Alleviation of nitrogen stress in rice (Oryza sativa) by ceria nanoparticles†
Received
17th July 2020
, Accepted 29th August 2020
First published on 31st August 2020
Abstract
This study explored the potential of ceria nanoparticles (CeO2 NPs) to alleviate stress in hydroponic rice caused by low N (LN) and high N (HN) stresses. The N content in plants was measured after 3 weeks of treatment with CeO2 NPs. The impact of CeO2 NPs on plants under medium N (MN, a normal condition) was studied as a comparison. LN resulted in N deficiency while HN led to N excess in plants while impairing plant growth. CeO2 NPs (100 and 500 mg L−1) increased the N levels in roots and shoots under LN by 6–12% and 22–30%, respectively. However, under HN stress, 500 mg L−1 CeO2 NPs reduced the N levels in roots and shoots by 9% and 6%, respectively. CeO2 NP treatment enhanced the activities of key enzymes involved in N assimilation, glutamine synthetase (GS), glutamine oxoglutarate aminotransferase (GOGAT) and glutamate dehydrogenase (GDH), accounting for the increased N content in plants under LN. Under HN, 500 mg L−1 CeO2 NPs downregulated the GS and GDH activity by 42% and 36%, respectively. CeO2 NPs reduced oxidative membrane and DNA damage and enhanced plant tolerance to N-stress by regulating the antioxidant enzyme system and the levels of proline and phytohormones including gibberellin 3, abscisic acid, zeatin riboside and indole-3-acetic acid. However, when N is supplied normally, CeO2 NPs caused oxidative stress in plants thereby impairing plant growth. A change of N conditions altered the root exudate composition and led to different extents of transformation of CeO2 NPs at the root interface and different Ce uptake and translocation in plants. This study for the first time reported that CeO2 NPs could act as an alleviator of N stress in rice, while it might be a risk when the N supply is normal.
Environmental significance
Nitrogen (N) stress caused by N deficiency or N excess widely exists in agriculture, and can cause plant stress and growth impairment. This study for the first time reported that CeO2 nanoparticles can improve rice growth under both N deficiency and N excess conditions by enhancing nitrogen assimilation and use efficiency in plants, alleviating oxidative stress via regulating the antioxidant system, and enhancing plant tolerance to N stress by regulating the proline and phytohormone levels. However, when N was supplied normally, CeO2 nanoparticles caused oxidative damage and reduced plant tolerance thus impairing plant growth.
|
Introduction
Nitrogen (N) is an essential element for plant growth. However, N stress in plants can be caused by both N deficiency and N excess.1,2 Since N is a major component of amino acids, proteins, enzymes, and nucleic acids as well as photosynthetic pigments, the lack of N can severely impair plant growth, e.g., photosynthesis.3 N deficiency also causes reduced flowering,4 fruiting5 or carbohydrate content,1 and thus a lower nutritional value of the resulting crops. Application of N fertilizers such as ammonium nitrate and urea is used to improve the N-deficient conditions; however, excessive use of N fertilisers also cause N stress to plants.2 Excessive N may enlarge the space between xylem cells thereby reducing the strength of stems and causing lodging (bending near the stem base) of crops which makes them difficult to harvest, reducing yields.6 Moreover, the use efficiency of N by plants is very poor and currently stands at only 45% globally of the total soil N content,7 with the rest being lost into water causing water pollution (e.g., eutrophication) or air causing greenhouse effects (N2O) and air quality deterioration (ammonia volatilization).
Nanotechnology offers great potential to tailor fertilizer delivery and improve the nutrient use efficiency (NUE) by plants, thereby reducing their environmental release and subsequent environmental consequences.8,9 A recent study showed that incorporating urea into hydroxyapatite nanostructures can regulate the slow release of urea, which led to a better yield at a 50% lower urea application rate.10 Many studies have shown that nanomaterials may also enhance the tolerance of plants to external stresses such as drought, flooding, cold, heat, salinity and heavy metal stress.11 For example, TiO2 NPs have been reported to improve plant growth under drought12 or cold13 stress. CuO NPs as low as 1 mg L−1 can enhance the tolerance to pathogens of tomato grown in soil infested with Fusarium oxysporum.14 CeO2 NPs also showed potential to improve plant growth under stress conditions, such as excess light, heat, dark chilling15 and high salinity,16 which have been reported recently. CeO2 NPs (500 mg kg−1 dry sand) can shorten the root apoplastic barrier which allowed more Na+ flux to shoots which led to better physiological performance of rapeseed grown in sand.16 CeO2 NPs are also able to scavenge reactive oxygen species (ROS) and enhance photosynthesis in Arabidopsis thaliana.15 Such redox functioning of CeO2 NPs is mainly derived from the oxygen defects in the lattice.17 CeO2 NPs have two oxidation states (+3, +4) which alternate during redox reactions; large amounts of surface oxygen vacancies are generated via oxygen loss or electron transfer during these redox reactions. Therefore, the ROS scavenging activity is highly dependent on the amount of surface Ce3+. Compared with bulk CeO2, nano-sized CeO2 has a larger fraction of surface Ce3+ and oxygen vacancies thereby having stronger ROS scavenging activity. Due to this feature, CeO2 NPs have attracted considerable research interest and have been widely used, e.g., as additives in cosmetics, diesel and tyres.18 The potential of CeO2 NPs in agricultural application has only been explored recently and understanding is still in its infancy. In addition, negative effects of CeO2 NPs on plant growth have been also reported. As noted by Lowry et al.,8 many challenges and barriers need to be overcome before the real potential of nanomaterials (NMs) in agriculture can be achieved. A major barrier is that the interaction of NMs with plants, and the effects of these interactions on the behaviour of NMs, such as the transformations in the soil and at the soil/root interface and the uptake in plants,19 as well as on the plant growth, are deeply interrelated and their exploration have only begun recently. Additionally, the environmental safety and human safety of NMs and their residues in plants and soil lack sufficient understanding currently.
The present study aims to explore the potential of CeO2 NPs to alleviate stress caused by N deficiency or N excess and to improve the NUE, and to investigate the impacts (implications) of CeO2 NPs on plant growth under normal conditions. Plant growth with CeO2 NP treatment under the two types of stress as well as normal conditions was evaluated by determining the plant phenotype (biomass, seedling length), the regulation of the antioxidant systems, and the occurrence of DNA damage. N assimilation and metabolism, and the enzymes involved in the processes, were measured. Ce uptake and accumulation in rice plants, and the influence of the N conditions on this, were also determined. This study provides significant insights into the physiological impacts of CeO2 NPs on plant growth as well as a potential way to improve N use efficiency by plants under N stress conditions.
Materials and methods
Chemicals, CeO2 NPs and characterization
CeO2 NPs were purchased from Pantian Powder Materials (Shanghai, China). The morphology and size of the CeO2 NPs were characterized by transmission electron microscopy (TEM, JEOL, Japan) (Fig. S1†). Raman spectra were recorded using a LabRAM HR Evolution Raman spectrometer (Horiba Scientific, Japan) (Fig. S2†). The zeta potential and hydrodynamic sizes of CeO2 NPs (10 mg L−1) in deionized water and nutrient solution were analysed with a Zetasizer Nano ZS90 (Malvern, UK) (Table S1†). Other chemicals were purchased from Sigma Aldrich.
Plant growth and treatments
Rice seeds (Y900) were purchased from the Chinese Academy of Agricultural Sciences. Seeds with a uniform size were selected and sterilized in 5% H2O2 for 30 min. Seeds were germinated in petri dishes with moist filter paper in the dark at 25 °C. After 7 days, uniform seedlings were selected, and each seedling was anchored to plastic foam and transferred into a 250 mL beaker containing 100 mL of Kimura B nutrient solution.20 Eight replicates were set for each treatment. The seedlings were allowed to grow in a growth chamber (PRX-450C, Saifu, China) with a day/night temperature of 28 °C/25 °C, day/night humidity of 50%/70% and 16 h photoperiod (light intensity of 1.76 × 104 lux) for 10 days before treatment.
The seedlings were then treated with 100 mg L−1 and 500 mg L−1 CeO2 NPs under three different N conditions, i.e. low nitrogen (LN), medium nitrogen (MN) and high nitrogen (HN). The N concentrations were 0.72 mM, 2.86 mM and 7.15 mM in LN, MN and HN solutions, respectively. The MN solution represents the Kimura solution with normal N supplement, while the LN and HN conditions will cause N stress. The pH of the solutions was adjusted to 5.5. CeO2 NPs were added into the solutions to a concentration of 100 mg L−1 or 500 mg L−1 followed by sonicating pre-treatment in a water bath for 20 min. The seedlings were then treated with the CeO2 NP suspensions. To simulate a single dose of exposure, no further treatments were applied. The suspensions were replenished every other day with the nutrient solutions for compensation of transpired water. The plants were harvested after treatment for 3 weeks. The seedling length and fresh weight were measured.
Determination of C and N content in plants
Fresh seedlings were dried at 105 °C for 30 min and at 75 °C overnight. Dry weights of shoots and roots were measured followed by grinding into powders. The dry powders were then used to measure the total C and N content using a C/N analyser (Vario ELIII, Elementar, Germany).
Stress responses of rice to CeO2 NP treatments
The superoxide dismutase (SOD) and peroxidase (POD) activities, and malondialdehyde (MDA) and proline content were measured to evaluate the stress responses of rice to the CeO2 NP treatments. SOD, POD and MDA were measured using assay kits purchased from Nanjing Jiancheng Bioengineering Institute (Nanjing, China). Proline was measured following a previously described method.21 Briefly, fresh samples were homogenized with cooling using an ice bath and diluted with 3% sulfosalicylic acid to 10 mL followed by centrifugation at 1500g for 10 min. The supernatant was mixed with acidic ninhydrin and glacial acetic acid and reacted in boiling water for 1 h followed by addition of methylbenzene. The absorbance of the extractives was measured at 520 nm and the concentration was calculated based on a calibration curve. To ensure accuracy and linearity, standard SOD, POD, MDA and proline with known concentrations (6 concentrations) were prepared and analyzed following the same procedure described in the kits for sample analysis. Six replicate samples were tested for each data point and the experiments were repeated three times.
Determination of DNA damage
The DNA damage was evaluated by measuring the content of 8-hydroxy-2 deoxyguanosine (8-OHdG) in the plants. Fresh samples were ground in liquid nitrogen followed by homogenization in a buffer solution containing 2% CTAB, 100 mM Tris-HCl, 2% polyvinylpyrrolidone (PVP), 1.5 mM NaCl, 0.2 mM EDTA and 1% mercaptoethanol. DNA was extracted using a DNA kit (Qiagen, France), and 8-OHdG was quantified using a DNA damage ELISA kit (Stress Marq Biosciences, Inc, Thermo, USA). Six replicate samples were tested for each data point and the experiments were repeated three times. The 8-OHdG solutions (0–60 ng ml−1) were analysed as standards following the same procedure described in the assay kit.
Enzyme activity involved in N assimilation and metabolism
The activities of glutamine synthetase (GS), glutamine oxoglutarate aminotransferase (GOGAT) and glutamate dehydrogenase (GDH) were measured following previously described methods. Briefly, for GS, fresh samples were ground in liquid nitrogen and extracted in cold buffer solution consisting of hydroxylamine hydrochloride (100 mM, pH 7.4), 1 mM MgCl2, 1 mM EDTA, and 10 mM 2-mercaptoethanol. The homogenate was incubated at 37 °C for 15 min followed by addition of FeCl3 solution (0.37 mM FeCl3, 0.2 M trichloroacetic acid and 0.6 mM HCl). Samples were centrifuged at 4000g for 10 min. The absorbance of the supernatants at 540 nm was measured.
For GOGAT, samples were firstly homogenized in a buffer solution containing 0.2 M Na3PO4, 2 mM EDTA, 50 mM KCl, 1% 2-mercaptoethanol and 0.5% Triton X-100. The homogenate was filtered and centrifuged at 2000g for 10 min. (NH4)2SO4 solids were added into the supernatant to allow precipitation, and the precipitates were dissolved in the aforementioned buffer followed by passing through a Sephadex G-75 column to obtain the enzyme solution. A solution consisting of 10 mM α-ketoglutaric acid, 1 mM KCl, 37.5 mM Tris-HCl (pH 7.6), 0.6 mM NADH and 8 mM L-glutamine was added into the enzyme solution. Absorbance at 340 nm was recorded.
To determine GDH, fresh samples were homogenized in deionized water. The samples were then added into a solution containing 0.1 M Tris-HCl (pH 8.3), 1.5 M NH4Cl, 0.225 M α-ketoglutaric acid and 7.5 mM NADH at 30 °C and allowed to react for 2 min. The absorbance at 340 nm was recorded.
All the tests were repeated three times with six replicate samples analysed. GS, GOGAT and GDH with known concentrations were analysed as standards following the same procedure described above.
Phytohormone levels in plants
The levels of phytohormones including gibberellin 3 (GA3), abscisic acid (ABA), zeatin riboside (ZR) and indole-3-acetic acid (IAA) in extracted and purified shoots and roots were determined following the method described previously.22 Briefly, fresh samples were ground into homogenates in methanol and centrifuged at 5000g for 10 min. The supernatants were used to measure the phytohormones using the enzyme-linked immunosorbent assay (ELISA) following the instruction provided by the manufacturer (Solarbio, Beijing, China). All the tests were repeated three times. Six replicate samples were analysed for each data point. GA3, ABA, ZR and IAA with known concentrations were analysed as standards following the same procedure described in the assay kits.
Determination of Ce content in plants
Dry sample (shoots/roots) powders were digested with a mixture of HNO3 and H2O2 using a microwave digestion system (MARS Xpress, CEM, USA). The residue after digestion was diluted with deionized water to 15 mL. Total Ce concentrations were measured by ICP-MS (Thermo, USA). Indium was used as an internal standard. Ce standard solutions (Thermo, USA) in the range 0.1 to 100 ng mL−1 were used for calibration. Bush branches and leaves (GBW 07602) were also digested as standard reference samples; the mean recovery from which was 101%. The limit of detection was 0.002 ng mL−1.
CeO2 NP dissolution in root exudates
To determine whether root exudates under different N conditions affect the CeO2 NP dissolution and uptake in plants, we extracted the root exudates from the plants according to a previously described method with modifications.23 Briefly, plants growing under LN, MN or HN conditions for 10 days without the presence of CeO2 NPs were transferred to new beakers, each of which containing 100 mL sterilized deionized water, and were cultivated in the dark for another 24 h. The solutions in the beakers were collected and filtered through filter paper (11 μm). CeO2 NP suspensions of 100 and 500 mg L−1 were prepared from the filtered root exudate solutions and placed in the plant growth chamber for 3 weeks. Samples were centrifuged and the Ce concentrations were measured by ICP-MS following the aforementioned methods.
Data analysis
Data were expressed as mean ± standard deviation (SD) (n = 8). Statistical analysis was performed on IBM SPSS 19.0. One-way ANOVA was used to evaluate the significance between data (multiway comparison such that differences between all groups were all compared). P < 0.05 was considered significantly different.
Results and discussion
CeO2 NPs improve plant growth under N stress
The seedling length and fresh and dry biomass of plants after treatment with CeO2 NPs under different N conditions are shown in Fig. 1. When the CeO2 NPs were not present (control groups), N stress (LN and HN) affected the plant growth significantly. Compared with the MN conditions, LN stress resulted in longer roots and higher root biomass with a decreased shoot length and biomass (Fig. 1A–C). The increase of root biomass/length and inhibition of shoot growth of plants under LN stress are in accordance with previous studies.24 The enhanced root growth was an adaptive response to N deficiency in order to increase the root absorption of N, resulting in an anatomical change. It was reported that the density of the cells in the meristematic zones and the volume of the cells in the elongation zones both increased as responses to N deficiency.24 However, due to the limit of N nutrient, the shoot elongation and biomass production were inhibited (Fig. 1D–F). In contrast, when N was supplied excessively, both the root and shoot growth were inhibited, which also agree with previous reports.2 These results suggest a successful simulation of N stresses.
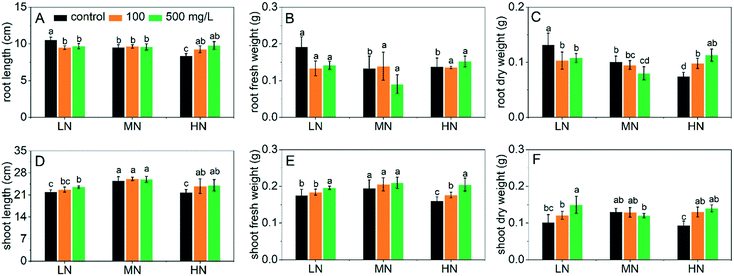 |
| Fig. 1 Seedling length and fresh and dry biomass of rice following CeO2 NP treatments under three N conditions. (A–C) show the root length and fresh and dry weight, respectively. (D–F) show the shoot length and fresh and dry weight, respectively. Different lowercase letters indicate a significant difference at P < 0.05. | |
Addition of CeO2 NPs significantly improved the growth of plants under LN and HN stress (Fig. 1). The root lengths under LN stress were reduced by 10% and 8% following 100 and 500 mg L−1 CeO2 NP treatments compared to the MN controls (Fig. 1A); the fresh and dry biomass of roots was reduced by 26–31% and 18–22%, respectively (Fig. 1B and C). The reduction of plant root growth indicates alleviation of the LN stress by the CeO2 NPs, i.e. the plants did not need more root volume to facilitate absorption of N. As a consequence, the biomass of shoots under LN stress was significantly increased by the addition of the CeO2 NPs (Fig. 1E and F). CeO2 NPs also improved the plant growth under HN stress by promoting the seedling elongation (Fig. 1A and D) as well as the biomass production (Fig. 1B and C; E and F). However, CeO2 NPs showed no significant positive effects on the plant growth under MN conditions and actually reduced the plant biomass at 500 mg L−1, which is in accordance with results reported in many previous studies performed under normal growth conditions where CeO2 NPs may exhibit toxic effects to plants at high concentrations.25,26 Overall, the data suggest that CeO2 NPs can improve the growth of rice under N stress, and the mechanisms involved were explored in the following experiments.
CeO2 NPs enhance N assimilation by regulating key enzymes involved in N assimilation and metabolism
In this study, the C content under different N conditions was not significantly different (Fig. 2A and B), while the N content in roots and shoots increased with the increase of N supply as expected (Fig. 2C and D). The N content in roots under HN was 1.55 and 0.8 fold higher than that under LN and MN, and it was 1.45 and 0.44 fold higher in shoots under HN than that under LN and MN. This resulted in a shift of the C/N balance. The C/N ratio in roots increased to 36.4 under LN and decreased to 14.2 under HN (Fig. 2E), and the C/N ratio in shoots increased from 13.7 to 23.2 under LN and decreased to 9.5 under HN (Fig. 2F), suggesting that CeO2 NP treatment had more significant effects on the C/N balance in plants under LN than under HN stress.
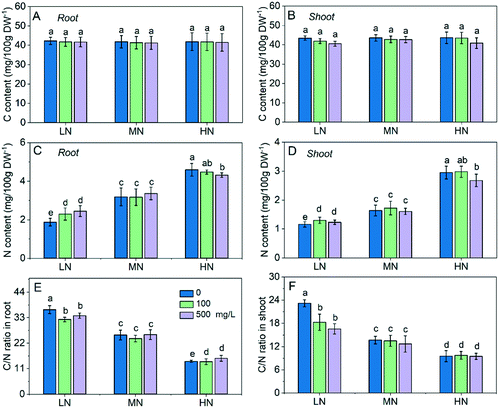 |
| Fig. 2 Carbon (C) content in roots (A) and shoots (B). N content in roots (C) and shoots (D). C/N ratio in roots (E) and shoots (F). Different lowercase letters indicate a significant difference at P < 0.05 – in all cases the MN conditions were the control conditions to which the LN and NH conditions are compared. | |
The C content in roots and shoots were not significantly affected by CeO2 NP treatment compared with that of their own control (Fig. 2A and B). The N content in roots and shoots under LN stress was enhanced by 6–12% and 22–30%, respectively, following CeO2 NP treatments (Fig. 2C and 2D). Under HN stress, the high dose (500 mg L−1) of CeO2 NP treatment reduced the N content in roots and shoots by 9% and 6%, respectively. As a consequence, the C/N ratios under LN stress was reduced by 11% and 7% in roots and 21% and 29% in shoots, respectively, following 100 and 500 mg L−1 CeO2 NP treatments (Fig. 2E and F). C and N are both essential elements for all living organisms. In plants, maintaining the C/N balance is important for the normal functioning of the plant. The C/N ratio has been used as an indicator of the growth, senescence and nutrient mobilization of plants.2 These results suggest that CeO2 NPs can improve the N absorption by plants to cope with N deficiency under LN stress while they reduce the N absorption by plants to avoid N overload and maintain the C/N balance in plants under the excessive N conditions.
We examined the activity of the three key enzymes involved in N assimilation. The activities of glutamine synthetase (GS), glutamine oxoglutarate aminotransferase (GOGAT) and glutamate dehydrogenase (GDH) in roots under LN stress were all enhanced by CeO2 NP treatments (Fig. 3B–D). The GS and GOGAT activities in shoots were not affected by CeO2 NPs (Fig. 3E and G); however, as an alternative enzyme for N assimilation, the GDH activity was enhanced by 44–80% (Fig. 3G). Under HN stress, CeO2 NPs did not significantly affect the enzyme activities in roots but downregulated the GS and GDH activity thereby reducing N assimilation in shoots (Fig. 3E and G). Under MN conditions, CeO2 NPs did not affect the activity of GS and GOGAT in roots but reduced the activity of GDH at 500 mg L−1 (Fig. 3D); similar phenomena were observed in shoots (Fig. 3E–G).
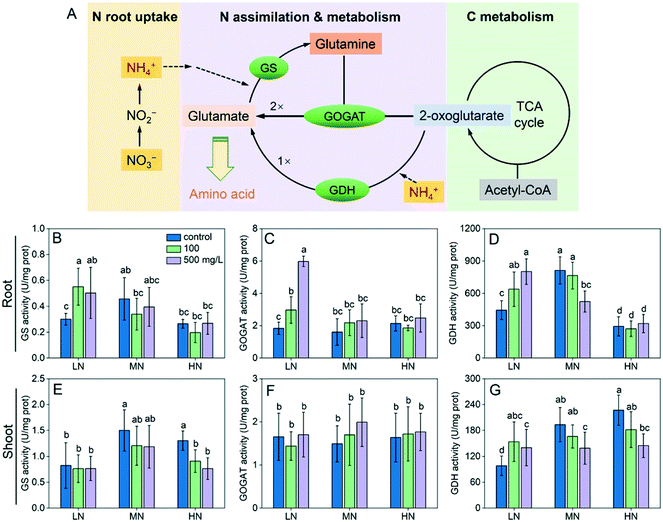 |
| Fig. 3 (A) Schematic showing the key N assimilation process in plants. GS, GOGAT and GDH activity in roots (B–D) and shoots (E–G) under different N conditions following CeO2 NP treatments. Different lowercase letters indicate a significant difference at P < 0.05. | |
Plants take up N mainly in the forms of NO3− and NH4+ which were supplied as KNO3, Ca(NO3)2 and (NH4)2SO4 in the nutrient solutions. The NO3− is transformed to NH4+ for plant assimilation, a process which transforms inorganic N into organic N compounds such as amino acids. The glutamine synthetase/glutamate synthase (GS/GOGAT) cycle is the primary pathway of N assimilation in plants (Fig. 3A).27 In the first step, GS catalyses the reaction of NH4+ with glutamate, producing glutamine. GOGAT subsequently catalyses the transfer of the amide group from glutamine to 2-oxoglutarate (produced during C metabolism), which produces two molecules of glutamate. As an alternative pathway of glutamate formation, glutamate dehydrogenase (GDH) catalyses the reaction of 2-oxoglutarate with NH4+, which forms one molecule of glutamate. The glutamate is then used by plants for synthesis of amino acids. The aforementioned results correlated well with the results of seedling length, biomass and N content. In summary, CeO2 NPs can enhance the N assimilation by regulating the key enzymes involved under N deficiency thereby enhancing the plant growth; when N is supplied excessively, CeO2 NPs can also regulate the enzymes to reduce the N assimilation. Analysis of the genes associated with the N assimilation enzymes at the transcriptomic level is necessary in future studies to further understand the impact of CeO2 NPs on the N metabolism.
CeO2 NPs alleviate oxidative stress and enhance plant tolerance
The impact of CeO2 NPs on antioxidant enzymes and oxidative stress in rice plants is shown in Fig. 4. Under LN stress, the SOD and POD activities in roots and shoots were not different compared with those under MN (Fig. 4A, B, E and F); however, the MDA content in roots and shoots was 66% and 32% higher under LN stress (Fig. 4C and G), suggesting oxidative damage of the cell membrane. LN also caused oxidative DNA damage to plants, as evidenced by the significantly increased levels (increased by 93% compared with that under MN) of 8-OHdG in shoots (Fig. 4H). HN stress did not induce significant MDA over accumulation in roots compared with the MN conditions (Fig. 4D); however, it caused 147% higher accumulation of MDA in shoots (Fig. 4H), suggesting oxidative damage in shoots.
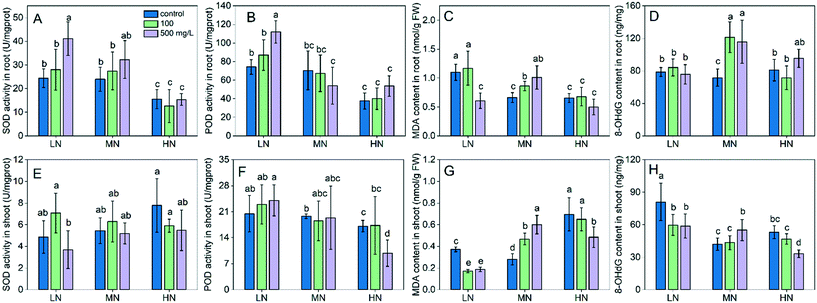 |
| Fig. 4 SOD activity (A and E), POD activity (B and F), and MDA (C and G) and proline (D and H) content in roots and shoots under different N conditions with CeO2 NP treatments. The upper and bottom rows represent the results in roots and shoots, respectively. Different lowercase letters indicate a significant difference at P < 0.05. | |
Under LN conditions, CeO2 NPs significantly increased the SOD activity and reduced the MDA content in roots at 500 mg L−1. CeO2 NPs at 100 and 500 mg L−1 also reduced the MDA accumulation in shoots by 54% and 49%, respectively (Fig. 4G). Under HN conditions, CeO2 NPs did not change the MDA levels in roots while they reduced the POD activity by 43% and MDA content by 25% in shoots at 500 mg L−1. However, under MN conditions, the high dose (500 mg L−1) of CeO2 NPs caused oxidative damage, as shown by the over accumulation of MDA in both roots and shoots (Fig. 4C and G). These data suggest that CeO2 NPs alleviate the oxidative damage caused by both N deficiency and N excess. The higher reduction of MDA levels by 500 mg L−1 CeO2 NP treatment under LN stress (49%) than under HN stress (25%) suggests that CeO2 NPs were more effective in alleviating stress caused by LN than by HN. Alleviation of oxidative damage by CeO2 NPs was further shown by the decreased level of 8-OHdG, a biomarker of oxidative DNA damage. CeO2 NPs at 100 and 500 mg L−1 reduced the 8-OHdG levels in shoots under LN stress by 54% and 50%, respectively (Fig. 4H). CeO2 NPs at 500 mg L−1 reduced the 8-OHdG level in shoots by 37% under HN stress (Fig. 4H).
The capacity of CeO2 NPs to reduce oxidative stress, as mentioned previously, may be related to their ROS scavenging activity due to the exchange of Ce3+/Ce4+ and the surface oxygen vacancies in the lattice. Due to this feature, the potential of CeO2 NPs as mimics of antioxidant enzymes such as SOD or CAT has been explored.28,29 The potential of CeO2 NPs to enhance plant tolerance has been also explored recently.30,31 It was reported that CeO2 NPs can scavenge hydroxyl radicals and thus enhance plant tolerance to salinity stress.30 The reduced oxidative damage in our study may also be related to the ROS scavenging activity of CeO2 NPs.
In addition to the antioxidative enzymes, we also explored whether the reduced oxidative stress in the presence of CeO2 NPs was related to proline accumulation. A key feature of proline is that it accumulates to high levels in plants as a response to stresses, which has been documented in numerous studies.32 Over accumulation of proline imparts plant stress tolerance by maintaining osmotic balance, preventing membrane leakage and maintaining redox potential.33 Proline buffers the redox potential in cells because its synthesis and oxidation involve the generation and cycling of NADP+ and NADPH; thus, it is vital for plant antioxidant defence.34 As shown in Fig. 5, LN did not affect the proline level in rice plants compared with the MN conditions, while the HN increased the proline levels in both roots and shoots. This suggests that proline is only involved in the plant tolerance to HN stress. CeO2 NP treatment did not change the proline levels under LN stress, suggesting that proline accumulation was not the pathway via which CeO2 NPs alleviated the plant stress arising from the lack of N. However, addition of CeO2 NPs increased the proline levels in both roots and shoots thereby enhancing the plant stress tolerance under HN stress, which agrees with the results that the oxidative damage (MDA) was reduced by the CeO2 NP treatments (Fig. 4C and 4G). Overall, these results suggest that CeO2 NPs can alleviate the oxidative stress in plants and enhance plant tolerance to stress by stimulating proline accumulation.
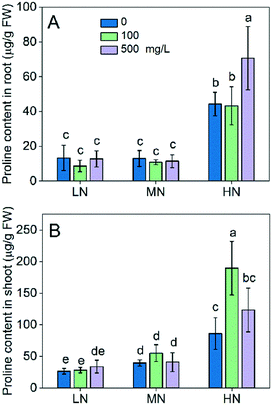 |
| Fig. 5 Proline content in roots (A) and shoots (B). Data were expressed as mean ± SD. Different lowercase letters indicate a significant difference at P < 0.05. | |
CeO2 NPs enhanced plant tolerance to stress by regulating phytohormones
Phytohormones are a group of signalling molecules playing pivotal roles in modulating plant growth under various environmental conditions, including abiotic stress.35 ABA, which is a well-known “stress phytohormone”, is the most studied hormone due to its essential role in plant adaption and tolerance to abiotic stress. Endogenous ABA levels in plants may increase rapidly in response to external stress such as drought, salinity, heat or environmental contaminants.36 Under water stress, ABA signals plant leaves to take actions such as stomatal closure, reducing leaf expansion to reduce transpiration and thus avoid water loss.37 It was also reported that ABA is involved in the modification of root architecture for adaption to drought38 or N deficiency. In our study, LN stress did not affect the ABA level in roots (Fig. 6A) but increased the ABA level in shoots by 42% (Fig. 6B) as compared with the MN conditions. HN stress caused a more dramatic response of ABA in the plants. The ABA levels in roots and shoots were 2.1 and 2.2 fold higher in HN than those under MN conditions (Fig. 6A and B). CeO2 NP treatments did not significantly affect the ABA levels in roots under all N conditions while they reduced the ABA levels in shoots under LN and HN stress, suggesting that the stress was reduced. However, under MN conditions, CeO2 NPs at 100 mg L−1 and 500 mg L−1 upregulated the ABA levels by 88% and 71%, respectively (Fig. 6B), suggesting that CeO2 NP treatment caused stress in plants growing under normal conditions.
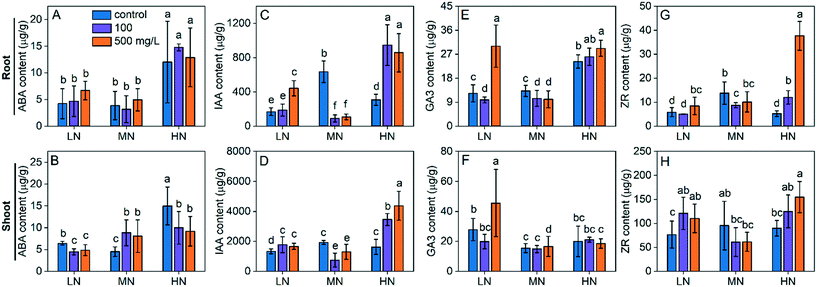 |
| Fig. 6 Content of phytohormones, including ABA (A and B), IAA (C and D), GA3 (E and F) and ZR (G and H), in plant roots (upper) and shoots (bottom). Different lowercase letters indicate a significant difference between groups at P < 0.05. | |
IAA, also known as auxin, which has been studied for a century, plays vital roles in the regulation of plant growth and development, and the adaptive modulation of plant growth under stress conditions, although the exact mechanism is not yet fully understood.39 It was reported that external stress such as salinity reduced the IAA level in plants40 and that exogenously applied IAA can promote plant growth.41 As shown in Fig. 6C and D, LN and HN stress reduced the IAA levels in both roots and shoots. CeO2 NP treatments increased the IAA levels in roots and shoots under LN and HN stress. Moreover, CeO2 NPs caused a higher increase under HN stress (177–205% in roots and 112–168% in shoots) than under LN stress (11–161% in roots and 25–32% in shoots). The results suggest that CeO2 NPs promote plant growth and tolerance by upregulating the IAA levels. However, the IAA levels were decreased by CeO2 NPs under MN, suggesting the inhibition of plant growth by CeO2 NP treatment when N is supplied normally.
GA is a growth hormone regulating various processes in plants such as germination, stem elongation, flowering and senescence. The role of GA as an indicator of abiotic stress is also increasingly evident.42 Reduction of ABA levels in plants represents a stress response that contributes to the restriction of plant growth. The LN stress did not induce significant alteration of the GA content in plants while HN stress caused 83% higher GA content in roots, as compared with the MN conditions (Fig. 6E and F), suggesting that the GA response is more sensitive to HN stress. CeO2 NP (500 mg L−1) treatment increased the GA levels in roots and shoots under LN stress by 150% and 70%, respectively, and increased the GA level by 20% in roots under HN stress. The GA response to CeO2 NP treatments under the MN conditions is not significant.
ZR is the most active form of naturally occurring cytokinins which regulate plant growth as well as plant response to abiotic stress.43 ZR is often considered as an antagonist of ABA; an increased ABA content is usually accompanied by a decreased ZR content. In our study, both LN and HN stress caused reduced ZR levels in plants (Fig. 6G and H), which corresponds with the increased ABA levels. CeO2 NP treatments increased the ZR levels under both LN and HN conditions. Overall, these results suggest that CeO2 NPs alleviate plant stress by regulating the phytohormone levels in plants.
Differential Ce uptake in plants affected by N supply conditions
Since the CeO2 NPs can alleviate plant stress via different routes including acting as a ROS scavenger, it is crucial to know the Ce content in plants. As shown in Fig. 7, the Ce accumulation in plants is dose dependent. Interestingly, the Ce content in roots and shoots depended on the N concentration following the order: LN > MN > HN. The Ce content in roots treated with 500 mg L−1 CeO2 NPs was 1.6- and 3.4-fold higher under LN conditions than those under MN and HN conditions, respectively (Fig. 7A). The Ce content in shoots treated with 500 mg L−1 CeO2 NPs was 1.3- and 1.4-fold higher under LN conditions than those under MN and HN conditions, respectively (Fig. 7B). We deduced that this phenomenon might be related to the different root activities under different N conditions. Under N deficiency, the plants increased the root biomass for absorption of N,24 which may also enhance the absorption of CeO2 NPs simultaneously. However, under HN conditions, the root growth was inhibited, and the absorption capacity was reduced correspondingly. Moreover, root exudates play an essential role in the uptake and transformation of CeO2 NPs.44 The composition of root exudates changes with the variation of the root environment.45 We extracted the root exudates and found that dissolution of CeO2 NPs in root exudates extracted under LN conditions was higher than that under the other two conditions (Fig. 7C). It has been reported that under N deficiency, the amount of metabolites such as glutarate, adipate, 2-hydroxyisobutyrate, succinate, 2-isopropylmalate, raffinose, abscisate, GABA, L-glutamine, L-glutamate, and calcium pantothenate in root exudates increased.46 These metabolites all have carboxylic groups that may chelate the Ce3+ thereby enhancing the dissolution of CeO2 NPs, which may account for the high Ce uptake under LN conditions. Enhanced dissolution of CeO2 NPs and uptake of Ce in plants in the presence of carboxyl compounds (e.g., ascorbic acid, citric acid) have been previously reported.46 Inorganic ligands such as phosphates, which can reprecipitate and immobilize the Ce3+, can restrict the uptake of Ce in plants.
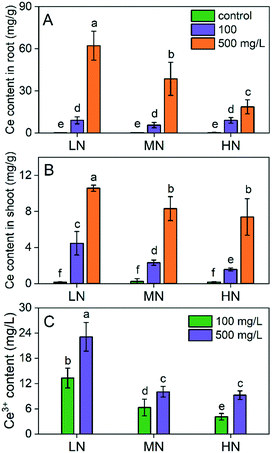 |
| Fig. 7 Ce content in plant roots (A) and shoots (B) and the dissolution of CeO2 NPs (C) in root exudates extracted under different N conditions. Different lowercase letters indicate significant difference at P < 0.05. | |
In summary, this study for the first time reported the alleviation of N stress (deficit or excess) and improvement of plant growth under N stress by CeO2 NP treatments. CeO2 NPs can enhance N assimilation and improve the N use efficiency in plants by regulating the key enzymes involved in N assimilation. Moreover, CeO2 NPs alleviate the oxidative damage caused by N stress by regulating the antioxidant enzymes, proline accumulation and phytohormone levels in the rice plants. All these effects contributed to the improved plant growth. Ce uptake in plants was affected by the N stress conditions; N deficiency resulted in increased dissolution of CeO2 NPs thereby increasing Ce accumulation in plants, which was mainly attributed to the altered root biomass and increased amount of carboxyl compounds in the root exudates. Notably, CeO2 NPs showed no positive effects on plant growth and inhibited plant growth at high concentrations in the absence of N stress, suggesting that caution should be still made to the unintentionally discharged CeO2 NPs in areas with normal growth conditions. It should be noted that this study was carried out in a hydroponic system. The impacts of CeO2 NPs on plants under different N conditions can be made complex by the soil conditions and different agricultural management (e.g., water regime). Future studies in soils are thus needed. Taken together, this study provides significant insights into the physiological effects of CeO2 NPs on plant growth and a potential way to improve N use efficiency by plants under N stress conditions, which in fact widely exist in realistic N fertilization activity.
Associated content
Characterization of CeO2 NPs including TEM, Raman spectroscopy, and zeta potential and hydrodynamic size measurements is provided in the ESI.†
Conflicts of interest
The authors declare no conflict of interest.
Acknowledgements
This work was supported by the National Key R&D Program of China (2017YFD0801103, 2017YFD0801300), the NSFC-Guangdong Joint Fund (U1401234), the National Natural Science Foundation of China (41371471, 41130526). Funding support from the Institute for Global Innovation theme Environmental Pollution Solutions (project no. 2076) of the University of Birmingham is acknowledged.
References
- T. Araya, K. Noguchi and I. Terashima, Effect of nitrogen nutrition on the carbohydrate repression of photosynthesis in leaves of Phaseolus vulgaris L, J. Plant Res., 2010, 123, 371–379 Search PubMed.
- L. Kong, Y. Xie, L. Hu, J. Si and Z. Wang, Excessive nitrogen application dampens antioxidant capacity and grain filling in wheat as revealed by metabolic and physiological analyses, Sci. Rep., 2017, 7, 43363 Search PubMed.
- L. Ding, K. Wang, G. Jiang, D. Biswas, H. Xu, L. Li and Y. Li, Effects of nitrogen deficiency on photosynthetic traits of maize hybrids released in different years, Ann. Bot., 2005, 96, 925–930 Search PubMed.
- Y.-L. Lin and Y.-F. Tsay, Influence of differing nitrate and nitrogen availability on flowering control in Arabidopsis, J. Exp. Bot., 2017, 68, 2603–2609 Search PubMed.
- N. Frias-Moreno, A. Nuñez-Barrios, R. Perez-Leal, A. C. Gonzalez-Franco, A. Hernandez-Rodriguez and L. Robles-Hernandez, Effect of nitrogen deficiency and toxicity in two varieties of tomatoes (Lycopersicum esculentum L.), Agric. Sci., 2014, 5, 1361 Search PubMed.
- M. Zhang, H. Wang, Y. Yi, J. Ding, M. Zhu, C. Li, W. Guo, C. Feng and X. Zhu, Effect of nitrogen levels and nitrogen ratios on lodging resistance and yield potential of winter wheat (Triticum aestivum L.), PLoS One, 2017, 12, e0187543 Search PubMed.
- L. Lassaletta, G. Billen, B. Grizzetti, J. Anglade and J. Garnier, 50 year trends in nitrogen use efficiency of world cropping systems: the relationship between yield and nitrogen input to cropland, Environ. Res. Lett., 2014, 9, 105011 Search PubMed.
- G. V. Lowry, A. Avellan and L. M. Gilbertson, Opportunities and challenges for nanotechnology in the agri-tech revolution, Nat. Nanotechnol., 2019, 14, 517–522 Search PubMed.
- M. Kah, N. Tufenkji and J. C. White, Nano-enabled strategies to enhance crop nutrition and protection, Nat. Nanotechnol., 2019, 14, 532–540 Search PubMed.
- N. Kottegoda, C. Sandaruwan, G. Priyadarshana, A. Siriwardhana, U. A. Rathnayake, D. M. Berugoda Arachchige, A. R. Kumarasinghe, D. Dahanayake, V. Karunaratne and G. A. Amaratunga, Urea-hydroxyapatite nanohybrids for slow release of nitrogen, ACS Nano, 2017, 11, 1214–1221 Search PubMed.
- M. N. Khan, M. Mobin, Z. K. Abbas, K. A. AlMutairi and Z. H. Siddiqui, Role of nanomaterials in plants under challenging environments, Plant Physiol. Biochem., 2017, 110, 194–209 Search PubMed.
- J. Faraji and A. Sepehri, Ameliorative effects of TiO2 nanoparticles and sodium nitroprusside on seed germination and seedling growth of wheat under PEG-stimulated drought stress, J. Seed Sci., 2019, 41, 309–317 Search PubMed.
- V. Kardavan Ghabel and R. Karamian, Effects of TiO2 nanoparticles and spermine on antioxidant responses of Glycyrrhiza glabra L. to cold stress, Acta Bot. Croat., 2020, 79 DOI:10.37427/botcro-2020-025.
- W. H. Elmer and J. C. White, The use of metallic oxide nanoparticles to enhance growth of tomatoes and eggplants in disease infested soil or soilless medium, Environ. Sci.: Nano, 2016, 3, 1072–1079 Search PubMed.
- H. Wu, N. Tito and J. P. Giraldo, Anionic cerium oxide nanoparticles protect plant photosynthesis from abiotic stress by scavenging reactive oxygen species, ACS Nano, 2017, 11, 11283–11297 Search PubMed.
- L. Rossi, W. Zhang and X. Ma, Cerium oxide nanoparticles alter the salt stress tolerance of Brassica napus L. by modifying the formation of root apoplastic barriers, Environ. Pollut., 2017, 229, 132–138 Search PubMed.
- P. Trogadas, J. Parrondo and V. Ramani, CeO2 surface oxygen vacancy concentration governs in situ free radical scavenging efficacy in polymer electrolytes, ACS Appl. Mater. Interfaces, 2012, 4, 5098–5102 Search PubMed.
- F. R. Cassee, E. C. van Balen, C. Singh, D. Green, H. Muijser, J. Weinstein and K. Dreher, Exposure, health and ecological effects review of engineered nanoscale cerium and cerium oxide associated with its use as a fuel additive, Crit. Rev. Toxicol., 2011, 41, 213–229 Search PubMed.
- P. Zhang, Z. Guo, Z. Zhang, H. Fu, J. C. White and I. Lynch, Nanomaterial Transformation in the Soil–Plant System: Implications for Food Safety and Application in Agriculture, Small, 2020, 2000705 Search PubMed.
- R. F. Chen, R. F. Shen, P. Gu, X. Y. Dong, C. W. Du and J. F. Ma, Response of rice (Oryza sativa) with root surface iron plaque under aluminium stress, Ann. Bot., 2006, 98, 389–395 Search PubMed.
- L. S. Bates, R. P. Waldren and I. Teare, Rapid determination of free proline for water-stress studies, Plant Soil, 1973, 39, 205–207 Search PubMed.
- H. Gawronska, A. Deji, H. Sakakibara and T. Sugiyama, Hormone-mediated nitrogen signaling in plants: implication of participation of abscissic acid in negative regulation of cytokinin-inducible expression of maize response regulator, Plant Physiol. Biochem., 2003, 41, 605–610 Search PubMed.
- K. Tawaraya, R. Horie, T. Shinano, T. Wagatsuma, K. Saito and A. Oikawa, Metabolite profiling of soybean root exudates under phosphorus deficiency, Soil Sci. Plant Nutr., 2014, 60, 679–694 Search PubMed.
- L. Qin, T. C. Walk, P. Han, L. Chen, S. Zhang, Y. Li, X. Hu, L. Xie, Y. Yang and J. Liu, Adaption of roots to nitrogen deficiency revealed by 3D quantification and proteomic analysis, Plant Physiol., 2019, 179, 329–347 Search PubMed.
- P. Zhang, Y. Ma, Z. Zhang, X. He, Y. Li, J. Zhang, L. Zheng and Y. Zhao, Species-specific toxicity of ceria nanoparticles to Lactuca plants, Nanotoxicology, 2015, 9, 1–8 Search PubMed.
- Y. Ma, C. Xie, X. He, B. Zhang, J. Yang, M. Sun, W. Luo, S. Feng, J. Zhang and G. Wang, Effects of Ceria Nanoparticles and CeCl3 on Plant Growth, Biological and Physiological Parameters, and Nutritional Value of Soil Grown Common Bean (Phaseolus vulgaris), Small, 2020, 1907435 Search PubMed.
- C. Masclaux-Daubresse, M. Reisdorf-Cren, K. Pageau, M. Lelandais, O. Grandjean, J. Kronenberger, M.-H. Valadier, M. Feraud, T. Jouglet and A. Suzuki, Glutamine synthetase-glutamate synthase pathway and glutamate dehydrogenase play distinct roles in the sink-source nitrogen cycle in tobacco, Plant Physiol., 2006, 140, 444–456 Search PubMed.
- Y. Yang, Z. Mao, W. Huang, L. Liu, J. Li, J. Li and Q. Wu, Redox enzyme-mimicking activities of CeO 2 nanostructures: Intrinsic influence of exposed facets, Sci. Rep., 2016, 6, 35344 Search PubMed.
- Y. Li, X. He, J. J. Yin, Y. Ma, P. Zhang, J. Li, Y. Ding, J. Zhang, Y. Zhao and Z. Chai, Acquired superoxide-scavenging ability of ceria nanoparticles, Angew. Chem., Int. Ed., 2015, 54, 1832–1835 Search PubMed.
- H. Wu, L. Shabala, S. Shabala and J. P. Giraldo, Hydroxyl radical scavenging by cerium oxide nanoparticles improves Arabidopsis salinity tolerance by enhancing leaf mesophyll potassium retention, Environ. Sci.: Nano, 2018, 5, 1567–1583 Search PubMed.
- J. P. Giraldo, M. P. Landry, S. M. Faltermeier, T. P. McNicholas, N. M. Iverson, A. A. Boghossian, N. F. Reuel, A. J. Hilmer, F. Sen and J. A. Brew, Plant nanobionics approach to augment photosynthesis and biochemical sensing, Nat. Mater., 2014, 13, 400–408 Search PubMed.
-
P. E. Verslues and S. Sharma, Proline metabolism and its implications for plant-environment interaction, The Arabidopsis Book, 2010, vol. 8, p. e0140 Search PubMed.
- S. Hayat, Q. Hayat, M. N. Alyemeni, A. S. Wani, J. Pichtel and A. Ahmad, Role of proline under changing environments: a review, Plant Signaling Behav., 2012, 7, 1456–1466 Search PubMed.
-
M. A. Hossain, M. A. Hoque, D. J. Burritt and M. Fujita, in Oxidative damage to plants, Elsevier, 2014, pp. 477–522 Search PubMed.
- S. H. Wani, V. Kumar, V. Shriram and S. K. Sah, Phytohormones and their metabolic engineering for abiotic stress tolerance in crop plants, Crop J., 2016, 4, 162–176 Search PubMed.
- K. Vishwakarma, N. Upadhyay, N. Kumar, G. Yadav, J. Singh, R. K. Mishra, V. Kumar, R. Verma, R. Upadhyay and M. Pandey, Abscisic acid signaling and abiotic stress tolerance in plants: a review on current knowledge and future prospects, Front. Plant Sci., 2017, 8, 161 Search PubMed.
-
H. Pirasteh-Anosheh, A. Saed-Moucheshi, H. Pakniyat and M. Pessarakli, Stomatal responses to drought stress, Water Stress and Crop Plants, John Wiley & Sons, Ltd, (Chichester), UK, 2016, pp. 24–40 Search PubMed.
- M. A. Rosales, C. Maurel and P. Nacry, Abscisic acid coordinates dose-dependent developmental and hydraulic responses of roots to water deficit, Plant Physiol., 2019, 180, 2198–2211 Search PubMed.
- A. Kohli, N. Sreenivasulu, P. Lakshmanan and P. P. Kumar, The phytohormone crosstalk paradigm takes center stage in understanding how plants respond to abiotic stresses, Plant Cell Rep., 2013, 32, 945–957 Search PubMed.
- H. Du, H. Liu and L. Xiong, Endogenous auxin and jasmonic acid levels are differentially modulated by abiotic stresses in rice, Front. Plant Sci., 2013, 4, 397 Search PubMed.
- M. G. Javid, A. Sorooshzadeh, S. A. M. M. Sanavy, I. Allahdadi and F. Moradi, Effects of the exogenous application of auxin and cytokinin on carbohydrate accumulation in grains of rice under salt stress, Plant Growth Regul., 2011, 65, 305–313 Search PubMed.
- E. H. Colebrook, S. G. Thomas, A. L. Phillips and P. Hedden, The role of gibberellin signalling in plant responses to abiotic stress, J. Exp. Biol., 2014, 217, 67–75 Search PubMed.
- V. Verma, P. Ravindran and P. P. Kumar, Plant hormone-mediated regulation of stress responses, BMC Plant Biol., 2016, 16, 86 Search PubMed.
- P. Zhang, Y. Ma, Z. Zhang, X. He, J. Zhang, Z. Guo, R. Tai, Y. Zhao and Z. Chai, Biotransformation of ceria nanoparticles in cucumber plants, ACS Nano, 2012, 6, 9943–9950 Search PubMed.
- P. Zhang, Y. Ma, C. Xie, Z. Guo, X. He, E. Valsami-Jones, I. Lynch, W. Luo, L. Zheng and Z. Zhang, Plant species-dependent transformation and translocation of ceria nanoparticles, Environ. Sci.: Nano, 2019, 6, 60–67 Search PubMed.
- K. Tawaraya, R. Horie, T. Wagatsuma, K. Saito and A. Oikawa, Metabolite profiling of shoot extract, root extract, and root exudate of rice under nitrogen and phosphorus deficiency, Soil Sci. Plant Nutr., 2018, 64, 312–322 Search PubMed.
Footnotes |
† Electronic supplementary information (ESI) available. See DOI: 10.1039/d0en00757a |
‡ The two authors contributed equally. |
|
This journal is © The Royal Society of Chemistry 2020 |