. A. Oturan, J. Peiroten, P. Chartrin and A. J. Acher, Complete destruction of p-nitrophenol in aqueous medium by electro-Fenton method, Environ. Sci. Technol., 2000, 34, 3474–3479 CrossRef CAS.
- M. S. Yahya, N. Oturan, K. El Kacemi, M. El Karbane, C. T. Aravindakumar and M. A. Oturan, Oxidative degradation study on antimicrobial agent ciprofloxacin by electro-fenton process: Kinetics and oxidation products, Chemosphere, 2014, 117, 447–454 CrossRef CAS PubMed.
- T. Shirafuji, Y. Ishida, A. Nomura, Y. Hayashi and M. Goto, Reaction mechanisms of methylene-blue degradation in three-dimensionally integrated micro-solution plasma, Jpn. J. Appl. Phys., 2017, 56, 06HF02 CrossRef.
- Y. Zhang, Z. Shen, X. Zhou, M. Zhang and F. Jin, Solvent isotope effect and mechanism for the production of hydrogen and lactic acid from glycerol under hydrothermal alkaline conditions, Green Chem., 2012, 14, 3285–3288 RSC.
- S. Hisaindee, M. A. Meetani and M. A. Rauf, Application of LC-MS to the analysis of advanced oxidation process (AOP) degradation of dye products and reaction mechanisms, TrAC, Trends Anal. Chem., 2013, 49, 31–44 CrossRef CAS.
- C. Petucci, J. Diffendal, D. Kaufman, B. Mekonnen, G. Terefenko and B. Musselman, Direct analysis in real time for reaction monitoring in drug discovery, Anal. Chem., 2007, 79, 5064–5070 CrossRef CAS PubMed.
- M. Girod, E. Moyano, D. I. Campbell and R. G. Cooks, Accelerated bimolecular reactions in microdroplets studied by desorption electrospray ionization mass spectrometry, Chem. Sci., 2011, 2, 501–510 RSC.
- H. Zhang, X. Li, K. Yu, N. Li, J. He, H. You and J. Jiang, On-line monitoring of photolysis reactions using electrospray ionization mass spectrometry coupled with pressurized photoreactor, Anal. Chim. Acta, 2018, 1013, 36–42 CrossRef CAS PubMed.
- K. Yu, H. Zhang, J. He, R. N. Zare, Y. Wang, L. Li, N. Li, D. Zhang and J. Jiang, In Situ Mass Spectrometric Screening and Studying of the Fleeting Chain Propagation of Aniline, Anal. Chem., 2018, 90, 7154–7157 CrossRef CAS PubMed.
- J. Jiang, H. Zhang, M. Li, M. T. Dulay, A. J. Ingram, N. Li, H. You and R. N. Zare, Droplet Spray Ionization from a Glass Microscope Slide: Real-Time Monitoring of Ethylene Polymerization, Anal. Chem., 2015, 87, 8057–8062 CrossRef CAS PubMed.
- H. Zhang, K. Yu, N. Li, J. He, L. Qiao, M. Li, Y. Wang, D. Zhang, J. Jiang and R. N. Zare, Real-time mass-spectrometric screening of droplet-scale electrochemical reactions, Analyst, 2018, 143, 4247–4250 RSC.
- R. Li, J. Kong, H. Liu, P. Chen, G. Liu, F. Li and W. Lv, A sulfate radical based ferrous–peroxydisulfate oxidative system for indomethacin degradation in aqueous solutions, RSC Adv., 2017, 7, 22802–22809 RSC.
- M. S. Yahya, N. Beqqal, A. Guessous, M. R. Arhoutane and K. El Kacemi, Degradation and mineralization of moxifloxacin antibiotic in aqueous medium by electro-Fenton process: Kinetic assessment and oxidation products, Cogent Chem., 2017, 3, 1290021 CrossRef.
- Y. Ji, C. Ferronato, A. Salvador, X. Yang and J.-M. Chovelon, Degradation of ciprofloxacin and sulfamethoxazole by ferrous-activated persulfate: Implications for remediation of groundwater contaminated by antibiotics, Sci. Total Environ., 2014, 472, 800–808 CrossRef CAS PubMed.
- X. Ao, W. Liu, W. Sun, M. Cai, Z. Ye, C. Yang, Z. Lu and C. Li, Medium pressure UV-activated peroxymonosulfate for ciprofloxacin degradation: Kinetics, mechanism, and genotoxicity, Chem. Eng. J., 2018, 345, 87–97 CrossRef CAS.
- A. Dirany, I. Sires, N. Oturan, A. Ozcan and M. A. Oturan, Electrochemical treatment of the antibiotic sulfachloropyridazine: kinetics, reaction pathways, and toxicity evolution, Environ. Sci. Technol., 2012, 46, 4074–4082 CrossRef CAS PubMed.
- X. Liu, D. Yang, Y. Zhou, J. Zhang, L. Luo, S. Meng, S. Chen, M. Tan, Z. Li and L. Tang, Electrocatalytic properties of N-doped graphite felt in electro-Fenton process and degradation mechanism of levofloxacin, Chemosphere, 2017, 182, 306–315 CrossRef CAS PubMed.
- Y. Zhou, X. Liu, Y. Zhao, S. Luo, L. Wang, Y. Yang, M. A. Oturan and Y. Mu, Structure-based synergistic mechanism for the degradation of typical antibiotics in electro-Fenton process using Pd-Fe3O4 model catalyst: Theoretical and experimental study, J. Catal., 2018, 365, 184–194 CrossRef CAS.
- H. Zhou, S. Wu, Y. Zhou, Y. Yang, J. Zhang, L. Luo, X. Duan, S. Wang, L. Wang and D. C. W. Tsang, Insights into the oxidation of organic contaminants by iron nanoparticles encapsulated within boron and nitrogen co-doped carbon nanoshell: Catalyzed Fenton-like reaction at natural pH, Environ. Int., 2019, 128, 77–88 CrossRef CAS PubMed.
- C. Liu, B. Wu and X. E. Chen, Sulfate radical-based oxidation for sludge treatment: A review, Chem. Eng. J., 2018, 335, 865–875 CrossRef CAS.
- C. Liang, Z.-S. Wang and C. J. Bruell, Influence of pH on persulfate oxidation of TCE at ambient temperatures, Chemosphere, 2007, 66, 106–113 CrossRef CAS PubMed.
- D. Ouyang, J. Yan, L. Qian, Y. Chen, L. Han, A. Su, W. Zhang, H. Ni and M. Chen, Degradation of 1,4-dioxane by biochar supported nano magnetite particles activating persulfate, Chemosphere, 2017, 184, 609–617 CrossRef CAS PubMed.
- J. Ma, Y. Ding, L. Chi, X. Yang, Y. Zhong, Z. Wang and Q. Shi, Degradation of benzotriazole by sulfate radical-based advanced oxidation process, Environ. Technol., 2019 DOI:10.1080/09593330.2019.1625959.
Footnote |
† Electronic supplementary information (ESI) available: MSn mass spectra of intermediates, signal intensities of intermediates obtained at different reaction times and potential mechanism of free radical attack for CIP degradation in an EF-like system. See DOI: 10.1039/c9ew00868c |
|
This journal is © The Royal Society of Chemistry 2020 |